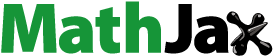
Abstract
Aiming to sequestrate the excessive carbon dioxide and convert the acidified seawater, an improved method of carbon dioxide mineralization is developed based on electrode separation mechanism and extra oxygen-supplying technique. By electrode separation the neutralizations of the anodic acidity and the cathodic alkalinity, as well as the precipitation and the dissolution of calcium carbonate (CaCO3), are prevented. In addition, the extra-supplied oxygen prevents the evolution of hydrogen, which enhances the electric conductivity of the porous cathode and the deposition of CaCO3. A series of indoor physical experiments were conducted and the results show that the acidified seawater was successfully converted to alkaline in 72h. The speed of carbon mineralizing sequestration is significantly enhanced by supplying extra oxygen. The carbon dioxide mineralization speed increases with the immerse ratio of the aerator due to the more reacted oxygen and the less hydrogen evolution, which gives more porous space in the cathode for more conductive seawater and more deposition of CaCO3. The extra-supplied oxygen increases the CaCO3 -deposition by 100-214% under excessive atmospheric- CO2 conditions and 117-200% under normal atmospheric- CO2 conditions, respectively. This method has an application potential for quick conversion of locally acidified seawater in emergent circumstances.
Introduction
In coastal areas where fossil-fuel-fired power stations, petrochemical works, and cement manufacturing plants are located, excessive atmospheric CO2 usually impacts atmospheric and ocean environments [Citation1]. Ocean mixing, upwelling, downwelling, and circulation can bring excessive carbon to some local ocean sites, which usually causes seawater acidification [Citation2,Citation3]. This will cause the decomposition of marine shells and a decline in fishery resources, thus endangering coastal and marine ecosystems [Citation4–12]. In addition to increasing energy efficiency, switching to less carbon-intensive energy sources, and absorbing and utilizing CO2 onshore for carbon reduction [Citation13], turning to the marine carbon sink is also an important option [Citation14–16]. Quick carbon reduction and sequestration at the locally acidified marine site is a crucial method of environmental protection and is essential for the recovery and sustainable development of the marine ecosystem.
As an efficient method of carbon reduction, marine carbon sequestration is developing rapidly because the marine carbon sink is the most significant on Earth, with a storage capacity of up to 93% of the global carbon [Citation17]. There are three predominant methods of marine carbon sequestration: sequestration by geological injection, sequestration by biological utilization , and sequestration by ionic mineralization.
The capacity of the global marine realm to contain CO2 by geological injection sequestration is estimated at 400–10,000 × 108 tons, reaching about 1.33–33.33 times the annual global atmospheric emission of 340 × 108 tons [Citation18–20]. The concept of seabed sequestration was first proposed for injecting CO2 into thermohaline currents and then being carried and spread into the deep ocean [Citation21]. After that, various onshore and offshore geological sequestration techniques have been greatly improved with the additional step of injecting CO2 into subsurface porous rock formations [Citation22–25]. By this method, the injected CO2 is mainly captured from the atmosphere or immediately from emission, instead of from the seawater directly.
Sequestration involving marine biological use can reduce the CO2 concentration in the seawater directly. Corallines utilize a large amount of CO2 in their photosynthesis and thus decrease CO2 concentration and convert acidified seawater. Coral reefs are estimated to have the ability to sequester 900 million tons of carbon annually [Citation26]. Reef formation is accompanied by a certain amount of mineral deposition of calcium carbonate (CaCO3) [Citation27,Citation28]. However, in acidified marine environments, the corallines and coral reefs tend to die or become bleached [Citation29], which will cause them to lose their ability to sequester carbon.
In recent years, marine carbon mineralizing sequestration has become an efficient method of carbon reduction and marine acidification conversion [Citation30–33]. The main advantage of this method is brought about by the abundant marine calcium ions (Ca+), which chemically react with the bicarbonate ions (HCO3−) and are sufficient in limiting the decrease in concentration so as not to cause significant negative effects on the marine ecosystem [Citation20,Citation31]. However, the reduction reaction of Ca+ and HCO3− is difficult to produce in acidified marine environments. It usually requires a huge amount of alkaline reagent [Citation18,Citation34], which limits its application due to the possible negative impact of the added alkaline reagents on marine ecology.
Compared with throwing alkaline reagents into the ocean, a preferable method is to electrolyze the seawater to create a strong local alkaline environment at the cathode. The cathode-precipitated CaCO3 can be sequestered permanently and utilized as an excellent bio-cement or other construction material due to its merits of high strength, self-growth, and uniform thickness. Therefore, the electrochemical precipitation technique of CaCO3 has been developed further since first being proposed in the 1940s [Citation35–38]. Regarding bulk carbon sequestration, however, its effect is neutral because the acidity created at the anode would increase the dissolution of CaCO3 and release CO2 simultaneously. Theoretically, separating the anode from the marine cathode by placing it in its own container will prevent the neutralization of anodic acidity and cathodic alkalinity. Thus, this will lead to the precipitation and dissolution of CaCO3 in the seawater – but research on this technique is rarely reported.
Based on the electrode-separating technique, an improved method of electrochemical CO2 mineralization for carbon sequestration in acidified seawater is proposed in this study. The proposed method uses active carbon felt as the electrodes due to its high electrical conductivity and porous nature, allowing easy deposition of CaCO3. The anode is placed in a separate container and connected with the marine cathode through a seawater corridor to prevent the neutralization of acidity/alkalinity of the seawater. In addition, an extra oxygen-supplying device, in the form of a wave-current-driven aerator, is introduced to increase the speed of carbon sequestration. The main function of the aerator is to prevent the evolution of hydrogen and thus to improve the electrical conductivity and the speed of CaCO3 deposition on the cathode. Compared to previous work, the comprehensive utilization of the active carbon electrodes, the electrode-separation technique, and the oxygen-supplying device are new developments. Because the material of the electrodes is easily obtained, and because the electrode separation technique and the oxygen supplying technique are both easily applied in real ocean sites, this newly proposed method is expected to enhance the speed of carbon removal and sequestration in real acidified seawater. To investigate the effects and the efficiency of this method, a series of indoor experiments were conducted with a permeable cylinder installed underwater as the wave-current driven aerator.
Theoretical background
Carbon mineralizing sequestration by electrode separation technique
Carbon mineralizing sequestration by cathodic precipitation of CaCO3 is based on the electrochemical deposition technique. In this technique, the water molecule is firstly electrolyzed to generate around the cathode, and then the abundant Ca + ions and
ions in the seawater undergo a synthesis reaction with
ions to precipitate CaCO3 and deposit it on the cathode [Citation36–38].
In a normal marine environment where no extra oxygen is supplied, the electrolysis of water produces ions and hydrogen gas (
) at the cathode. The electrolytic reaction is:
(1)
(1)
Then the ions locally combined by negative charge (
) undergo a reduction reaction with
and
to precipitate
The reaction is:
(2)
(2)
It should be noted that the acidified marine water is still alkaline (pH > 7), which allows the above reactions (EquationEquations (1)–(2)) to occur. And the ions can be compensated for by continuous electrolysis of water (EquationEquation (1)
(1)
(1) ).
Simultaneously, the electrolysis of water produces ions and oxygen gas (
) at the anode, and then these
ions dissolve in the seawater until they react with
to produce
ions. These two reactions are:
(3)
(3)
(4)
(4)
Consequently, in the situation where both electrodes are placed in one electrolyte of the seawater, the net reaction is neutral regarding marine pH and alkalinity, as well as precipitation and dissolution of in the seawater [Citation36–38]. In this study, however, the anode is separated and placed in its own container to limit the anodic
and
ions in the container; therefore, the net precipitation of
on the cathode in acidified seawater can occur.
Acceleration of reaction and deposition with extra oxygen supply
In a situation where extra oxygen is involved, the cathodic electrolysis reaction of the seawater prevents the evolution of hydrogen, which exposes more porous space of the active carbon cathode to the seawater. This therefore enhances the electrical conductivity of the cathode and the deposition rate of The reaction is [Citation39]:
(5)
(5)
Then, the reaction of the dissolved marine CO2 and ions is accelerated to generate
more quickly; this reaction is:
(6)
(6)
Simultaneously, the precipitation rate of is enhanced (EquationEquation (2)
(2)
(2) ). As a result, the speed of carbon reduction and the mineralizing sequestration of
in acidified seawater increases. It should be noted that in normal acidified seawater and under normal air–sea exchange conditions, there is no quick creation of
and no disturbance on the sea surface. Therefore, Equation (6) is limited by low dissolved
concentration and slow re-equilibration from the air. With the method proposed in this work, the aerator accelerates the creation of
and improves the exchange of air–seawater, and thus enhances Equation (6).
Experimental set-up
The experimental set-up comprises a water flume, an individual anode container, a pair of electrodes, a set of direct electric current supply devices, and a set of aerators. The indoor rectangular recycling water flume measures 8 m long, 0.3 m wide, and 0.4 m high. Two water pools were installed, at the upstream and downstream ends of the water flume, to adjust and regulate the water level in the flume. The experimental water was driven by a pump installed in the recycling layer. To model excessive atmospheric conditions, a 0.25 m high arched top cover was installed over the flume. The cover and the flume were bound with rubber bands. Two 0.05 m diameter holes were created in the cover to inject air with excess
Side views of the experimental set-up are shown in .
Figure 1. (a) Schematic of the water flume in side view. (b) Photograph of the water flume. (c) Schematic of the water flume system, top view.
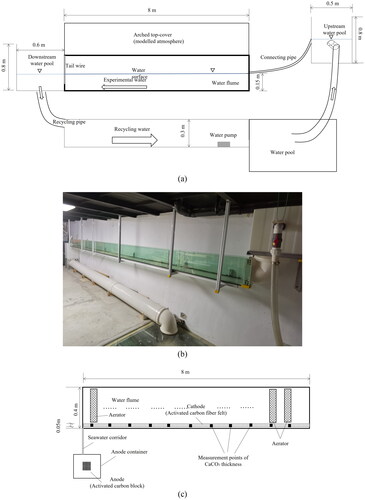
The electrolysis device comprises a pair of electrodes. The cathode was installed inside the water flume, and the anode was put in a container outside. The water flume with the cathode and the container with the anode were interconnected at the downstream end by a water corridor. The material of both electrodes was activated carbon fiber with a specific surface area of 1500 m2/g, a specific resistance of 720·cm, and a specific capacitance of 162 F/g. The cathode was made of a piece of felt 7.5 m long, 0.15 m high, and 0.05 m thick and was installed starting 0.5 m from the upstream end of the water flume and parallel to the side wall. A wood frame was set up to support the cathode felt. The anode comprised a 10 cm3 cubic block that was placed outside in a cubic container measuring 25 cm3. The water flume and the container were connected by a water corridor at the downstream end of the water flume ().
The aerator was designed in the form of a permeable cylinder, which is easily driven by marine waves and currents (). The permeable cylinder has a diameter of 0.3 m and a length of 0.24 m (). A series of 12 aerators were installed parallelly underwater along the side wall of the water flume, at uniform intervals of 0.3 m ().
Eight sets of experiments were conducted at an indoor temperature of 20 °C. Three immersion ratios of the aerator under two atmospheric CO2 conditions, and two sets of control tests without an aerator, were carried out (). The initial atmospheric CO2 was set at an excessive concentration of in the A test series and was set at a normal concentration of
in the B test series. Tests A1–A3 and B1–B3 were carried out to investigate the effects and efficiency of marine pH improvement and carbon mineralizing sequestration. Control tests A0 and B0 were used to investigate the effects and efficiency of the set-up without an aerator, under normal and excessive atmospheric CO2 concentrations, respectively. Each experimental test was repeated three times, each time with a new cathode and anode. Accurate initial atmospheric
values for all the tests are shown in . In the experimental test, the two holes on the top cover of the water flume were sealed.
Table 1. Properties of the aerators and initial atmospheric concentration.
The experimental water was taken from the East China Sea, and sediment particles of diameter greater than 55 μm were filtered out. The pH and composition of the collected seawater, including the concentrations of bicarbonate ions (), carbonate ions (
), and carbon dioxide (
), are shown in . During the experimental tests, calcium chloride (
was added into the water flume every 5 min to create a calcium ion solution and maintain the concentration of
within the range of 0.01–0.015 mol/L. The seawater in the anode container was neutralized using an alkaline reagent (
) every 20 min and was changed every hour. It should be noted that the calcium ions are abundant in the real ocean and thus did not need to be replenished to the cathode area artificially.
Table 2. Marine pH and composition of seawater (mol/L).
The original pH of the experimental seawater was 8.1, indicating a healthy alkaline marine environment. Before every experimental test, CO2 was injected into the experimental water to decrease the marine pH to 7.5 and create an acidified marine environment.
For all the experiments, a direct electric current of 1.5 V was supplied to the electrodes, flow velocity was set at 0.35 m/s, and the amplitude of the seawater wave was 0.05 m. The total thickness of deposited on two sides of the cathode was measured ultrasonically. Ten measurement points were set along the center line of the cathode fiber felt (). The interval between two neighboring measurement points was 0.068 m.
Marine pH and deposition were expected to increase over time in the experiment due to supplying extra oxygen and the continual conversion of CO2 to
But the conversion rate would vary with time because the
covering the cathode would cause a change in the electric current. Therefore, marine pH was measured every 2 h during the first 12 h, and then measured every 4 h from the 12th hour to the 36th hour, and then every 6 h from the 36th hour to the 72nd hour.
Results
The carbon mineralization system performs carbon sequestration at different speeds under both conditions with and without an aerator. This process is accompanied by pH variation under the counter-balanced effects of the flow, wave, and aerator in squeezing out CO2 and precipitating in the seawater. Therefore, the pH variation and the
deposition were simultaneously observed in the experiments. In addition, the final atmospheric CO2 in every experimental test was observed to investigate the mechanism of the electro-chemical carbon mineralization system in carbon reduction.
Marine pH variation
Marine pH variation of the seawater was observed under two aerator conditions (with and without an aerator) and two atmospheric CO2 conditions.
Marine pH variation in a carbon mineralization system with an aerator
The variation in the marine pH of the six experimental tests of carbon mineralization with an aerator are shown in , and the rate of improvement in pH over 72 h is shown in . and Citation4 show that under the double atmospheric CO2 condition, marine pH increases from 7.5 to 7.81, 7.84 and 7.88 after 72 h at aerator immersion ratios of 0.18, 0.35 and 0.5, respectively. In addition, the marine pH improvement rate increases with the immersion ratio of the aerator, reaching 4.13%, 4.53%, and 5.07% at the three respective immersion ratios.
and Citation4 show that under normal atmospheric CO2 conditions, the proposed method increases marine pH more significantly and thus converts the same originally acidified seawater to a more healthy alkaline environment. At the three aerator immersion ratios of 0.18, 0.35, and 0.5, marine pH increases from 7.5 to 7.92, 7.97, and 8.08 after 72 h, and the improvement rate of marine pH increases with the aerator immersion ratio as well, reaching 5.6%, 6.27%, and 7.73%, respectively.
A comparison of and b shows the speed of marine pH improvement is much higher under normal atmospheric CO2 conditions () than at double atmospheric CO2 conditions (). The marine pH improvement rate increases by 36.6%, 38.4% and 52.5% at the three immersion ratios of 0.18, 0.35 and 0.5, respectively. The main reason is that the continually supplied excess CO2 releases more (Equation (7)), which causes the slower alkalization of the acidified seawater.
Marine pH variation in carbon mineralization system without an aerator
shows the variation of marine pH by the normal electro-chemical carbon mineralizing technique without an aerator. Marine pH increases from 7.5 to 7.62 after 72 h under double atmospheric CO2 conditions, an improvement of 1.6%. Under normal atmospheric CO2 conditions, in contrast, marine pH increases from 7.5 to 7.73 after 72 h, improved by 3.1%. Although marine pH is improved under both atmospheric CO2 conditions, the normal electrochemical carbon mineralizing technique occurs at a lower speed than that with the aerator ().
A comparison of indicates the introduction of an aerator increases the marine pH improvement speed significantly, increasing it by 158–216% under excessive carbon emission conditions and by 83–153% under normal carbon emission conditions.
Sequestration of 

The thickness of deposition on the cathode, which represents the carbon sequestration in the seawater, was observed under two aerator conditions (with and without an aerator) and two atmospheric CO2 conditions.
Deposition of 
in a carbon mineralization system with an aerator
The variation of cathodic deposition with time is shown in , and the final thickness of deposition in 72 h is shown in . and Citation6 show that under the double atmospheric CO2 condition, the sequestered
reaches 1.4, 1.8 and 2.2 mm in 72 h at an aerator immersion ratio of 0.18, 0.35 and 0.5, respectively.
Furthermore, and Citation6 show that under normal atmospheric CO2 conditions, the deposition is less than that under double atmospheric CO2 conditions, reaching 1.3, 1.5, and 1.8 mm after 72 h at aerator immersion ratios of 0.18, 0.35 and 0.5, respectively. In addition, under both atmospheric conditions, the
deposition increases with the immersion ratio of the aerator ( and and Citation6).
Deposition of 
in a carbon mineralization system without an aerator
and Citation6 show the variation of thickness using the normal electrochemical carbon mineralization technique without an aerator. The deposited
reaches 0.7 mm after 72 h under double atmospheric CO2 conditions, whereas it reaches 0.6 mm under normal atmospheric CO2 conditions. A comparison of indicates the introduction of an aerator improves the carbon sequestration speed by 100–214% under double atmospheric CO2 conditions, and by 117–200% under normal carbon atmospheric conditions.
Contrary to the marine pH improvement efficiency, carbon sequestration speed is higher under double atmospheric CO2 conditions than that under normal atmospheric CO2 conditions at three aerator immersion ratios and without an aerator ( and Citation6). The higher sequestration might be caused by the higher amount of CO2 dissolved in the seawater, corresponding to the greater atmospheric CO2, which precipitates more under the double atmospheric CO2 condition.
Variation of atmospheric CO2
The final atmospheric CO2 concentration after 72 h in the experiments is shown in . In tests A0–A3, the final atmospheric CO2 concentration is decreased by 25.8–41.9%. In tests B1–B3, the final atmospheric CO2 concentration is reduced by 0–0.79%, but in test B0, it increases slightly (by 0.79%). In both A and B test series, the atmospheric CO2 reduction increases with the immersion ratio of the aerator. This indicates that the main function of the aerator is to supply extra oxygen to the seawater to participate in the electrolysis reaction of water, instead of squeezing out CO2 from the seawater.
Discussion
The proposed carbon mineralization method in this study is validated as effective and efficient in carbon reduction and carbon sequestration in acidified seawater. To understand the mechanism and develop this method further, four points should be noted:
Both the marine pH improvement rate and the
deposition rate decrease after 12 h of experiment time ( and ). This might be caused by decreased electric current passing through the deposited cathode since the
is a poor conductor [Citation37]. Although in the previous marine-site experiments, the cathode was not found to decrease its conductivity due to the generated
creating pores on the cathode (EquationEquation (1)
(1)
(1) ) [Citation38], the extra oxygen supplied in this study restricts the evolution of
(EquationEquation (5)
(5)
(5) ). Therefore, the less porous
may decrease the cathodic conductivity after deposition has covered a large part of the cathodic surface.
The generated
or
ions at the anode still need to be neutralized or utilized in the separated container. The electrode-separation technique limits the negative effects of the neutralization outside the seawater. In addition, the utilization of
and
as well as their chemical production (
) is more applicable outside the seawater [Citation32–40].
Because the speeds of pH improvement and carbon sequestration increase with the immersion ratio of the aerator, the optimized installation of the aerator should consider the maximum immersion ratio at which the aerator can be driven by marine waves and currents.
In real ocean application, the electrode material needed per ton of sequestered
will depend on the specific surface area, specific resistance and specific capacitances of the active carbon fiber, because these three factors affect the conductivity and, therefore, the rate and amount of CaCO3 deposition. The ranges of these three factors are wide; therefore, how much cathodic material is needed has to be evaluated according to the specific materials in use.
The efficiency changes with deposition build-up due to changes in specific surface area, specific resistance, and specific capacitance of the active carbon fiber. Therefore, the efficiency also needs to be evaluated according to the specific materials when used in real ocean applications.
Conclusions
The proposed method is based on the comprehensive utilization of active carbon fiber electrode material, the electrode-separating technique, and the oxygen-supplying technique. This method is validated as effective for quick carbon reduction and sequestration in acidified seawater through indoor water-flume experiments. The main conclusions are the following:
By the proposed method, net deposition of
occurs in the acidified seawater, which is accompanied by marine pH improvement due to consumption of
and thus the method is effective in carbon reduction and carbon sequestration.
The aerator enhances carbon sequestration significantly, with the fundamental mechanism being that the extra oxygen prevents the evolution of hydrogen and thus increases the electrical conductivity and the cathodic
deposition;
The carbon sequestration rate increases with the immersion ratio (
) of the aerator due to the increased oxygen supply, therefore the optimal installation of the aerator in the site should consider the maximum
at which the wave and current can drive the aerator.
Due to its merits of quick removal of carbon and easy site implementation, the proposed method has application potential for quick carbon reduction and carbon sequestration in the emergent circumstances of locally acidified seawater. In addition, this method sequesters the marine on the active carbon fiber permanently, which is potentially beneficial for climate change mitigation and the global environment.
Acknowledgements
This work is supported in part by the National Science Foundation Council under Grants 51479109 and 51479137.
Data availability statement
The authors confirm that the data supporting the findings of this study are available at 10.6084/m9.figshare.21501075.
Disclosure statement
No potential conflict of interest was reported by the authors.
References
- Sebos I. Fossil fraction of CO2 emissions of biofuels. Carbon Manag. 2022;13(1):154–163. doi:10.1080/17583004.2002.2046173.
- Luo X, Zhang R, Wang J. Product market competition and carbon disclosure: evidence from China. Carbon Manag. 2022;13(1):379–400. doi:10.1080/17583004.2002.2100830.
- Caldeira K, Wickett ME. Oceanography: anthropogenic carbon and ocean pH. Nature. 2003;425(6956):365–365. doi:10.1038/425365a.
- Jiao NZ. Developing ocean negative carbon emission technology to support national carbon neutralization. Bull Chin Acad Sci. 2021;36(2):179–187. (in Chinese)
- Dutkiewicz S, Morris JJ, Follows MJ, et al. Impact of ocean acidification on the structure of future phytoplankton communities. Nature Clim Change. 2015;5(11):1002–1006. doi:10.1038/NCLIMATE2722.
- Munday PL. New perspectives in ocean acidification research: editor’s introduction to the special feature on ocean acidification. Biol Lett. 2017;13(9):20170438. doi:10.1098/rsbl.2017.0438.
- Doney SC. The dangers of ocean acidification. Sci Am. 2006;294(3):58–65. doi:10.1038/scientificamerican0306-58.
- Lebrec M, Stefanski S, Gates R, et al. Ocean acidification impacts in select pacific basin coral reef ecosystems. Reg Stud Mar Sci. 2019;28:100584. doi:10.1016/j.rsma.2019.100584.
- Wu F, Lu W, Shang Y, et al. Combined effects of seawater acidification and high temperature on hemocyte parameters in the thick shell mussel Mytilus coruscus. Fish Shellfish Immunol. 2016;56:554–562. doi:10.1016/j.fsi.2016.08.012.
- Gazeau F, Parker LM, Comeau S, et al. Impacts of ocean acidification on marine shelled molluscs. Mar Biol. 2013;160(8):2207–2245. doi:10.1007/s00227-013-2219-3.
- Ross PM, Parker L, O’Connor WA, et al. The impact of ocean acidification on reproduction, early development and settlement of marine organisms. Water. 2011;3(4):1005–1030. doi:10.3390/w3041005.
- Kurihara H. Effects of CO2-driven ocean acidification on the early developmental stages of invertebrates. Mar Ecol Prog Ser. 2008;373:275–284. doi:10.3354/meps07802.
- Orr FM. Onshore geologic storage of CO2. Science. 2009;325(5948):1656–1658. doi:10.1126/science.1175677.
- White CM, Strazisar BR, Granite EJ, et al. Separation and capture of CO2 from large stationary sources and sequestration in geological formations-coalbeds and deep saline aquifers. J Air Waste Manag Assoc. 2003;53(6):645–715. doi:10.1080/10473289.2003.10466206.
- Sabine CL, Feely RA, Gruber N, et al. The oceanic sink for anthropogenic CO2. Science. 2004;305(5682):367–371. doi:10.1126/science.1097403.
- Jiao N, Herndl GJ, Hansell DA, et al. Microbial production of recalcitrant dissolved organic matter: long-term carbon storage in the global ocean. Nat Rev Microbiol. 2010;8(8):593–599. doi:10.1038/nrmicro2386.
- Shi T, Zheng XQ, Zhang H, et al. Coral reefs: potential blue carbon sinks for climate change mitigation. Bull Chin Acad Sci. 2021;36(3):270–278. (in Chinese)
- Olajire AA. A review of mineral carbonation technology in sequestration of CO2. J Pet Sci Eng. 2013;109:364–392. doi:10.1016/j.petrol.2013.03.013.
- Xie HP, Xie L, Wang YF, et al. CCU: a more feasible and economic strategy than CCS for reducing CO2 emissions. J Sichuan Univ. 2012;44(4):1–5. (in Chinese)
- Wang J, Zhao Y, Li J, et al. Research progress of carbon dioxide capture, fixation and utilization. Inorg Chem Ind. 2020;52(4):12–17. doi:10.11962/1006-4990.2019-0307.
- Marchetti C. On geoengineering and the CO2 problem. Clim Change. 1977;1(1):59–68. doi:10.1007/BF00162777.
- Orr JC, Fabry VJ, Aumont O, et al. Anthropogenic ocean acidification over the twenty-first century and its impact on calcifying organisms. Nature. 2005;437(7059):681–686. doi:10.1038/nature04095.
- Tibane LV, Pöllmann H, Ndongani FL, et al. Evaluation of the lithofacies, petrography, mineralogy, and geochemistry of the onshore Cretaceous Zululand Basin in South Africa for geological CO2 storage. Int J Greenhouse Gas Control. 2021;109:103364. doi:10.1016/j.ijggc.2021.103364.
- Schrag DP. Storage of carbon dioxide in offshore sediments. Science. 2009;325(5948):1658–1659. doi:10.1126/science.1175750.
- Connelly DP, Bull JM, Flohr A, et al. Assuring the integrity of offshore carbon dioxide storage. Renew Sustainable Energy Rev. 2022;166:112670. doi:10.1016/j.rser.2022.112670.
- Kinsey DW, Hopley D. The significance of coral reefs as global carbon sinks-response to greenhouse. Global Planet Change. 1991;3(4):363–377. doi:10.1016/0921-8181(91)90117-F.
- Yan HQ, Yu KF, Shi Q, et al. Air-sea CO2 fluxes and spatial distribution of seawater pCO2 in Yongle Atoll, Northern-Central South China Sea. Cont Shelf Res. 2018;165:71–77. doi:10.1016/j.csr.2018.06.008.
- Putnam HM, Barott KL, Ainsworth TD, et al. The vulnerability and resilience of reef-building corals. Curr Biol. 2017;27(11):R528–R540. doi:10.1016/j.cub.2017.04.047.
- Hughes TP, Kerry JT, Álvarez-Noriega M, et al. Global warming and recurrent mass bleaching of corals. Nature. 2017;543(7645):373–377. doi:10.1038/nature21707.
- Fabry VJ. Ocean science-marine calcifiers in a high-CO2 ocean. Science. 2008;320(5879):1020–1022. doi:10.1126/science.1157130.
- Wang W, Hu M, Zheng Y, et al. CO2 fixation in Ca2+-/Mg2+-rich aqueous solutions through enhanced carbonate precipitation. Ind Eng Chem Res. 2011;50(13):8333–8339. doi:10.1021/ie1025419.
- Jose-Luis GM, Ammar E, Jennie M, et al. Conceptual design of a CO2 capture and utilization process based on calcium and magnesium rich brines. J CO2 Util. 2018;27:161–169. doi:10.1016/j.jcou.2018.07.011.
- Zhao Y, Wu M, Yuan J, et al. Thorough conversion of CO2 through two-step accelerated mineral carbonation in the MgCl2-CaCl2-H2O system. Sep Purif Technol. 2019;210:343–354. doi:10.1016/j.seppur.2018.08.011.
- Zhao Y, Zhang Y, Liu J, et al. Trash to treasure: seawater pretreatment by CO2 mineral carbonation using brine pretreatment waste of soda ash plant as alkali source. Desalination. 2017;407:85–92. doi:10.1016/j.desal.2016.12.018.
- Hilbertz WH, Fletcher D, Krausse C. Mineral accretion technology: applications for architecture and aquaculture. Ind Forum. 1977;8(4-5):78–84.
- Hilbertz WH. Electrodeposition of minerals in sea-water: experiments and applications. IEEE J Ocean Eng. 1979; 4(3):94–113. doi:10.1109/JOE.1979.1145428.
- Goreau TJF. Marine electrolysis for building materials and environmental restoration. Electrolysis. 2012;13:273–290. doi:10.5772/48783.
- Goreau TJF, Prong P. Biorock electric reefs grow back severely eroded beaches in months. J Mar Sci Eng. 2017;5(4):48. doi:10.3390/jmse5040048.
- Zaslavschi I, Shemer H, Hasson D, et al. Electrochemical CaCO3 scale removal with a bipolar membrane system. J Membr Sci. 2013;445:88–95. doi:10.1016/j.memsci.2013.05.042.
- North M, Styring P. Perspectives and visions on CO2 capture and utilization. Faraday Discuss. 2015;183:489–502. doi:10.1039/c5fd90077h.