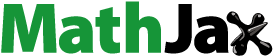
ABSTRACT
Effective wastewater treatment is essential due to the dye environmental threats. Photocatalysis offers an eco-friendly dye degradation solution. Herein, we report a double Z-scheme photocatalyst, i.e. LaFeO3/Boron-doped g-C3N4/Fe2O3 (LFO/B-CN/FO), fabricated via a hydrothermal method. The formation of this ternary system is supported by structural, surface, and morphological analyses. Differential scanning calorimetry and thermal gravimetric analysis demonstrated the crystallization behavior of the composite. Because of synergetic effects in the ternary composite and boron doping, the optical band gap energy is reduced to 1.6 eV. Under visible light irradiation, the LFO/B-CN/FO nanocomposite degraded navy-blue dye by 98% in 130 min at neutral pH, surpassing CN, B-CN, and binary composites of B-CN with LFO and FO. The degradation process was rationalized using pseudo-first- and pseudo-second-order kinetic models, which confirmed high catalytic efficiency. The double Z-scheme heterojunction enhanced light absorption, charge separation, and carrier lifetime, hence improving the photocatalytic activity. Overall, the as-fabricated LFO/B-CN/FO ternary composite outperformed reference catalysts in terms of chemical reactivity, optical characteristics, and electrical performance. This work opens up a new route for the design and fabrication of double Z-scheme ternary composite photocatalysts for potential wastewater treatment and renewable energy applications.
Introduction
Earth is currently facing major environmental problems as a result of the direct release of industrial toxins into the ecosystem (Citation1). Rapid industrial growth and increasing anthropogenic pollution are major problems that are endangering the ecology, especially water bodies, and putting humans and other living things at risk (Citation2). Water contains a range of contaminants, including heavy metal ions, pharmaceuticals, and chemical dyes. Over time, the need of clean water has been rising while its availability is on the decline. Therefore, in order to meet the demand for water, it is necessary to purify wastewater and make it suitable for consumption by humans and other organisms (Citation3). Given the increasing environmental pollution caused by organic dyes, it is imperative to create photocatalytic technology that can efficiently transform hazardous dyes into water, carbon dioxide, and less toxic chemical compounds. Thus, in order to fulfill these requirements, it is necessary to develop a highly efficient visible light active photocatalyst that produces electron–hole (e−−h+) pairs for redox reactions. Furthermore, it has been noted that the utilization of conventional photocatalysts like CeO2, TiO2, and ZnO, among others, exhibits limited efficiency in harnessing visible light and experiences rapid recombination of photoinduced electron–hole pairs, hence reducing their practical applicability. Hence, our future strategy should prioritize the development of a novel photocatalyst that exhibits enhanced optical activity when exposed to visible light (Citation4).
Researchers investigated that the design and fabrication of 2D g-C3N4-based heterostructure photocatalysts have the potential to act as highly efficient visible light active catalysts. They possess significant potential and provide benefits such as a large specific surface area, small charge diffusion distance, and excellent redox capability. However, rapid recombination of charges hindered the photocatalytic performance of individual 2D g-C3N4 nanosheets. Various methods, such as elemental doping, co-catalyst introduction, and heterojunction construction, have been extensively investigated to address this limitation. The synthesis of Z-scheme heterojunctions, composed of two or more semiconductors having a staggered band structure, has shown significant and promising steps forward. While compared to conventional type-II heterojunction photocatalysts such as g-C3N4/ZnO, TiO2/g-C3N4, and g-C3N4/Ag2CrO4 etc., Z-scheme systems including WO3/g-C3N4, SnS2/g-C3N4, etc. demonstrate exceptional photocatalytic redox features upon stimulation by light, resulting in improved charge separation (Citation5).
In order to improve the ability of Z-scheme system to absorb more light, efficiently separate charge carriers, and retain strong redox features, a new photocatalytic system based on g-C3N4, combined with metal oxides or nonmetal oxides, must be designed. The purpose of this system is to effectively utilize solar energy and improve the redox capabilities in Z-scheme heterojunctions based on g-C3N4 (Citation6). A ternary Z-scheme photocatalyst, made up of BiFeO3-g-C3N4-WO3, was prepared using a wet chemical technique and subsequently utilized for water splitting to evolve H2 and 2,4-dichlorophenol degradation, achieving rates of 90 μmol·h–1·g–1 and 63%, respectively. The improved performance, in comparison to pure g-C3N4, was attributed to the interfacial contacts between g-C3N4 and coupled BiFeO3 and WO3 constituents (Citation7). Likewise, carbamazepine (CBZ) was degraded by Co3O4/CuBi2O4/SmVO4, a dual Z-scheme heterojunction photocatalyst, under visible light, by 76.1% ± 3.81. The Z-scheme heterojunction's ability to promote effective electron–hole separation, better interfacial contact, and visible light absorption is responsible for this improvement (Citation8). Reduced graphene oxide (rGO) was utilized as an electron mediator in the synthesis of the Z-scheme ternary photocatalyst ZnMoO4/BiFeWO6/rGO. Anthraquinonic acid blue 25 (AB 25) was degraded by ZM/BFW/rGO with remarkable photocatalytic performance; the degradation percentage was higher than that of the ZM (24%) and BFW (36%), when compared across 180 min. In addition, to confirm its promise for scalable wastewater treatment applications, the photocatalyst showed excellent stability with 89% efficiency after four cycles (Citation9).
Likewise, a double Z-scheme heterojunction i.e. LaFeO3/g-C3N4/BiFeO3 was fabricated via a wet chemical technique and used for the degradation of ciprofloxacin. It was observed that the ternary nanocomposite exhibits superior performance compared to the individual counterparts i.e. pure g-C3N4, LaFeO3, BiFeO3 (Citation10). Furthermore, a ternary composite i.e. g-C3N4/Ag3PO4/AgI was fabricated and utilized in the photocatalytic degradation of nitenpyram. The resultant photocatalyst revealed exceptional performance compared to the individual coupling counterparts. A double z-scheme WO3/Bi2O3/g-C3N4 photocatalyst was synthesized and utilized in efficient visible light catalytic degradation of tetracycline pollutants. Similarly, α-Fe2O3/g-C3N4/TiO2 ternary nanocomposite was fabricated via the two steps hydrothermal and calcination methods and utilized for the removal of Rhodamine B dye. The as-fabricated photocatalyst revealed 95.7% dye removal performance in only 50 min which was attributed to the synergetic interaction among the TiO2, g-C3N4, and α-Fe2O3 counterparts (Citation11).
The LaFeO3 perovskite semiconductor has outstanding performance in advanced oxidation processes, especially in the elimination of organic pollutants, degradation of harmful gases, and producing hydrogen fuel through water splitting. Its small band gap of 2.1 eV, good stability, low cost, and nontoxic nature make it a desirable catalyst. Nevertheless, there are certain challenges to overcome, such as low surface area, high charge carrier’s recombination, and limited visible light response, which ultimately boosts the photocatalytic efficiency. Fabricating Z-scheme heterojunction between g-C3N4 and LaFeO3 can remarkably improve the charge carrier’s separation, as a result, the photocatalytic performance of g-C3N4 can be enhanced. Similarly, the coupling of hematite (α-Fe2O3), a semiconductor photocatalyst with a band gap of 2.2 eV, can also improve the performance of g-C3N4 (Citation12).
Thus, as expected, coupling of LaFeO3 and Fe2O3 with boron-doped g-C3N4 can result in a ternary double Z-scheme heterojunction which can significantly promote the photocatalytic performance of g-C3N4. Since g-C3N4 has a highly negative conduction band potential (−1.05 V vs NHE) which can strongly reduce the adsorbed oxygen to •O2− (−0.33 V vs NHE). Furthermore, valence band potentials of LaFeO3 (+2.21 V versus NHE) and Fe2O3 (+2.2 V vs NHE) are highly positive which can oxidize H2O/OH− to produce •OH radicals (+1.99 V vs NHE) (Citation13). Herein, the as-fabricated LFO/B-CN/FO double Z-scheme heterojunction is utilized in photocatalysis for navy-blue dye degradation. The catalyst revealed exceptional performance due to the improved light absorption and charge carrier’s separation, as well as enhanced stability. This work will open up new routes for the design and fabrication of highly efficient Z-scheme photocatalysts for energy production and environmental remediation.
Materials and methods
Chemicals
Analytical grade chemicals were purchased from Sinopharm Chemical Reagent Co., Ltd. and used as received. These include boric acid (H3BO3), melamine, citric acid monohydrate (C6H8O7H2O), iron(III) nitrate nonahydrate (Fe(NO3)3·9H2O), lanthanum(III) nitrate hexahydrate (La(NO3)3·6H2O), HCl (35%), NaOH, ethanol, methanol, ethylene glycol, CH3COONa, polyethylene glycol-600 (PEG-600), ethanol, benzoquinone (BQ), ethylenediaminetetraacetic acid (EDTA), and isopropyl alcohol (IPA) and navy-blue dye. Scheme 1 displays the structural formula of navy blue (C32H21N5Na2O6S2, molecular weight: 681.65).
Synthesis of boron-doped g-C3N4 nanocomposites (B-CN)
Boric acid (0.25 g) was dissolved in 50 mL of deionized (D.I) water, which resulted in a colorless transparent solution. Subsequently, 5 g of melamine was introduced into the aqueous solution, followed by stirring at 500 rpm for 15–20 min. The mixture was then transferred to a Teflon-lined autoclave and maintained at 200°C for 12 h. Afterward, the resultant mixture was recovered from the autoclave and subjected to heating for 4 h at 550°C with a heating rate of 10°C per min. The preparation of pure g-C3N4 (CN) followed the same procedure as used for B-CN, but without the addition of boric acid (Citation14).
Synthesis of LaFeO3 (LFO)
The LFO nanoparticles were prepared via a facile gel-combustion process and then calcined at a temperature of 200°C for 2 hours. In a typical experiment, 5 mL of D.I water was taken in a 25 mL beaker and then 2.1014 g of C6H8O7·H2O, 2.0201 g of Fe(NO3)3·9H2O, and 2.1650 g of La(NO3)3·6H2O were added to it. To make a homogenous solution, the mixture was magnetically agitated for 20 min at 500 rpm. To create a gel, the resulting solution was heated at 80°C for 12 h in an oven. It was then placed in a 30 mL corundum crucible and calcined in a muffle furnace at 200°C for 2 h with a temperature rise of 5°C min−1. Ethanol and DI water were used to wash the resulting powders after they naturally cooled to 25°C. The resulting material was then vacuum-dried for 4 hours at 100°C (Citation15).
Synthesis of Fe2O3 (FO)
Typically, a solvothermal technique was used to synthesize FO nanoparticles. Initially, 80 mL of ethylene glycol was used to dissolve 8.08 g of Fe(NO3)3·9H2O, which was then magnetically agitated at 500 rpm for 30 min at room temperature (25°C). About 2.0 g of polyethylene glycol-600 (PEG-600) and 7.20 g of CH3COONa were then added to the mixture, and agitated at 500 rpm for 1.5 h. The resultant solution was then transferred to 100 mL volume autoclaves lined with Teflon and heated at 200°C for 22 h in an oven to undergo solvothermal treatment. When the autoclave cooled to ambient temperature (25°C), the precipitate was washed with pure water and ethanol several times and then dried in an oven at 60°C for 12 h. Finally, the precipitate was calcined in a muffle furnace at 470°C for 2 h in an air environment (Citation16).
Synthesis of binary and ternary composites
A wet chemical method synthesis was used to synthesize the LaFeO3–B-g-C3N4 (LFO/B-CN) composites, with B-g-C3N4 being chosen as the base material. In 100 mL of a water and ethanol (1:1) mixture solvent, a predetermined quantity (5 g) of previously synthesized B-CN was dissolved. The solution was then stirred (at 500 rpm) for 5 h while 5% by weight LFO was added. Last but not least, the solution was calcined in the air for 2 h at 500°C (temperature ramp = 5°C min-1) after being dried for 12 h at 65°C. Fe2O3-B-g-C3N4 (FO/B-CN) and LaFeO3–B-g-C3N4–Fe2O3 (LFO/B-CN/FO) composites were prepared using a similar process. 5% by weight of FO was utilized in place of LFO for the preparation of FO/B-CN composite, while 5% by weight of both LFO and FO were added to the B-CN dispersion in the case of LFO/B-CN/FO composites preparation (Citation7). A pictorial representation of the procedure can be seen from Scheme 2.
Characterization
The synthesized materials were examined for various characteristics, including phase changes, surface charge, electronic transitions, elemental composition, thermal stability, chemical structure, etc. UV–Visible spectrometer (UV1800ENG240 V, SOFT, Shimadzu Corporation, Japan, model UV-1800, 220–240V-50–60 Hz 140VA) was used to study the optical properties of the materials. Fourier-transform infrared spectrometry (FT-IR, with attenuated total reflection, ATR, accessory model IRSpirit, produced by Shimadzu, 220–240 V) was used for surface chemical analysis. Scanning electron microscopy (SEM) and energy dispersive X-ray analysis (EDX) (FEGSEM/EDS model number: 354900-7673-000-08 en, Germany, 30 kv), analysis was done to confirm the morphology and composition of samples. The transmission electron microscopy (TEM) technique was used for capturing the TEM images. X-ray diffractometer (XRD, Model no: AXRD-LPD System USA, 220 V, 20A) was used for measuring the XRD patterns of the samples. Furthermore, the photocatalyst's point of zero charge (pHpzc) was assessed using the pH-drift method (pH meter, Model: HI 5521, powered by a 12 Vdc power supply). Similarly, UV–Visible spectrometer, in the wavelength range of 200–800 nm, was also used to assess the photocatalytic activities of these materials toward degradation of navy-blue dye.
Photocatalytic activity evaluation
The photocatalytic degradation tests of NB dye were conducted under visible light irradiation in order to assess the photocatalytic activity of the as-synthesized photocatalysts, as shown in Figure S1. The materials were also tested in the absence of light/catalyst. The least amount of NB dye was determined to have been degraded by using CN, B-CN, LFO/B-CN, FO/B-CN, and LFO/B-CN/FO photocatalysts in the dark. Thus, the degradation of NB was photocatalytic (light dependent) and was negligible in the dark. A 250 mL glass reactor was used for the NB dye degradation tests. To determine the adsorption equilibrium for each degradation test, 0.1 g of the catalyst was added to 100 mL of NB solution (50 mg/L) and stirred at 500 rpm in the dark for 30 min. The solution was then exposed to visible light for 130 min by using a 300 W Xenon lamp. After the photocatalytic reaction, the concentration of NB was measured using a UV–Visible spectrophotometer (UV1800ENG240 V, SOFT). The % removal was measured by using Equation (1) (Citation1). Similarly, the pseudo-first-order and pseudo-second-order kinetics models were calculated using Equations (2–5) (Citation17).
(1)
(1)
(2)
(2)
(3)
(3)
(4)
(4)
(5)
(5) where C0 and Ct are the NB dye concentration (mg/L), before and after different time intervals in the presence of light, respectively. Where, k (min−1) and k (M−1min−1) represents the degradation rate constant for the pseudo-first-order- and pseudo-second-order kinetics models respectively.
Effect of concentration on the λmax of navy-blue dye
Double beam UV–visible spectrophotometer was used to measure the concentration of NB dye at its λmax (i.e. 613 nm). At this wavelength, the broad absorption band is correlated to the chromophore of the azo group which is responsible for the appearance of blue color (Citation18). The λmax is not greatly changed with concentration; however, absorbance is continuously decreased. For this purpose, different concentration dye solutions were prepared. The absorbance versus wavelength plots, at various concentrations, are shown in Figure S1(a). Likewise, in this study, a calibration curve has been drawn for NB dye at the wave length (λmax) of 613 nm for several solutions of different concentrations i.e. 5, 10, 20, 30, 40 and 50, 60, 70, 80, 90, and 100 mg/L. The calibration (absorbance vs concentration) curve obtained for NB is shown in Figure S1(b). The concentration range used was 5–100 mg/L with a regression factor (R2) = 0.998, slope = 2.312, and the intercept = 0.019.
Results and discussion
X-ray diffraction
The X-ray diffraction (XRD) patterns of CN, B-CN, FO/B-CN, LFO/B-CN, and LFO/B-CN/FO were measured as revealed in (a). For CN, the in-plane structure of the trigonal nitrogen of tri-s-triazine layer units is represented by the less prominent peak at 2θ = 12.9° (100 plane). On the other hand, the more noticeable peak at 2θ = 27.4° (002) is linked to the conjugated aromatic segments interplane stacking, with an interlayer spacing of roughly 0.32 nm (the π–π stacking of heptazine rings (JCPDS Card No. 87-1526)). In B-CN, except for the peaks at 12.9° and 27.4°, the 2θ peaks at 20.4°, 41.7° and 50.23° are correlated to the crystallographic planes (103), (224), and (323), indicating the presence of Boron (JCPDS Card #: 01-070-0154) (Citation19). In the case of FO/B-CN, the distinct diffraction peaks at 33.2°, 35.6°, 40.9°, 49.5°, 54.1°, 57.6°, 62.4°, and 64.0° corresponds to the crystal planes (104), (110), (113), (024), (116), (018), (214), and (300) of hexagonal α-Fe2O3 (JCPDS 33-0664) (Citation20). Similarly, in LFO/B-CN sample, the peaks at 2θ values of 22.9°, 32.35°, 39.86°, 46.37°, 56.46°, 67.69°, and 76.98° correspond to the (101), (121), (220), (202), (240), (242), and (204) crystallographic planes of LaFeO3 (JCPDS card no. 88-0641). Similarly, the corresponding peaks of CN, B-CN, LaFeO3 and Fe2O3 can be seen in the XRD patterns of the LFO/B-CN/FO ternary composite (Citation10).
UV–visible spectroscopy analysis
The powder samples were dispersed in a 1:1 ratio water/absolute ethanol mixture to conduct the UV–Visible absorption measurements and the corresponding spectra are revealed in (b). As obvious, after boron doping, the intrinsic band gap of CN is slightly reduced and the B-CN revealed more absorption compared to the bare CN. Notably, after coupling LFO and FO, the optical absorption of binary (i.e. FO/B-CN and LFO/B-CN) and ternary (i.e. LFO/B-CN/FO) composites is significantly enhanced compared to the B-CN catalyst. This is due to the small band gaps of LFO and FO compared to the B-CN photocatalyst (Citation14). The band gap energy of the samples was calculated via the Tauc plot equation (Equation 6) using the UV–visible absorption data.
(6)
(6) In this equation, “Eg” represents the band gap energy, “A” is the proportionality constant, “υ” indicates photon frequency, “h” is the Planck constant, “α” is the absorption coefficient, and “γ” is the electronic transition, which might have the values of 2, 1/2, 2/3, or 1/3, depending on the kind of transition. (c) shows the Tauc plots of CN, B-CN, FO/B-CN, LFO/B-CN, and LFO/B-CN/FO catalysts. When (αhυ)2 was plotted against (hυ), an almost linear trend was seen, suggesting that direct permitted transitions are responsible for the absorption edge. The optical band gap (Eg) is represented by the value of the X-axis intersected by a straight line drawn. Clear and estimated optical energy band gap values of 2.7 and 2.5 eV were predicted for the CN and B-CN catalysts, respectively. These values are consistent with earlier research findings published in the literature (Citation21). A red shift in the absorption edges was seen when FO/LFO was added to B-CN, suggesting that heterojunction has been formed between the counter parts. It appears that adding LFO and FO to B-CN increased its capacity to absorb visible light, which led to a slight red shift. This observation implies that the optical properties of the base material can be adjusted by coupling proper band gap semiconductor nanomaterials. The band gaps of CN, B-CN, FO/B-CN, LFO/B-CN, and LFO/B-CN/FO nanocomposites were determined to be 2.7, 2.5, 2.2, 1.8, and 1.6 eV, respectively, based on the Tauc's equation curves (Citation22).
FT-IR spectroscopy analysis
(d) reveals the FT-IR spectra of CN, B-CN, FO/B-CN, LFO/B-CN, and LFO/B-CN/FO catalysts measured in the wavenumber range of 500–4000 cm−1. The FT-IR spectrum helps to assess the composition/surface functional groups of the catalysts. Notably, the peaks centered between 1200 and 1650 cm−1 are responsible for the CN heterocycle stretching modes. The broad band observed in the range of 2800−3400 cm−1 corresponds to the aromatic ring of the uncondensed terminal NH2 group (Citation23). Additionally, the peak at 2172 cm−1 correlates to the asymmetric stretching vibration mode of cyano group (CN or NC). The binary and ternary composites exhibit all the major vibration peaks of CN, demonstrating that the structure of CN does not change during the coupling process. In the case of B-CN catalyst, the observed distinctive absorption peak at about 810 cm−1 is associated with the bonds out-of-plane stretching vibrations (Citation24). The distinctive absorption peak at 573 cm−1 in the LFO/B-CN, FO/B-CN, and LFO/B-CN/FO composites could be attributed to the stretching vibration modes of Fe–O bonds. Peaks at 651, 756, and 873 cm-1 are due to the presence of iron content confirming the successful fabrication of nanocomposites (Citation25). Similarly, the peak at 530.2 cm−1, corresponding to the lattice oxygen of La–O, showed the presence of LaFeO3 in LFO/B-CN and LFO/B-CN/FO (Citation10).
Scanning electron microscopy analysis
SEM analysis was done to investigate the morphology of the as-prepared samples as can be seen in (a–e). Notably, the B-CN sample ((b)) exhibits a smoother, more porous surface, and a more streamlined shape than the pristine CN ((a)), which has a typical two-dimensional laminar structure (Citation26). The SEM image of FO/B-CN ((c)) demonstrates the aggregation of B-CN nanosheets along with ultra-small α-Fe2O3 nanoparticles anchored on its surface (Citation27). Additionally, in LFO/B-CN, the LFO nanoparticles were dispersed across the B-CN nanosheets as revealed in (d) (Citation28). The SEM image of LFO/B-CN/FO ternary composite ((e)) clearly demonstrates the presence of FO and LFO nanoparticles on the surface of B-CN catalyst. The potential photocatalytic reactions are possible due to their larger, rough and round surfaces (Citation28). (f) represents the SEM image of LFO/B-CN/FO ternary composite after usage of it, which reflects that no obvious changes were observed in its surface after its usage.
Transmission electron microscopy analysis
TEM was used to investigate the structural morphologies of the CN, B-CN, FO/B-CN, LFO/B-CN, and LFO/B-CN/FO photocatalysts as revealed in (a–e). The 2D morphology and lamellar structure of CN and B-CN are confirmed as shown in (a,b) (Citation21). As obvious, the CN surface becomes rough after the introduction of boron. After coupling FO nanoparticles to the lamellar structure of B-CN, a heterojunction was formed as revealed in (c) (Citation14). Likewise, (d) reveals the dispersion of LFO nanoparticles over the surface of B-CN in the binary LFO/B-CN composite. Notably, the TEM image of LFO/B-CN/FO reveals the presence of both LFO and FO nanoparticles over the surface of B-CN as displayed in (e). This confirms the successful fabrication of ternary heterojunction (Citation29).
Point of zero charge (pHpzc) of photocatalyst
One of the most important interfacial parameters is the pHpzc, or pH at the point of zero charges. The quality and quantity of surface charge of photocatalyst is directly affected by changing the pH of solution, which then directly or indirectly affects the photocatalytic activity of the catalyst. In general, a number of factors, such as the photocatalyst type, structure, synthesis method, and nature of the medium have a substantial impact on the pHpzc. The pH-drift method was used to estimate the pHpzc values of CN, B-CN, FO/B-CN, LFO/B-CN, and LFO/B-CN/FO photocatalysts. The pHpzc of the photocatalysts are the positions at which the initial and final pH of the aqueous solution containing the material/catalyst after 24 h are equivalent. By doing this, the point of zero charge pH values for CN, B-CN, FO/B-CN, LFO/B-CN, and LFO/B-CN/FO were determined to be 6.4, 6, 6.51, 6.5, and 6.69, respectively, as shown in and Figure S2. The surface is positively charged when the pH of the solution is lower than the pHpzc of the photocatalyst. On the other hand, the surface is negatively charged when the pH value is higher than the pHpzc of the photocatalyst. Under neutral pH conditions, it is found that the surface charge of all photocatalysts is supposed to be negative as this pH is higher than the pHpzc, while in acidic conditions, the surface charge is expected to be positive. Similar results were also repotted for pHpzc of BSO-BCN/Y (10) (Citation30).
Energy dispersive X-ray spectroscopy analysis
Figure S3(a–e) displays the EDS spectrum as well as the matching elemental composition peaks of CN, B-CN, FO/B-CN, LFO/B-CN, and LFO/B-CN/FO catalysts. As shown in Figure S3(a), the prominent peak in the CN EDS spectrum indicates the existence of C and N elements. Likewise, EDS spectrum peaks (Figure S3(b)) demonstrate the existence of C, N, and B. Using EDS spectra, the chemical makeup of the coupled FO with B-CN was assessed (Figure S3(c)). Similarly, Figure S3(d) displays the EDS spectrum of LFO/B-CN, whereas Figure S3(e) displays the EDS spectrum of LFO/B-CN/FO photocatalyst. The inset table shows that the ternary photocatalyst contains C, N, B, La, Fe, and O elements. Overall, these findings demonstrate the successful fabrication of the photocatalysts with high purity.
Thermal gravimetric analysis
TGA/DSC analyses for CN, B-CN, FO/B-CN, LFO/B-CN, and LFO/B-CN/FO photocatalysts were carried out under an N2 atmosphere in the range of 25−1000°C at 10°C/min to evaluate the thermal stability of the photocatalysts. The vaporization of the adsorbed H2O or other volatile contaminants on the sample surface is the main cause of the initial mass loss, which occurs below 200°C, as illustrated in (a,b) (Citation31). Further heating to 530.27°C results in a 91.77% weight loss, which is explained by the chemical decomposition of CN. In the case of B-CN, the decomposition temperature was approximately 683.68°C, and the weight loss was 95.37% at 541.05°C. The weight loss for FO/B-CN was 91.73% at 531.15°C, indicating that the addition of FO enhanced the decomposition temperature and raised the peak temperature to 707.34°C. As obvious, the weight loss for LFO/B-CN photocatalyst was 87.25% at 520.88°C and the peak temperature was 565.61°C. Notably, in the case of LFO/B-CN/FO ternary nanocomposite, the decomposition temperature was raised. As a result, the percent weight loss was 43.82% at 642.89°C. This clearly indicates that LFO/B-CN/FO ternary composite is more stable at high temperatures. These findings reveal that the significantly enhanced thermal stability of B-CN is due to the strong interaction between LFO, B-CN and FO components (Citation32).
Photocatalytic activities of the materials
Effect of catalysts dose
The as-fabricated photocatalysts were used to assess their photocatalytic activity for the degradation of NB dye. A 300-Watt Xenon lamp was used as a source of visible light irradiation. Furthermore, the degradation of NB dye was studied in the presence of photocatalysts under dark conditions, and in the presence of photocatalysts and light. As obvious, under dark conditions, very little amount of NB is removed by the LFO/B-CN/FO ternary composite. The as-synthesized photocatalysts revealed variable levels of photocatalytic activity, which is correlated to the generation, migration, and dissociation of photogenerated electron–hole pairs (). (a) showed that after 130 min of visible light irradiation, CN, B-CN, FO/B-CN, LFO/B-CN, and LFO/B-CN/FO photocatalysts degraded ∼21.37%, 34.37%, 66.05%, 73.56%, and 91.13% of NB dye, respectively, under identical conditions (i.e. photocatalyst dosage of 0.1 g, 100 mL NB dye solution with 50 mg/L concentration, pH of 7, temperature of 25°C). These results reveal the potential significance of the as-synthesized photocatalysts in environmental remediation. The percentage degradation of NB dye removal was calculated using Equation (1) (Citation33).
Figure 6. (a) Effect of different composites of CN, (b) effect of different NB dye concentrations, (c) effect of pH, and (d) effect of temperature.
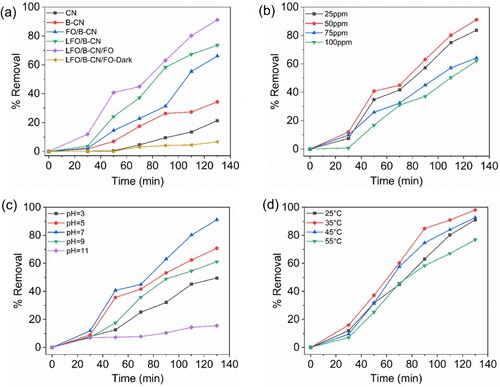
The LFO/B-CN/FO ternary composite exhibits superior NB dye degradation performance compared to the CN, B-CN, FO/B-CN, and LFO/B-CN photocatalysts. The significant NB dye degradation performance of the LFO/B-CN/FO photocatalyst is attributed to the improved charge carrier’s separation and transfer and extended light absorption, as these are considered important factors during pollutant degradation reactions. Notably, this structural characteristic also strengthens the conductive structure and increases the photo-absorption of LFO/B-CN/FO ternary nanocomposite, which further encourages the separation of photo-excited () pairs (Citation34).
In addition, the photocatalytic degradation of NB dye was evaluated using different kinetics models such as first-order, second-order, third-order, zero-order, pseudo-first-order (PFO), and pseudo-second-order (PSO). For the degradation of NB dye, the PFO and PSO kinetics models revealed the highest regression factor (R2) values among others. Equations (2) and (5) were utilized to ascertain the kinetics of NB dye removal of PFO and PSO, respectively (Citation33). As shown in (a), the R2 values of PFO kinetics model for CN, B-CN, FO/B-CN, LFO/B-CN, and LFO/B-CN/FO were found to be 0.809, 0.935, 0.838, 0.938, and 0.869, respectively, at identical conditions (i.e. 0.1 g catalyst, 50 mg/L NB dye, 25°C temperature, and pH 7). These findings led to the conclusion that the photocatalytic degradation of NB dye follows the PFO kinetics model. (a) illustrates the linear relationship between the inverse of and reaction time, which indicates the linear relationship for the removal of NB dye over the CN, B-CN, FO/B-CN, LFO/B-CN, and LFO/B-CN/FO photocatalysts. The plot of
versus time “t” was used to calculate the corresponding rate constant “k” from the slope for PFO kinetics as shown in Equation (2). In addition, Equation (7) was used to calculate the half-life (t1/2).
(7)
(7) Table S1 enlists the kinetic rate constants (k), half-lives (t1/2), and regression coefficients (R2) for the removal of NB dye using CN, B-CN, FO/B-CN, LFO/B-CN, and LFO/B-CN/FO photocatalysts. These kinetic results showed similarity with previously reported literature (Citation10). For CN, B-CN, FO/B-CN, LFO/B-CN, and LFO/B-CN/FO photocatalysts, the corresponding PFO rate constant (k) values were 0.0018, 0.0035, 0.0083, 0.0112, and 0.0179 per min. Notably, compared to other photocatalysts, LFO/B-CN/FO ternary composite has the highest rate constant value. Likewise, for CN, B-CN, FO/B-CN, LFO/B-CN, and LFO/B-CN/FO photocatalysts, the half-life (t1/2, min) values were found to be 378.68, 196.87, 82.79, 61.43, and 38.71 min, respectively. Again, it is obvious that the LFO/B-CN/FO photocatalyst has the shortest half-life (t1/2). These results reveal that the LFO/B-CN/FO ternary nanocomposite exhibits the highest performance for NB dye degradation compared to the other photocatalysts under identical conditions.
Figure 7. PFO kinetics for (a) the effect of different photocatalysts, (b) the effect of different concentrations of NB dye, (c) the effect of pH, and (d) the effect of temperature.
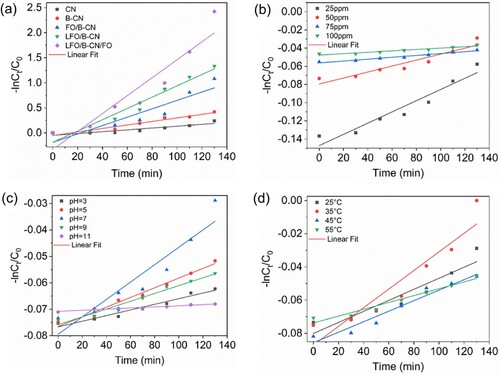
The degradation of NB dye over the CN, B-CN, FO/B-CN, LFO/B-CN, and LFO/B-CN/FO photocatalysts followed the PSO kinetics model because they had regression factor values of 0.828, 0.940, 0.916, 0.955, and 0.977, respectively (see Table S2). When 0.1 g of each photocatalyst was added while maintaining other parameters constant, a linear relationship was found between the reaction time and the inverse of as shown in (a). This indicates that the degradation process is adhered to the PSO kinetics model. The rate constant (k), regression factor (R2), and half-life (t1/2, min) values for CN, B-CN, FO/B-CN, LFO/B-CN, and LFO/B-CN/FO photocatalysts are shown in Table S2. The trend followed by these photocatalysts is similar to the previously published materials (Citation35). After stirring in the dark for 30 min, the final concentration of NB dye was monitored at specific time intervals (i.e. 20 min) under visible light irradiation, to calculate the percentage degradation of NB dye using Equation (1). Additionally, Equations (5) and (8) were used to determine the kinetics of the NB dye degradation and their half-life (t1/2 min). In these formulas, k stands for the rate constant (min−1) for the PSO kinetics model, and C0 is the initial concentration of NB dye, which was taken as 50 mg/L (Citation36).
(8)
(8) In addition to the PFO kinetics, as shown in Table S1, it was found that the degradation of NB dye by these photocatalysts followed PSO kinetics as shown in Table S2. It is found that the PSO rate constant (k) values for CN, B-CN, FO/B-CN, LFO/B-CN, and LFO/B-CN/FO photocatalyst are predicted to be 0.0016, 0.0029, 0.0053, 0.0064, and 0.0073 (M−¹ min−¹), respectively. Further, LFO/B-CN/FO photocatalyst has the highest rate constant (k) value compared to the others as revealed in Table S2. Furthermore, the half-life (t1/2, min) values for CN, B-CN, FO/B-CN, LFO/B-CN, and LFO/B-CN/FO photocatalysts are estimated to be 417.46, 238.14, 129.05, 107.94, and 94.93, respectively as shown in Table S2. This indicates that LFO/B-CN/FO has the shortest half-life (t1/2 min).
Effect of initial concentration of navy-blue dye
We also investigated the effect of the initial concentration of NB dye on the photocatalytic activity of the photocatalysts. Target pollutants were selected while keeping all other parameters constant for different concentration of NB dye i.e. 25 to 100 mg/L in order to evaluate their impact on the photocatalytic performance of LFO/B-CN/FO photocatalyst. The catalyst dose, temperature and pH were maintained at 0.1 g, 25°C, and 7, respectively, under visible light irradiation and from the reaction mixture, 5 mL was taken at regular time intervals up to 130 min. The % degradation of NB dye after 130 min irradiation was measured to be 83.67, 91.13, 64.11, and 62.07% for 25, 50, 75, and 100 mg/L, respectively, as shown in (b). The results concluded that at 50 mg/L NB solution, the maximum degradation happened and after that concentration decrease was noted in degradation as shown in (b) and Tables S1 and S2. The interaction between NB dye molecules and the LFO/B-CN/FO photocatalyst surface-active sites is responsible for this behavior. The probability of NB dye molecules making contact with the active sites on the LFO/B-CN/FO photocatalyst surface increases with the increase in NB dye concentration (Citation37). However, concentrations over this threshold might prevent NB dye molecules from efficiently making contact with the LFO/B-CN/FO photocatalyst (Citation38). The interference may cause photocatalysis to proceed more slowly, which would reduce degradation efficiency. Moreover, the adsorption and degradation of NB dye molecules was facilitated by the catalyst surface, such as that of the LFO/B-CN/FO photocatalyst. At lower concentrations of NB dye (i.e. 50 mg/L), the probability of contact between NB molecules and the catalyst surface is maximum as can be seen in (b). However, at concentrations above this threshold, the dye molecules start to take up a lot of the surface area that is accessible, thereby reducing the degradation performance of the photocatalyst (Citation39).
After analyzing the plots, it was evident that the PFO ((b)) and PSO ((b)) reveal the highest regression factor (R2) values for NB dye, compared to the other kinetics models at identical factors. The regression factor values of PFO for 25, 50, 75, and 100 mg/L were found to be 0.972, 0.977, 0.988, and 0.955, respectively. It is clear from Table S1 data that 75 mg/L solution has the highest PFO reaction kinetics regression factor value. Similarly, the maximum rate constant value (k1 = 1.027 E−4 min−1) for PFO was observed at this concentration. This value is substantially higher than the k1 value for other concentrations, which are 6.170 E−4, 3.286 E−4, and 7.673 E−5 for 25, 50, and 100 mg/L, respectively, as illustrated in Table S1. Additionally, Table S1 displays the half-life (t1/2, min) values for PFO at different concentrations of NB dye. The half-life values were predicted to be 9031.0, 6743.9, 2108.8, and 1123.1 min for 100, 75, 50, and 25 mg/L, further demonstrating that in comparison to the other concentrations, the PFO half-life was lower for the 25 mg/L NB dye solution.
Similarly, the degradation data related to the influence of the initial concentration of NB dye were analyzed using the PSO kinetics model as shown in (b). Table S2 reveals the regression factor values i.e. 0.972, 0.977, 0.988, and 0.955 for 25, 50, 75, and 100 mg/L, respectively. The highest regression factor value was observed for the 75 mg/L NB dye solution. Notably, Table S2 displays the PSO rate constant (k2) values. As obvious, the highest PSO rate constant value was 0.0073 M–1 min−¹ at 50 mg/L. This value is much greater than other values, like 0.0068 at 25, 0.0051 at 75, and 0.0051 M−¹min−1 at 100 mg/L. In addition, the half-lives (t1/2, min) were determined for each dosage, and the results showed that their values at 25, 50, 75, and 100 mg/L are 100.58, 94.93, 134.0, and 135.35 min, respectively. This suggests that compared to other NB dye concentrations, the half-life at 50 mg/L is lower. The higher rate constant values indicate that the PFO and PSO appear to be favored over any potential competing reaction order, specifically at 50 mg/L concentration of NB dye solution (Citation24). As a result, in relation to the NB dye concentration, the degradation process over the LFO/B-CN/FO photocatalyst tends to follow the PFO and PSO. This suggests that the concentration of NB dye slightly influences the degradation kinetics and the compatibility of PFO and PSO behavior due to the concentration of NB dye and is in agreement with the previous reports (Citation40).
Effect of initial pH
The wastewater pH values fluctuate according to the dye content. Thus, we need to investigate how the pH of the medium influence the degradation of NB dye. In order to do so, the pH was adjusted while maintaining other parameters constant as shown in Table S1. This was done to evaluate the photocatalytic degradation performance of LFO/B-CN/FO photocatalyst toward NB dye. The degradation performance at pH 3, 5, 7, 9, and 11 were calculated to be 49.57, 70.75, 91.13, 61.19, and 15.54%, respectively, after irradiation for 130 min, as revealed in (c). The maximum degradation performance of 91.13% was observed at pH 7. It is imperative to maintain a neutral pH during photocatalysis in order to keep the outstanding degradation performance of the photocatalyst. At extreme pH values, the catalyst surface may lose its active sites which may result in the formation of complexes (Citation41). Conversely, when the pH drops below 7 and increases beyond 7, positive and negative charges can be visible on the catalyst surface. Under both basic and acidic conditions, the interaction of with positive holes produces OH*. In a basic environment,
oxidizes, resulting in the surface of LFO/B-CN/FO containing OH* (Citation42). On the other hand, the coulombic attraction between the positive active sites of the photocatalyst and
may limit the production of OH* in an acidic environment, thereby decreasing the effectiveness of the process. When it comes to anionic azo dyes, which are challenging to remove from negatively charged surfaces, the benefits of maintaining high pH levels are evident. Conversely, at lower pH values, electrons in the conduction band of the catalyst eliminate NB dye through the reductive breakage of azo bonds. Additionally, in acidic conditions, the surface area accessible for
absorption and dye degradation is decreased due to the heterojunction’s features of LFO/B-CN/FO photocatalyst. Consequently, the pH emerges as a critical factor influencing the characteristics of wastewater. It influences direct hole oxidation, direct electron reduction, and the activity of OH* in the conduction band. Protonation and deprotonation-induced pH variations also modify the dyes specifications, which have a fundamental impact on the dye’s redox activity and degradation features. The observed fluctuations in the photocatalytic degradation behavior of NB dye could perhaps be attributed to the substantial coulombic attraction on the LFO/B-CN/FO surface, particularly at the neutral pH (Citation43).
The PFO and PSO kinetics models, which are depicted in and , respectively, were found to be followed during the photocatalytic degradation process after employing several kinetics models. The rate constant (k), regression factor (R2), and half-life (t1/2) for PFO and PSO are shown in Tables S1 and S2, respectively. The observed rate constants (k1) for PFO were determined to be 1.064 E−4, 1.830 E−4, 3.286 E−4, 1.476 E−4, and 2.123 E−5 min−1 for pH values of 3, 5, 7, 9, and 11, respectively. At pH 7, a higher rate constant value was observed. Similar to this, the Regression factor (R2) values for PFO at pH 3, 5, 7, 9 and 11 were 0.978, 0.967, 0.981, 0.968, and 0.936, respectively, demonstrating quite excellent Regression coefficients. For pH values of 3, 5, 7, 9, and 11, the calculated half-life values were 6509.3, 3786.8, 2108.8, 4693.5, and 32636.3 min, respectively. In comparison to the other pH values determined and shown in Table S1, it was found that at pH 7, the rate constant value is higher and the half-life value is lesser; suggesting that neutral pH conditions are more fruitful in this case (Citation44).
As can be seen in (c), the degradation of NB dye over the LFO/B-CN/FO ternary nanocomposite also follows the PSO kinetics model. Table S2 contains the relevant data for the various parameters. At pH 3, 5, 7, 9, and 11, the rate constant values for PSO were determined to be 0.0040, 0.0057, 0.0073, 0.0052, and 0.0011 M−1 min−1, respectively. In a similar way, pH 7 has the highest rate constant. Additionally, the values of the regression coefficient (R2) were found to be 0.973, 0.961, 0.977, 0.962, and 0.923, at pH 3, 5, 7, 9, and 11, respectively. The rate constant and regression coefficients were found to be greater at pH 7. The half-life for PSO was also calculated as shown in Table S2. At pH 3, 5, 7, 9, and 11, the values were 169.4, 121.5, 94.93, 132.7, and 624.3 min, respectively. Again, a small value of the half-life was obtained at pH 7, compared to other pH values. Hence, it is confirmed that pH 7 is an appropriate value for photocatalytic degradation of NB dye over the LFO/B-CN/FO photocatalyst (Citation36).
Effect of temperature
The removal of NB dye over time by LFO/B-CN/FO photocatalyst at various temperatures i.e. 25, 35, 45, and 55°C was also studied and the results are depicted in (d). At the above-mentioned temperatures, the percentage decomposition of NB dye was 91.13, 98.10, 81.63, and 76.87%, respectively. It is clear that when the temperature rises from 25 to 35°C, the percentage degradation of NB dye increases. Nevertheless, the rate of NB dye removal decreased when the temperature increased to 55°C. This may be due to the fact that the active sites on the catalyst surface deactivate at high temperatures (Citation37).
After putting temperature-dependent data through a number of kinetics models, it was determined that the data followed PFO as well as PSO kinetics models as presented in Tables S1 and S2 and and , respectively. (d) displays the kinetic studies of NB dye degradation over LFO/B-CN/FO at various temperatures for PFO. The regression factor value for PFO was maximum at 25°C, reaching 0.987, as shown in Table S1. The PFO regression factor values at 35, 45, and 55°C were 0.961, 0.923, and 0.968, respectively. Additionally, it was found that the rate constant (k) values for PFO at 25, 35, 45, and 55°C were 3.325 E−4, 5.603 E−4, 3.165 E−4, and 2.059 E−4 min−1, respectively. According to these findings, the rate constant value peaked around 35°C. Similarly, the half-lives (t1/2) for PFO were 2083.9, 1236.6, 2188.9, and 3364.7 min at 25, 35, 45, and 55, respectively. In accordance with the previous findings, it was revealed that the half-life was highest at 55°C and lowest at 35°C (Citation45).
Figure 9. Plots of lnK versus the inverse of temperature (in Kelvin) for the thermodynamic parameter of the photocatalytic removal of NB dye.
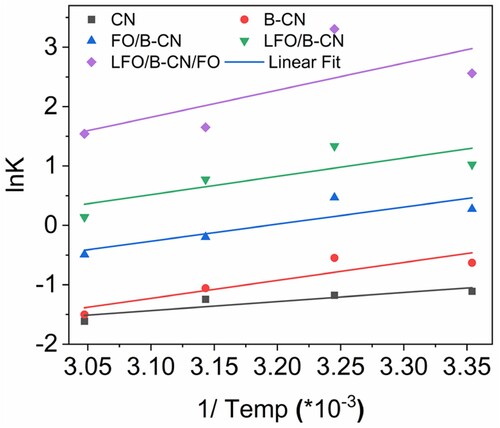
Likewise, the degradation of NB dye over LFO/B-CN/FO photocatalyst also followed the PSO kinetics model, as presented in Table S2 and (d). The regression coefficient values for the PSO kinetic model were determined to be 0.987, 0.961, 0.923, and 0.968 at temperatures of 25, 35, 45, and 55°C, respectively. The rate constants (k2) for 25, 35, 45, and 55°C were found to be 0.0074, 0.0083, 0.0071, and 0.0064 M−1 min−1, respectively. The PSO kinetics at these temperatures were found to have half-live periods of 93.14, 83.29, 96.51, and 107.10 min, respectively. At 35°C, the shortest half-life of 83.29 min was noted which implied that the half-life period increased with temperature after 25°C. These results are consistent with the findings reported in earlier studies (Citation36).
Degradation thermodynamics studies
In order to investigate the thermodynamic basis of the photocatalytic degradation of NB dye, Equations (9–11) were used to compute the changes in enthalpy (ΔH°), entropy (ΔS°), and Gibbs free energy (ΔG°). In order to estimate the free energy changes, it is necessary to calculate the apparent equilibrium constant () for the temperature range using the following equation.
(9)
(9) The photocatalytic degradation performance of NB dye is represented by
. It is the ratio of NB dye concentration (degraded) on the surface of the photocatalyst to the NB dye concentration un-degraded at an equilibrium state in the bulk of the solution. The ΔG° values were determined by applying Equation (10) to study the thermodynamics process. This method determines the photocatalytic degradation performance of ternary composite for NB dye and improves the understanding of the required energy dynamics, providing a stronger basis for optimizing photocatalytic degradation mechanisms (Citation30). In order to determine the changes in Gibbs free energy (ΔG°) and to get information regarding the photocatalytic degradation thermodynamics, Equation (10) was applied.
(10)
(10)
The photocatalyst degradation performance of the catalyst for NB dye is shown by the degradation equilibrium constant (), the R (universal gas constant) has a value of 8.314 J mol–1K–1. For further understanding of thermodynamics parameters, the following equation could be used.
(11)
(11) In Equation (11), T, ΔS°, and ΔH° present temperature (Kelvin), entropy change, and enthalpy change, respectively. The graph was obtained by plotting
versus 1/T as shown in . The intercept and slope were calculated from the values of ΔS° and ΔH°. Similarly, the ΔG° values were calculated as presented in Table S4. The ΔG° values were positive for CN, B-CN, and LFO/B-CN at all studied temperatures, which reveals that the degradation of NB dye over these photocatalysts is nonspontaneous. There is one exceptional case for LFO/B-CN photocatalyst because the ΔG° value is negative at 35°C which means that at this temperature, the degradation is spontaneous. Similarly, the ΔG° values are negative for FO/B-CN and LFO/B-CN/FO catalysts at all the applied temperatures. These negative values reveal that the degradation process of NB is spontaneous at all temperatures. These results provide a dynamic report for photocatalytic degradation of NB dye, which provides the significance of enthalpy and entropic contributions in various catalytic approaches, in thermodynamics complex frameworks. This complex thermodynamic study enhances our understanding of the photocatalytic process and capability, which makes a durable support to the area of environmentally remediation’s technology. The variations in Gibbs free energy (ΔG°) of NB dye degradation reveal that it is a spontaneous and feasible thermodynamics process. The ΔG° positive values for CN, B-CN, and LFO/B-CN photocatalysts reveal that the degradation pathway is nonspontaneous and needs external energy for the degradation of NB dye. The main cause is the barrier in high activation energy that restricts the active sites for surface adsorption. Conversely, the negative ΔG° values linked to FO/B-CN and LFO/B-CN/FO catalysts reveal the presence of spontaneous degradation mechanisms. Such a process indicates a favorable set of conditions for the reaction to occur, most likely as a result of improved charge carrier separation, improved adsorptive interactions, or increased availability of active sites properties that are specific to the binary (LFO/B-CN) and ternary (LFO/B-CN/FO) composites. These results emphasize the energy dynamics of NB dye degradation and show that how the composition of the photocatalyst affects the degradation pathways for thermodynamic viability.
Recyclability of photocatalyst
The success of photocatalytic processes is largely dependent on the life time and effectiveness of a photocatalyst. Using the same photocatalyst for NB dye degradation over several cycles, the studies were performed for recycling in order to evaluate the reusability of the LFO/B-CN/FO ternary nanocomposite. Eight consecutive cycles made up the recycling process, as shown in (a,b). Centrifugation was done to recover the photocatalyst, which was then thoroughly cleaned with deionized water and absolute ethanol before being heated for a final time for 12 h at 65°C and then calcination was done at 650°C in order to remove the NB dye molecules from the catalyst materials for further use. After such treatments, the catalyst material was re-used for the degradation of NB dye. A slow but visible decline in photocatalytic activity was seen during the recycling tests. For each of the eight cycling runs, the degrading performance was found to be 98.10, 94.59, 90.80, 87.09, 85.92, 84.40, 82.21, and 79.96%, for first, second, third, fourth, fifth, sixth, seventh and eighth cycles, respectively. These outcomes highlight the catalyst's stability, reusability, and total recoverability from the reaction system. It was noted that the degradation performance slightly decreased with the re-use of these materials, which could have been caused by catalyst poisoning or mass loss during the NB dye degradation process. Notably, as shown in (c,d), the reusability patterns observed for each run were consistent with the PFO and PSO kinetic models, similar to previously published reports (Citation46).
Comparative study
Based on the literature cited in this work and elsewhere, it is observed that the catalytic and photocatalytic abilities of the materials are affected by several factors, including the chemical and physical nature and structure of the catalyst, and the arrangement of constituent moieties in the composite, the amount of catalyst, target pollutant and other experimental conditions. In this regard, Table S4 is added to highlight a comparison of the removal performance of the LFO/B-CN/FO photocatalyst with recently reported photocatalysts. Brief outlines for this comparative study are summarized here.
Polyaniline photocatalyst was used for the degradation of methyl orange and methylene blue dyes which showed 42 and 97% removal, respectively, in less than 3 h. The first-order kinetics was followed by the degradation process of both the dyes (Citation47). Similarly, using a glucose-assisted hydrothermal process, LaFeO3/g-C3N4/ZnO Z-scheme photocatalyst was synthesized. The 10%–LaFeO3/g-C3N4/ZnO composite degraded 97.43% of 20 mg/L phenol in 150 min. Even after 5 catalytic cycles, the catalyst maintained 92.13% degradation performance, confirming its high stability (Citation48). The g-C3N4/SnFe2O4/GO photocatalyst was used to decompose methylene blue dye that revealed 98% degradation in 1 h. This ternary nanocomposite was found reusable for five times (Citation49). Similarly, a ternary composite of g-C3N4-WO3/rGO was used for methylene blue dye degradation. The composite showed 76% dye degradation within 180 min. The degradation followed the PFO kinetics model (Citation50). Likewise, a novel g-C3N4/ZnO/α-Fe2O3 ternary Z-scheme heterojunction photocatalyst showed 99.34% degradation performance for Tartrazine dye in 95 min. The degradation followed the PFO kinetics model and reusable for three times (Citation29). Beyond these, another ternary composite of AgCl/Au/CN was used for rhodamine B dye degradation which showed 93.1% degradation within 85 min. The composite was reusable for four times (Citation51). The ternary metal/g-C3N4/ZnO nanocomposite was used for EBT and MO dyes degradation which showed 23 and 24% degradation in 120 and 130 min, respectively (Citation52). The Ag/FeWO4/g-C3N4 composite was used for Rhodamine B dye degradation which showed 98% removal in 150 min (Citation53). A ternary composite i.e. Ag/ZnO/S-g-C3N4 was used for MB dye degradation which showed 98% degradation within 90 min (Citation40). The Trichosporon beigelii NCIM-3326 was used for NB dye degradation through microbial treatment. Under these conditions, 82% degradation was achieved within 48 h (Citation54). Similarly, a novel g-C3N4/rGO/Bi2Fe4O9 Z-scheme nanocomposite was used for Congo red dye degradation which showed 86.7% removal within 60 min (Citation55). In the same manner, CoAl2O4 nanoparticles were used for NB dye degradation which showed 67% degradation in 180 min (Citation56). LaNiO3/g-C3N4/MoS2 Z-scheme photocatalysts demonstrated pollutant removal capability with 96.6% Cr(VI) reduction and 91.6% TC degradation (Citation57). Similarly, the Ag/g-C3N4/LaFeO3 ternary nanocomposite showed efficiency of 98.97% (MB in 90 min) and 94.93% (TC in 120 min) (Citation58). The g-C3N4 nanosheets/layered MoS2/grapheme composite, which was synthesized by in situ adsorption, demonstrated better photocatalytic activity because of improved charge carrier separation via the interconnected surfaces and fast charge transfer pathways (Citation59). The NiF nanoparticles were synthesized by thermally treating water to improve the adsorption of cationic crystal violet dye. Adsorption kinetics were evaluated using intra-particle diffusion, Elovich, pseudo-first-order, and pseudo-second-order models (Citation60). The formation of ZnO-activated carbon nanocomposites was achieved through the co-precipitation. Congo red dye (CR) and malachite green (MG) were both readily adsorbed and photocatalyzed by the nanocomposites. The capacity of ZnO-AC was found to be greater than their other samples, while following the PSO adsorption kinetics mechanism. In the presence of hydroxyl radicals, photodegradation followed PFO (Citation61).
It is clear from the table that in the current study, a new LaFeO3/B-g-C3N4/Fe2O3 ternary composite which follows a double Z-scheme charge transfer mechanism was synthesized and used in the degradation of NB dye. The synthesized composite revealed 98% degradation within 130 mins under neutral pH and 35°C. This double Z-scheme demonstrates superiority over g-C3N4, B-g-C3N4, and their binary composites with LaFeO3 and Fe2O3 metal oxides. In addition, as-fabricated ternary composite shows better dye degradation performance compared to most of the previously reported catalysts as mentioned in Table S4. The overall performance of the ternary photocatalyst could be attributed to the plentiful number of active sites, improved light absorption, and promoted charge carrier’s separation.
Photocatalytic process mechanism
In order to verify the active species involved in the dye degradation process, scavenger trapping experiments were carried out for NB dye degradation over the LFO/B-CN/FO ternary composite photocatalyst. The results are displayed in Figure S5(a, b). During the photocatalysis process, various radical scavengers, including benzoquinone (BQ), ethylenediaminetetraacetic acid disodium (EDTA-2Na), and isopropyl alcohol (IPA), were employed to identify the production of superoxide radicals, holes, and hydroxyl radicals, respectively. During each experiment, identical conditions i.e. photocatalyst dosage of 0.1 g, 100 mL NB dye solution with 50 mg/L concentration, pH of 7, the temperature of 35°C, and 5 drops of 1 mM related scavenger were employed. The scavenger trapping experiments over the LFO/B-CN/FO ternary photocatalyst revealed that the primary active species for the dye degradation process are the hydroxyl and superoxide radicals. The addition of EDTA-2Na resulted in a recorded degradation efficiency of approximately 91.2%, indicating that holes are not involved in the degradation process. The addition of BQ and IPA decreased the NB dye degradation performance to 54.48 and 44.81%, respectively. The scavengers trapping experiment suggests that both hydroxyl and superoxide radicals contribute to the dye degradation process (Citation62).
To assess the photocatalytic performance of a catalyst, it is necessary to investigate its charge transfer mechanism. As shown in Scheme 3, under visible light irradiation, electron–hole pairs are produced in the LaFeO3/B-g-C3N4/Fe2O3 (LFO/B-CN/FO) ternary composite. The electrons of B-CN excite to its conduction band while holes remain in its valence band. Due to the proper alignment between LFO and FO components and B-CN, the excited electrons of LFO and FO recombines with the holes of B-CN. Thus, the photogenerated electrons in the conduction band of B-CN have strong reducing power and reduce the adsorbed oxygen to superoxide radicals () (Citation63). Meanwhile, the holes in the valence band of LFO and FO reveal strong enough oxidation potential and oxidize H2O/OH− to hydroxyl radicals (
). These reactive oxygenated species such as
and
further proceed the NB dye degradation reaction and convert it into CO2 and H2O. Thus, the heterojunction follows a double Z-scheme charge transfer mechanism which has strong redox power due to the efficient charge carrier’s separation during the photocatalytic reaction (Citation64). This suggests that the as-fabricated LFO/B-CN/FO photocatalyst can be considered an ideal catalyst for the degradation of organic pollutants (Citation48). The electron–hole pair’s generation, separation and surface redox properties in the as-fabricated ternary composite can be expressed in terms of Equations (12–17).
(12)
(12)
(13)
(13)
(14)
(14)
(15)
(15)
(16)
(16)
(17)
(17)
Scheme 3. Schematic illustration of photocatalytic degradation of NB dye over LFO/B-CN/FO photocatalyst.
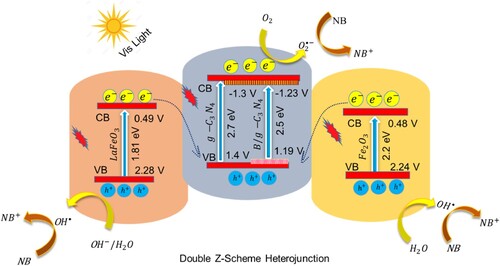
Enhanced charge carrier’s separation and transfer at the interface of LFO/B-CN/FO ternary composite is due to the proper band alignment between the counterparts. This probably reduces the likelihood of photogenerated holes and electrons recombining by fully utilizing them in redox reactions. As a result, the activity as well as stability of the ternary composite photocatalyst remarkably increases (Citation65–Citation68).
Future perspectives
Our future research will explore the application of the same composites for the degradation of a wider range of pollutants, aiming to validate their versatility and effectiveness. Additionally, these composites show potential for clean and sustainable energy solutions, such as CO₂ reduction and water splitting. We plan to investigate their conducting properties and solid-state conductivity. Furthermore, we will assess the applicability of these composites in energy storage devices, such as batteries and supercapacitors. The unique properties of the composites could lead to improved energy storage solutions, offering higher capacity, better stability, and longer lifespans. This broader scope will not only improve environmental remediation but also contribute to advancements in energy technology.
Limitations
The long-term durability of the photocatalysts under actual environmental conditions and the scalability of the hydrothermal synthesis process for industrial applications need to be assessed. The potential for efficiency fluctuations when using the photocatalysts on a larger scale is another drawback of our investigation. Furthermore, results acquired in a laboratory setting under controlled settings may not match the efficiency reported outside in the natural environment. The efficacy of the photocatalysts may be impacted by variables like temperature changes and sunshine intensity in natural settings that is the reason that our trials were carried out in artificial conditions. In order to guarantee consistent and dependable performance, these variables must be thoroughly evaluated and optimized for practical applications. To confirm the adaptability and efficacy of the ternary composite, further research should be carried out to explore its photocatalytic performance on a wider variety of contaminants and under natural environmental real samples.
Conclusion
A one-pot hydrothermal technique was used for the fabrication of CN, B-CN, binary composites (LFO/B-CN, FO/B-CN) and ternary composite (LFO/B-CN/FO). Various characterization techniques such as XRD, FT-IR, SEM, TEM, EDS, TGA/DSC, and UV–visible spectrophotometry were used to evaluate the structural, morphological, and chemical features of the as-fabricated photocatalyst. As obvious, boron doping remarkably reduced the band gap of CN photocatalyst and enhanced its light absorption. Coupling of LFO and FO significantly promoted charge carrier’s separation and transfer and also further improved the optical absorption of B-CN photocatalyst. The resultant LFO/B-CN/FO ternary composite degraded approximately 98% of navy-blue dye after visible light irradiation for 130 min at pH 7 and 35 °C for 50 ppm solution (100 ml). The photodegradation results followed pseudo-first and pseudo-second-order kinetics models. Under ambient conditions, all photocatalysts revealed pH values higher than their pHpzc, indicating that they have negative charges. Thermodynamics studies revealed that the dye degradation over CN, B-CN, and LFO/B-CN is nonspontaneous, while spontaneous for FO/B-CN and LFO/B-CN/FO. Reusability and recycling tests revealed that the catalyst is highly stable, reusable, and completely recoverable from the reaction system over the course of eight consecutive cycles. This research highlights the potential of designing ternary heterojunctions as highly efficient photocatalysts for degrading environmental persistent pollutants.
Supporting_Information_03072024_clean.docx
Download MS Word (2.1 MB)Acknowledgments
The authors are grateful to Abdul Wali Khan University Mardan, Pakistan, and Prince Sultan University, Riyadh, Saudi Arabia for the overall support during this work. Authors would like to thank Prince Sultan University for paying the Article Publication Charges. All authors have contributed to this study at different stages. Wali Muhammad, Abbas Khan, and Sajjad Hussain: study design, method design, analytical protocol design, writing, reviewing, and editing. Hammad Khan and Rasha A. Abumousa: experimental assistant, discussion during writing, and reviewing. Iltaf Khan and Sikandar Iqbal: experimental assistant, activities, and data analysis. Rasha A. Abumousa, Mohamed Bououdina, and Muhammad Humayun: experimental assistant, discussion during writing, reviewing, and editing. All authors read and approved the final version of this manuscript.
Disclosure statement
No potential conflict of interest was reported by the author(s).
Additional information
Funding
References
- Muhammad, W.; Hussain, S.; Khan, A.; Khan, H.; Khan, N.; Khan, S.U.; Ali, S.; Bououdina, M.; Humayun, M. Novel Magnetite/Persulphate/Ozone Hybrid System for Catalytic Degradation/Ozonation of Sunset Yellow Dye from Wastewater. Nanocomposites 2024, 10 (1), 122–138. https://doi.org/10.1080/20550324.2024.2335694
- He, Y.; Wang, S.; Chen, N. Mineral Rents, Natural Resources Depletion, and Ecological Footprint Nexus in High Emitting Countries: Panel GLM Analysis. Resour. Policy 2024, 89, 104472. https://doi.org/10.1016/j.resourpol.2023.104472
- Nayak, A.; Chaudhary, P.; Bhushan, B.; Ghai, K.; Singh, S.; Sillanpää, M. Removal of Emergent Pollutants: A Review on Recent Updates and Future Perspectives on Polysaccharide-Based Composites Vis-à-Vis Traditional Adsorbents. Int. J. Biol. Macromol. 2024, 258, 129092. https://doi.org/10.1016/J.IJBIOMAC.2023.129092
- Du, Y.; Zhao, Q.; Liu, R.; Jiang, T. Preparation of g-C3N4 Nanosheet/WO3/Graphene Oxide Ternary Nanocomposite Z-Scheme Photocatalyst with Enhanced Visible Light Photocatalytic Activity. J. Clust. Sci. 2023, 34 (1), 273–283. https://doi.org/10.1007/s10876-022-02221-w
- Yuan, Y.; Guo, R.T.; Hong, L.F.; Ji, X.Y.; Lin, Z.D.; Li, Z.S.; Pan, W.G. A Review of Metal Oxide-Based Z-Scheme Heterojunction Photocatalysts: Actualities and Developments. Mater. Today Energy 2021, 21, 100829. https://doi.org/10.1016/j.mtener.2021.100829
- Hao, P.; Chen, Z.; Yan, Y.; Shi, W.; Guo, F. Recent Advances, Application and Prospect in g-C3N4-Based S-Scheme Heterojunction Photocatalysts. Sep. Purif. Technol. 2024, 330, 125302. https://doi.org/10.1016/j.seppur.2023.125302
- Ali, S.; Humayun, M.; Pi, W.; Yuan, Y.; Wang, M.; Khan, A.; Yue, P.; Shu, L.; Zheng, Z.; Fu, Q.;, et al. Fabrication of BiFeO3-g-C3N4-WO3 Z-Scheme Heterojunction as Highly Efficient Visible-Light Photocatalyst for Water Reduction and 2,4-Dichlorophenol Degradation: Insight Mechanism. J. Hazard. Mater. 2020, 397, 122708. https://doi.org/10.1016/j.jhazmat.2020.122708
- Mafa, P.J.; Malefane, M.E.; Idris, A.O.; Mamba, B.B.; Liu, D.; Gui, J.; Kuvarega, A.T. Cobalt Oxide/Copper Bismuth Oxide/Samarium Vanadate (Co3O4/CuBi2O4/SmVO4) Dual Z-Scheme Heterostructured Photocatalyst with High Charge-Transfer Efficiency: Enhanced Carbamazepine Degradation under Visible Light Irradiation. J. Colloid Interface Sci. 2021, 603, 666–684. https://doi.org/10.1016/j.jcis.2021.06.146
- Mafa, P.J.; Ntsendwana, B.; Mamba, B.B.; Kuvarega, A.T. Visible Light Driven ZnMoO4/BiFeWO6/RGO Z-Scheme Photocatalyst for the Degradation of Anthraquinonic Dye. J. Phys. Chem. C 2019, 123 (33), 20605–20616. https://doi.org/10.1021/acs.jpcc.9b05008
- Saravanakumar, K.; Park, C.M. Rational Design of a Novel LaFeO3/g-C3N4/BiFeO3 Double Z-Scheme Structure: Photocatalytic Performance for Antibiotic Degradation and Mechanistic Insight. Chem. Eng. J. 2021, 423, 130076. https://doi.org/10.1016/j.cej.2021.130076
- Rajendran, R.; Vignesh, S.; Raj, V.; Palanivel, B.; Ali, A.M.; Sayed, M.A.; Shkir, M. Designing of TiO2/α-Fe2O3 Coupled g-C3N4 Magnetic Heterostructure Composite for Efficient Z-Scheme Photo-Degradation Process under Visible Light Exposures. J. Alloys Compd. 2022, 894, 162498. https://doi.org/10.1016/j.jallcom.2021.162498
- Farooq, N.; ur Rehman, A.; Qureshi, A.M.; ur Rehman, Z.; Ahmad, A.; Aslam, M.K.; Javed, H.M.A.; Hussain, S.; Habila, M.A., AlMasoud, N., et al. Au@GO@g-C3N4 and Fe2O3 Nanocomposite for Efficient Photocatalytic and Electrochemical Applications. Surf Interfaces. 2021, 26, 101399. https://doi.org/10.1016/j.surfin.2021.101399
- Xu, C.; Jin, Z.; Cui, J.; Guo, F.; Hu, R.; Meng, W.; Hu, J.; Shen, X. One Step Grinding Method to Prepare BiFeO3/α-Fe2O3 Type-Ⅱ Heterojunction for Enhancing Phenolic Wastewater Degradation. Mater. Sci. Semicond. Process. 2023, 155, 107242. https://doi.org/10.1016/j.mssp.2022.107242
- Jiao, Y.; Li, Y.; Wang, J.; He, Z.; Li, Z. Novel B-N-Co Surface Bonding States Constructed on Hollow Tubular Boron Doped g-C3N4/CoP for Enhanced Photocatalytic H2 Evolution. J. Colloid Interface Sci. 2021, 595, 69–77. https://doi.org/10.1016/j.jcis.2021.03.134
- Hao, X.; Zhang, Y. Low Temperature Gel-Combustion Synthesis of Porous Nanostructure LaFeO3 with Enhanced Visible-Light Photocatalytic Activity in Reduction of Cr(VI). Mater. Lett. 2017, 197, 120–122. https://doi.org/10.1016/j.matlet.2017.03.133
- Xu, Y.; Huang, S.; Xie, M.; Li, Y.; Xu, H.; Huang, L.; Zhang, Q.; Li, H. Magnetically Separable Fe2O3/g-C3N4 Catalyst with Enhanced Photocatalytic Activity. RSC Adv. 2015, 5 (116), 95727–95735. https://doi.org/10.1039/C5RA18009K
- Simonin, J.P. On the Comparison of Pseudo-First Order and Pseudo-Second Order Rate Laws in the Modeling of Adsorption Kinetics. Chem. Eng. J. 2016, 300, 254–263. https://doi.org/10.1016/j.cej.2016.04.079
- Saad, I.; Ralha, N.; Abukhadra, M.R.; Al Zoubi, W.; Ko, Y.G. Recent Advances in Photocatalytic Oxidation Techniques for Decontamination of Water. J. Water Process Eng. 2023, 52, 103572. https://doi.org/10.1016/j.jwpe.2023.103572
- Aytimur, A.; Koçyiǧit, S.; Uslu, I.; Durmuşoǧlu, Ş; Akdemir, A. Synthesis and Characterization of Boron-Doped Bismuth Oxide-Erbium Oxide Fiber Derived Nanocomposite Precursor. J. Compos. Mater. 2014, 48 (19), 2317–2324. https://doi.org/10.1177/0021998313498100
- Wang, G.; Liu, T.; Luo, Y.; Zhao, Y.; Ren, Z.; Bai, J.; Wang, H. Preparation of Fe2O3/Graphene Composite and Its Electrochemical Performance as an Anode Material for Lithium Ion Batteries. J. Alloys Compd. 2011, 509 (24), L216–L220. https://doi.org/10.1016/j.jallcom.2011.03.151
- Singh, R.; Chauhan, M.; Garg, P.; Sharma, B.; Attri, P.; Sharma, R.K.; Sharma, D.; Chaudhary, G.R. A Critical Review on Visible Light Active Graphitic Carbon Nitride (g-CN) Based Photocatalyst for Environment Remediation Application: A Sustainable Approach. J. Clean. Prod. 2023, 427, 138855. https://doi.org/10.1016/J.JCLEPRO.2023.138855
- Liu, H.; Wu, S.; Wang, Q.; Zheng, Z.; Zhao, H.; Zhao, Y.; Xiao, L.; Cai, J.; Lu, Z., Yin, X., et al. Facile Fabrication of Exfoliated G-C3N4/MWCNTs/Fe3O4 Ternary Composites with Multi-Component Functional Synergy for High-Performance Microwave Absorption. J. Mater. Chem. C 2023, 11 (7), 2552–2564. https://doi.org/10.1039/d2tc05168k
- Li, Z.; Chen, C.; Yang, Q.; Liu, Q.; Zhang, Z.; Fang, X. Modifying the Bridging N Atoms of Polymeric Carbon Nitride to Achieve Highly Enhanced Photocatalytic Hydrogen Evolution. Appl. Surf. Sci. 2020, 530, 147287. https://doi.org/10.1016/j.apsusc.2020.147287
- Karimi, F.; Zare, N.; Jahanshahi, R.; Arabpoor, Z.; Ayati, A.; Krivoshapkin, P.; Darabi, R.; Dragoi, E.N.; Raja, G.G., Fakhari, F., et al. Natural Waste-Derived Nano Photocatalysts for Azo Dye Degradation. Environ. Res. 2023, 238, 117202. https://doi.org/10.1016/j.envres.2023.117202
- Arif, N.; Ma, Y.; Iqbal, M.A.; Zafar, M.N.; Liang, H.; Zhang, Q.; Zeng, Y.J. Enhanced Charge Separation in Dual Z-Scheme Au Decorated LaFeO3-g-C3N4-BiFeO3 System for Efficient H2 Production. Fuel 2023, 336, 126832. https://doi.org/10.1016/j.fuel.2022.126832
- Zhou, Y.; Chai, Y.; Sun, H.; Li, X.; Liu, X.; Liang, Y.; Gong, X.; Wu, Z.; Liu, C.; Qin, P. Design Strategies and Mechanisms of g–C3N4–Based Photoanodes for Photoelectrocatalytic Degradation of Organic Pollutants in Water. J. Environ. Manage. 2023, 344, 118545. https://doi.org/10.1016/j.jenvman.2023.118545
- Balu, S.; Velmurugan, S.; Palanisamy, S.; Chen, S.W.; Velusamy, V.; Yang, T.C.K.; El-Shafey, E.S.I. Synthesis of α-Fe2O3 Decorated g-C3N4/ZnO Ternary Z-Scheme Photocatalyst for Degradation of Tartrazine Dye in Aqueous Media. J. Taiwan Inst. Chem. Eng. 2019, 99, 258–267. https://doi.org/10.1016/j.jtice.2019.03.011
- Knežević, S.; Ostojić, J.; Ognjanović, M.; Savić, S.; Kovačević, A.; Manojlović, D.; Stanković, V.; Stanković, D. The Environmentally Friendly Approaches Based on the Heterojunction Interface of the LaFeO3/Fe2O3@g-C3N4 Composite for the Disposable and Laboratory Sensing of Triclosan. Sci. Total Environ. 2023, 857, 159250. https://doi.org/10.1016/j.scitotenv.2022.159250
- Zhang, W.; Ma, Y.; Zhu, X.; Wang, L.; Ye, J.; Hou, X.; Liu, S.; Lu, M.; Tian, H.; Hu, X. In Situ Synthesis of α-Fe2O3/LaFeO3 Modified with g-C3N4 and Ti3C2 for Construction of Multiple Z-Scheme/Schottky Heterojunctions as an Efficient Visible-Light Photocatalyst for Cr (VI) Reduction and Organic Pollutants Removal. J. Alloys Compd. 2022, 913, 165217. https://doi.org/10.1016/j.jallcom.2022.165217
- Heidari, S.; Haghighi, M.; Shabani, M. Ultrasound Assisted Dispersion of Bi2Sn2O7-C3N4 Nanophotocatalyst over Various Amount of Zeolite Y for Enhanced Solar-Light Photocatalytic Degradation of Tetracycline in Aqueous Solution. Ultrason. Sonochem. 2018, 43, 61–72. https://doi.org/10.1016/j.ultsonch.2018.01.001
- Han, Z.; Wang, N.; Fan, H.; Ai, S. Ag Nanoparticles Loaded on Porous Graphitic Carbon Nitride with Enhanced Photocatalytic Activity for Degradation of Phenol. Solid State Sci. 2017, 65, 110–115. https://doi.org/10.1016/j.solidstatesciences.2017.01.010
- Zheng, T.; Xia, W.; Guo, J.; Wang, K.; Zeng, M.; Wu, Q.; Liu, Y. Preparation of Flame-Retardant Polyamide 6 by Incorporating MgO Combined with g-C3N4. Polym. Adv. Technol. 2020, 31 (9), 1963–1971. https://doi.org/10.1002/pat.4920
- Bankole, O.M.; Olorunsola, T.D.; Ogunlaja, A.S. Photocatalytic Decontamination of Toxic Hexavalent Chromium in Water over Graphitic Carbon Nitride Supported Sulfur Nanoparticles. J. Photochem. Photobiol. A Chem. 2021, 405, 112934. https://doi.org/10.1016/j.jphotochem.2020.112934
- Khan, I.; Yuan, A.; Khan, S.; Khan, A.; Khan, S.; Shah, S.A.; Luo, M.; Yaseen, W.; Shen, X.; Yaseen, M. Graphitic Carbon Nitride Composites with Gold and ZIF-67 Nanoparticles as Visible-Light-Promoted Catalysts for CO2 Conversion and Bisphenol A Degradation. ACS Appl. Nano Mater. 2022, 5 (9), 13404–13416. https://doi.org/10.1021/acsanm.2c03067
- Machrouhi, A.; Khiar, H.; Elhalil, A.; Sadiq, M.; Abdennouri, M.; Barka, N. Synthesis, Characterization, and Photocatalytic Degradation of Anionic Dyes Using a Novel ZnO/Activated Carbon Composite. Watershed Ecol. Environ. 2023, 5, 80–87. https://doi.org/10.1016/j.wsee.2022.12.001
- Onwudiwe, D.C.; Gobile, N.; Oyewo, O.A.; Makgato, S.S. Photocatalytic Reduction of Hexavalent Chromium Using Zn2SnO4–ZnO Modified g-C3N4 Composite. Results Eng. 2023, 20, 101521. https://doi.org/10.1016/J.RINENG.2023.101521
- Roy, H.; Rahman, T.U.; Khan, M.A.J.R.; Al-Mamun, M.R.; Islam, S.Z.; Khaleque, M.A.; Hossain, M.I.; Khan, M.Z.H.; Islam, M.S., Marwani, H.M., et al. Toxic Dye Removal, Remediation, and Mechanism with Doped SnO2-Based Nanocomposite Photocatalysts: A Critical Review. J. Water Process Eng. 2023, 54, 104069. https://doi.org/10.1016/j.jwpe.2023.104069
- Ren, Y.; Wang, J.; Qu, G.; Ren, N.; Lu, P.; Chen, X.; Wang, Z.; Yang, Y.; Hu, Y. Study on the Mechanism of High Effective Mineralization of Rhodamine B in Three Dimensional Electrochemical System with γ-Fe2O3@CNTs Particle Electrodes. Sep. Purif. Technol. 2023, 314, 120487. https://doi.org/10.1016/j.seppur.2023.123616
- Khan, H.; Hussain, S.; Zahoor, R.; Arshad, M.; Umar, M.; Marwat, M.A.; Khan, A.; Khan, J.R.; Haleem, M.A. Novel Modeling and Optimization Framework for Navy Blue Adsorption onto Eco-Friendly Magnetic Geopolymer Composite. Environ. Res. 2023, 216, 114346. https://doi.org/10.1016/j.envres.2022.114346
- Iqbal, S.; Ahmad, N.; Javed, M.; Qamar, M.A.; Bahadur, A.; Ali, S.; Ahmad, Z.; Irfan, R.M.; Liu, G., Akbar, M.B., et al. Designing Highly Potential Photocatalytic Comprising Silver Deposited ZnO NPs with Sulfurized Graphitic Carbon Nitride (Ag/ZnO/S-g-C3N4) Ternary Composite. J. Environ. Chem. Eng. 2021, 9 (1), 104919. https://doi.org/10.1016/j.jece.2020.104919
- Naseer, A.; Nosheen, S.; Kiran, S.; Kamal, S.; Javaid, M.A.; Mustafa, M.; Tahir, A. Degradation and Detoxification of Navy Blue CBF Dye by Native Bacterial Communities: An Environmental Bioremedial Approach. Desalin. Water Treat. 2016, 57 (50), 24070–24082. https://doi.org/10.1080/19443994.2016.1138145
- Taoufik, N.; Sadiq, M.; Abdennouri, M.; Qourzal, S.; Khataee, A.; Sillanpää, M.; Barka, N. Recent Advances in the Synthesis and Environmental Catalytic Applications of Layered Double Hydroxides-Based Materials for Degradation of Emerging Pollutants through Advanced Oxidation Processes. Mater. Res. Bull. 2022, 154, 111924. https://doi.org/10.1016/j.materresbull.2022.111924
- Habibi, M.H.; Hassanzadeh, A.; Mahdavi, S. The Effect of Operational Parameters on the Photocatalytic Degradation of Three Textile Azo Dyes in Aqueous TiO2 Suspensions. J. Photochem. Photobiol. A Chem. 2005, 172 (1), 89–96. https://doi.org/10.1016/j.jphotochem.2004.11.009
- Suhan, M.B.K.; Al-Mamun, M.R.; Farzana, N.; Aishee, S.M.; Islam, M.S.; Marwani, H.M.; Hasan, M.M.; Asiri, A.M.; Rahman, M.M., Islam, A., et al. Sustainable Pollutant Removal and Wastewater Remediation Using TiO2-Based Nanocomposites: A Critical Review. Nano-Struct. Nano-Objects 2023, 36, 101050. https://doi.org/10.1016/J.NANOSO.2023.101050
- Mamatha, K.M.; Srinivasa Murthy, V.; Thammanna, B.M.; Naveen Kumar, T.; Jahagirdar, A.A.; Naveen Kumar, A.; Muniyappa, M.; Ravikumar, C.R.; Ananda Murthy, H.C. Electrochemical Sensor of Carboxymethyl Cellulose and Photocatalytic Degradation of Navy Blue Dye by Sonochemically Synthesized Titanium Oxide Nanoparticles. Sensors Int. 2023, 4, 100239. https://doi.org/10.1016/j.sintl.2023.100239
- Li, J.; Zhu, X.; Qiu, F.; Zhang, T.; Hu, F.; Peng, X. Facile Preparation of Ag/Ag2WO4/g-C3N4 Ternary Plasmonic Photocatalyst and Its Visible-Light Photocatalytic Activity. Appl. Organomet. Chem. 2019, 33 (3), e4683. https://doi.org/10.1002/aoc.4683
- Saha, S.; Chaudhary, N.; Kumar, A.; Khanuja, M. Polymeric Nanostructures for Photocatalytic Dye Degradation: Polyaniline for Photocatalysis. SN Appl. Sci. 2020, 2 (6), 1–10. https://doi.org/10.1007/s42452-020-2928-4
- Cui, J.; Xu, C.; Jin, Z.; Liu, H.; Hu, R.; Liu, F. Visible Light Photocatalysis: Efficient Z-Scheme LaFeO3/g-C3N4/ZnO Photocatalyst for Phenol Degradation. Environ. Sci. Pollut. Res. 2023, 30 (43), 96875–96890. https://doi.org/10.1007/s11356-023-29199-w
- Tahir, S.; Zahid, M.; Hanif, M.A.; Javed, M.Y. G-C3N4/Graphene Oxide/SnFe2O4 Ternary Composite for the Effective Sunlight-Driven Photocatalytic Degradation of Methylene Blue. Environ. Sci. Pollut. Res. Int. 2023, 30 (60), 125540–125558. https://doi.org/10.1007/s11356-023-31096-1
- Waheed, Z.; Ghazanfar, S.; Usman, M.; Asif, H.M.; Tariq, M.; Mahmood, K.; Haider, A.; Sirajuddin, M. Synthesis And Characterization Of Ternary Composite g-C3N4-Wo3/Rgo For Photocatalytic Activity In Degradation Of Methylene Blue. Bull. Chem. Soc. Ethiop. 2023, 37 (5), 1123–1131. https://doi.org/10.4314/bcse.v37i5.5
- Zhang, W.; Xu, D.; Wang, F.; Chen, M. AgCl/Au/g-C3N4 Ternary Composites: Efficient Photocatalysts for Degradation of Anionic Dyes. J. Alloys Compd. 2021, 868, 159266. https://doi.org/10.1016/j.jallcom.2021.159266
- Qamar, M.A.; Shahid, S.; Javed, M.; Shariq, M.; Fadhali, M.M.; Madkhali, O.; Ali, S.K.; Syed, I.S.; Awaji, M.Y., Shakir Khan, M., et al. Accelerated Decoloration of Organic Dyes from Wastewater Using Ternary Metal/g-C3N4/ZnO Nanocomposites: An Investigation of Impact of g-C3N4 Concentration and Ni and Mn Doping. Catalysts 2022, 12 (11), 1–18. https://doi.org/10.3390/catal12111388
- Saher, R.; Hanif, M.A.; Mansha, A.; Javed, H.M.A.; Zahid, M.; Nadeem, N.; Mustafa, G.; Shaheen, A.; Riaz, O. Sunlight-Driven Photocatalytic Degradation of Rhodamine B Dye by Ag/FeWO4/g-C3N4 Composites. Int. J. Environ. Sci. Technol. 2021, 18 (4), 927–938. https://doi.org/10.1007/s13762-020-02888-6
- Saratale, R.G.; Saratale, G.D.; Chang, J.S.; Govindwar, S.P. Decolorization and Biodegradation of Textile Dye Navy Blue HER by Trichosporon Beigelii NCIM-3326. J. Hazard. Mater. 2009, 166 (2–3), 1421–1428. https://doi.org/10.1016/j.jhazmat.2008.12.068
- Shekardasht, M.B.; Givianrad, M.H.; Gharbani, P.; Mirjafary, Z.; Mehrizad, A. Preparation of a Novel Z-Scheme g-C3N4/RGO/Bi2Fe4O9 Nanophotocatalyst for Degradation of Congo Red Dye under Visible Light. Diam. Relat. Mater. 2020, 109, 108008. https://doi.org/10.1016/j.diamond.2020.108008
- El Jabbar, Y.; ElHafdi, M.; Benchikhi, M.; El Ouatib, R.; Er-Rakho, L.; Essadki, A. Photocatalytic Degradation of Navy Blue Textile Dye by Nanoscale Cobalt Aluminate Prepared by Polymeric Precursor Method. Environ. Nanotechnol. Monit. Manag. 2019, 12, 100259. https://doi.org/10.1016/j.enmm.2019.100259
- Bao, J.; Jiang, X.; Huang, L.; Quan, W.; Zhang, C.; Wang, Y.; Wang, H.; Zeng, Y.; Zhang, W., Ma, Y., et al. Molybdenum Disulfide Loading on a Z-Scheme Graphitic Carbon Nitride and Lanthanum Nickelate Heterojunction for Enhanced Photocatalysis: Interfacial Charge Transfer and Mechanistic Insights. J. Colloid Interface Sci. 2022, 611, 684–694. https://doi.org/10.1016/j.jcis.2021.12.106
- Zhang, W.; Ma, Y.; Zhu, X.; Liu, S.; An, T.; Bao, J.; Hu, X.; Tian, H. Fabrication of Ag Decorated G-C3N4/LaFeO3 Z-Scheme Heterojunction as Highly Efficient Visible-Light Photocatalyst for Degradation of Methylene Blue and Tetracycline Hydrochloride. J. Alloys Compd. 2021, 864, 158914. https://doi.org/10.1016/j.jallcom.2021.158914
- Tian, H.; Liu, M.; Zheng, W. Constructing 2D Graphitic Carbon Nitride Nanosheets/Layered MoS2/Graphene Ternary Nanojunction with Enhanced Photocatalytic Activity. Appl. Catal. B Environ. 2018, 225, 468–476. https://doi.org/10.1016/j.apcatb.2017.12.019
- Debnath, S.; Das, R. Strong Adsorption of CV Dye by Ni Ferrite Nanoparticles for Waste Water Purification: Fits Well the Pseudo Second Order Kinetic and Freundlich Isotherm Model. Ceram. Int. 2023, 49 (10), 16199–16215. https://doi.org/10.1016/j.ceramint.2023.01.218
- Raizada, P.; Singh, P.; Kumar, A.; Sharma, G.; Pare, B.; Jonnalagadda, S.B.; Thakur, P. Solar Photocatalytic Activity of Nano-ZnO Supported on Activated Carbon or Brick Grain Particles: Role of Adsorption in Dye Degradation. Appl. Catal. A Gen. 2014, 486, 159–169. https://doi.org/10.1016/j.apcata.2014.08.043
- Palanivel, B.; Mani, A. Conversion of a Type-II to a Z-Scheme Heterojunction by Intercalation of a 0D Electron Mediator between the Integrative NiFe2O4/g-C3N4Composite Nanoparticles: Boosting the Radical Production for Photo-Fenton Degradation. ACS Omega 2020, 5 (31), 19747–19759. https://doi.org/10.1021/acsomega.0c02477
- Edwin Malefane, M.; John Mafa, P.; Thokozani Innocent Nkambule, T.; Elizabeth Managa, M.; Tawanda Kuvarega, A. Modulation of Z-Scheme Photocatalysts for Pharmaceuticals Remediation and Pathogen Inactivation: Design Devotion, Concept Examination, and Developments. Chem. Eng. J., 2023, 452 (P2), 138894. https://doi.org/10.1016/j.cej.2022.138894
- Malefane, M.E.; Feleni, U.; Mafa, P.J.; Kuvarega, A.T. Fabrication of Direct Z-Scheme Co3O4/BiOI for Ibuprofen and Trimethoprim Degradation under Visible Light Irradiation. Appl. Surf. Sci. 2020, 514, 145940. https://doi.org/10.1016/j.apsusc.2020.145940
- Qi, K.; Cui, N.; Zhang, M.; Ma, Y.; Wang, G.; Zhao, Z.; Khataee, A. Ionic Liquid-Assisted Synthesis of Porous Boron-Doped Graphitic Carbon Nitride for Photocatalytic Hydrogen Production. Chemosphere 2021, 272, 129953. https://doi.org/10.1016/j.chemosphere.2021.129953
- Zayan, J.M.; Rasheed, A.K.; John, A.; Khalid, M.; Ismail, A.F.; Aabid, A.; Baig, M. Investigation on Rheological Properties of Water-Based Novel Ternary Hybrid Nanofluids Using Experimental and Taguchi Method. Materials 2022, 15 (1), 28. https://doi.org/10.3390/ma15010028
- Khan, M.U.A.; Razak, S.I.A.; Arjan, W.S.A.; Nazir, S.; Anand, T.J.S.; Mehboob, H.; Amin, R. Recent Advances in Biopolymeric Composite Materials for Tissue Engineering and Regenerative Medicines: A Review. Molecules 2021, 26 (3), 619. https://doi.org/10.3390/molecules26030619
- Zayan, J.M.; Rasheed, A.K.; John, A.; Faris, W.F.; Aabid, A.; Baig, M.; Alallam, B. Synthesis and Characterization of Novel Ternary-Hybrid Nanoparticles as Thermal Additives. Materials 2023, 16 (1), 173. https://doi.org/10.3390/ma16010173