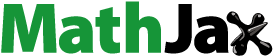
Abstract
Biodiesel, seen as a key alternative to fossil fuels, has drawn significant attention. In this study, we synthesised calcium oxide (CaO) nanoparticles and assessed their catalytic efficiency for biodiesel production from the nonedible seed oil of Argemone mexicana L. The nanoparticles were thoroughly characterised using scanning electron microscopy (SEM), X-ray diffraction (XRD), Fourier transform infrared (FT-IR), and thermogravimetric analysis (TGA) techniques. ASTM standard procedures were used to evaluate the fuel properties of biodiesel. Qualitative and quantitative analysis of biodiesel was conducted using gas chromatography –mass spectrometry (GC-MS), FT-IR, nuclear magnetic resonance (1H-NMR), and nuclear mangnetic resonance (13C-NMR). For biodiesel synthesis, the transesterification method was followed. The synthesised nanoparticles have a size of 28 nm, and the feedstock contains 43% oil content. Optimal biodiesel yield (96%) was achieved with a 1:9 oil to methanol ratio, 15 mg of catalyst, a temperature of 55 °C, and a reaction time of 50 min. Notably, the catalytic activity of CaO nanoparticles was diminished with repeated use. Most fuel properties were confirmed to the ASTM standard ranges. The results suggest the viability of the feedstock for large-scale biodiesel production.
Introduction
The two main components of sustainable economic growth are sustainability in the consistent supply of energy sources and energy security [Citation1]. Various energy sources are required to meet the daily energy demand of different industries, agriculture, and transportation [Citation2]. Conventional and nonrenewable energy sources are widely used to meet daily energy requirements and are considered expensive [Citation3]. Nonrenewable and conventional fossil fuels, such as petroleum, coal, and natural gas, are burned on a large scale, generating harmful gases [Citation4]. Due to the harmful effects of using these traditional fossil fuels to produce energy, researchers worldwide are focusing on the sustainable development of renewable and green energy sources [Citation4,Citation5]. Currently, most countries aim to achieve net zero carbon emissions by 2050–2070, but only 55% of countries worldwide have stated clear goals for enhanced reductions in carbon emissions by 2030 [Citation6].
To replace fossil fuels, biodiesel is considered the main alternative energy source. Biodiesel is a renewable feedstock composed of different fatty acids and methyl esters synthesised from algae, vegetable oil, and animal fats [Citation4]. Three different types of fatty acids (FAs) may be present in biodiesel: monounsaturated fatty acids (MUFAs), polyunsaturated fatty acids (PUFAs), and saturated fatty acids (SFAs). The melting temperature decreases with an increase in the number of double bonds; therefore, PUFAs have a lower melting point than SFAs. PUFAs are chemically more reactive than SFAs because chemical reactivity increases with the number of double bonds [Citation7].
Engine performance is one of the key characteristics of biodiesel as a green alternative to fossil fuel [Citation8]. Due to its concentrations of monoglycerides and FFAs, it has high lubricating properties. In addition to being used as a vehicle fuel and an energy source, biodiesel can be added to fossil fuels to increase their lubricity. Biodiesel is an excellent alternative to petro-diesel due to its higher lubricity, higher cetane number (>47), lower flash point (>130 °C), lower emissions of greenhouse gases and higher biodegradability [Citation9,Citation10].
Biodiesel is synthesised by transesterification of acylglycerols or FAs in the presence of primary alcohols over a suitable catalyst. The percentage of conversion of FAs to fatty acid methyl esters (FAMEs) is significantly influenced by the length of the alcohol chain and the amount of alcohol, since the reaction is reversible [Citation11]. Nonedible biomass or agricultural waste is economically advantageous for biofuel production [Citation12]. Moreover, biomass energy is advantageous because of its variety, abundance, and renewability. Generally, vegetation-derived biomass energy is widely known to be carbon neutral, as plants consume carbon dioxide for living and growth [Citation13]. The use of nonedible oils as feedstock for biodiesel is now the primary subject of attention worldwide. Due to their accessibility and low free fatty acid (FFA) content, the following nonedible oils are being examined for biodiesel production: Aleurites moluccana, Attalea speciosa, Azadirachta indica, Balanites aegyptiaca, Calophyllum inophyllum, Ceiba pentandra, Cerbera odollam, Croton megalocarpus, Euphorbia tirucalli, Gossypium herbaceum, Hevea brasiliensis, Jatropha curcas, Madhuca indica, Madhuca longifolia, Nicotiana tabacum, Pachira glabra, Pongamia pinnata, Sapium sebiferum, Simmondsia chinensis, Terminalia belerica and Zanthoxylum bungeanum [Citation14].
Currently, much work is being done to find economical catalysts to produce biodiesel in large quantities from nonedible seed oil (from widely available wild plants). In this paper, we have used the wild Mexican prickly poppy, or Argemone mexicana L., an annual herb of the family Papaveraceae [Citation15]. It is native to Mexico and has since spread naturally to subtropical and tropical areas of the world, including Africa, Australia, and Indo-Pakistan [Citation16]. The plant is a 20–125 cm tall herb with a prickly glabrous body. The leaves are alternate, elliptical-oblong, dentate, and prickly at the margin. The diameter of the flowers is from 3 to 8 cm, and each flower is sessile and surrounded by 2–3 foliaceous bracts. The sepals are imbricate, 5–7 mm wide and 8–12 mm long, with a sharp terete horn below the apex. The petals are 4–6 in number with an obovate shape and bright yellow colour. Each petal is 2–2.5 cm wide and 2.5–3.5 cm long. The aestivation of the petals is imbricate. Stamens are indefinite in number and 8–12 mm long. The ovary is ovate, 3–5 mm broad, and 8–10 mm long. The stigma is 3–6 lobed and red. The seeds are dark brown with a rounded shape [Citation17]. The seed oil content of Argemone mexicana seeds ranges from 30 to 50%, depending on the geography and growth environment [Citation16].
Transition metal oxides such as W [Citation18], Fe [Citation19], Ti [Citation20,Citation21], Cu [Citation22], Cd [Citation23], and Zn [Citation24] have acid-base bifunctional sites and are commonly used to catalyse the transesterification of ethanol–oil mixtures. However, group II metal oxides are more economical and, therefore, are preferred over transition metal oxides. Group II metal oxides such as MgO, CaO, BaO, and SrO are known for their basicity. Base-catalysed biodiesel production is initiated by the deprotonation of methanol by the base. Furthermore, nucleophilic attack of the methoxide ion over the triglyceride results in methyl ester formation. Group II activated carbon-supported metal oxide was utilised for biodiesel production from waste cooking oil in a 15:1 methanol to oil ratio at 65 °C for 90 min [Citation25]. The strength of the basic sites over the carbon support was found to occur in the following order: SrO > BaO > MgO > CaO-carbon. The low yield over MgO/C and BaO/C is due to their low number of basic sites and serious agglomeration, respectively. SrO has the highest number of basic sites, producing the highest biodiesel yield (94.27%). Lanthana-modified SrO (Sr/La = 8/1) acquired the highest concentration of weak basic sites and produced 97.25% methyl ester yield in 75 min at 65 °C from Prunus armeniaca seed oil (methanol/oil ratio; [M/O = 9]) [Citation26].
Although the over-supported carbon was minimal, the basicity of CaO was minimal, and its applicability as a catalyst needs to be capitalised on due to its cheapness, wide abundance, and non-toxic nature [Citation18]. CaO over alumina turned to inferior basicity and underwent serious agglomeration, which results in less activity [Citation27]. Taufiq-Yap et al. [Citation28] prepared CaO by precipitating calcium nitrate 4-hydrate with a mixture of NaOH and Na2CO3 at pH 11. The catalyst achieved a biodiesel yield of 60 °C in 90 min. However, CaO-La2O3 (Ca/La = 4) achieved the highest amount of basicity (including strong basic sites), resulting in the highest biodiesel yield (86.51%) at 65 °C from Jatropha curcas oil (M/O = 24) [Citation28]. CaO-CeO2 added bifunctional sites, a larger surface area, pore volume, and pore diameter in catalyst architects, and a ∼90% biodiesel yield <90% at 70 °C for 90 min from loquat seed oil (M/O = 9) [Citation12]. Yan et al. dispersed 30 wt% CaO-CeO2 on hydroxyapatite and achieved higher (92%) biodiesel yield at 65 °C in 3 h [Citation29]. More work with CaO-based catalysts is still needed to achieve more than 95% biodiesel yield.
Therefore, this study was conducted to synthesise CaO nanoparticles and to study the physico-chemical properties of the nanoparticles. Biodiesel was synthesised from the seed oil of Argemone mexicana using CaO nanoparticles as a catalyst. We recycled the CaO nanoparticles and studied the catalytic activity of recycled nanoparticles. In addition, qualitative and quantitative analyses of different types of FAMEs present in the biodiesel are carried out. The fuel properties of the biodiesel produced are compared with American society for testing and materials (ASTM) standards.
Materials and methods
CaO nanoparticle preparation
Calcium oxide (CaO) nanoparticle synthesis was carried out using the sol-gel method of Tahvildari et al. [Citation29]. As a precursor, calcium nitrate tetrahydrate (Merck, 99% purity) was added to distilled water and continuously stirred until completely dissolved, and then 25 mL of ethylene glycol (Merck, 99% purity) was added dropwise. After this, NaOH solution was also added dropwise and stirred for 2 h until a gel-like substance was formed. It was then filtered through Whatman filter paper (grade 3). The filtered gel was then kept in an oven at 105 °C for 2 h for dehydration. The gel was also cleaned with distilled water 4–5 times to reduce the alkalinity. It was dried in an oven for 4 h at 105 °C after being washed with distilled water. The dry gel was ground to a fine powder with the help of a pestle and mortar and then kept in the furnace for 1 h at 850 °C for calcination. The synthesised CaO nanoparticles were stored in a desiccator for future use [Citation20].
Characterisation of CaO nanoparticles
X-ray diffraction (XRD) is a fast analytical method usually used to determine the crystalline phase of materials [Citation30–33]. Through XRD, monochromatic crystalline X-ray interference is investigated [Citation33]. A Shimadzu-6000 model was used for XRD spectroscopy. The diffractometer had a Cuk-α source (K = 1.54 A°) that kept the applied voltage at 40 kV and the current at 30 mA. Data were obtained at 2θ with a range of 10–90°. The sizes of the nanoparticles were calculated using the Scherrer equation [Citation34]:
(1)
(1)
where β is the full width of half the maximum intensity (FWHM) in radians, λ is the wavelength of radiation (1.54 Å), and k is the shape factor (= 0.9).
Scanning electron microscopy (SEM) was performed using a JEOL JSM-5910 model microscope and an HT7800 Ruli model microscope. The scanned images were captured using the field emissions from the SEM microscope under a 20 kV accelerating voltage to evaluate the catalyst surface qualitatively and explain the events that occurred during pretreatment and calcination. The particle size was determined using the dynamic light scattering (DLS) method with the use of a Malvern Zetasizer Nano ZS96 Analyser. Samples were diluted in double-deionised water. The analysis was carried out at 25 °C in a disposable cuvette. For 60 s, the instrument count rate was set at 291.4 kcps.
The infrared spectra for the synthesised CaO nanoparticles were obtained using a VARIAN Model-AA280Z fourier transform infrared (FT-IR) spectrometer. The spectrometer was equipped with a GTA-120 graphite tube atomiser. Each spectrum was collected utilising four scans at a resolution of 4 cm−1 in a short period. The spectrometry was done in the 400–4000 cm−1 range. To process all the results, spectrum computer software was used. The Perkin Elmer Diamond apparatus was used with an airflow of 60 mL/min and a heating rate of 10 °C/min to obtain the results of thermogravimetric analysis (TGA) and derivative thermogravimetry (DTG).
Catalyst recovery
Calcium oxide nanoparticles were recycled by centrifugation, cleaned with distilled water and ethyl acetate 4 times, dried at 80 °C in the oven for 12 h, and then calcined at 800 °C. Recycled CaO nanoparticles were reused 5 times [Citation35,Citation36].
Evaluation of the fuel properties
Following ASTM standards, the fuel properties of the synthesised biodiesel were evaluated () [Citation34].
Table 1. Fuel properties of Argemone mexicana biodiesel (AMB), and American society for testing and materials (ASTM) standard methods adopted.
Analysing the physicochemical characteristics of biodiesel
The synthesis of Argemone mexicana biodiesel (AMB) was confirmed, and its physicochemical characteristics examined, by FT-IR, gas chromatography mass spectrometry (GC-MS), and nuclear mangnetic resonance (NMR) spectroscopic investigations. 13C and 1H NMR spectrometry on 11.75 T was performed at 21 °C using an Avance NEO Bunker 600 MHz spectrometer. The spectrometer was equipped with a broad band frequency (BBF) smart probe of 5 mm. The internal standard solvents for authentication were Si(CH3)4 and chloroform d. To measure the spectrum for 1H NMR (300 MHz), 1.0 scans, 8 scans of recycle time and a pulse length of 30 degrees were used. The spectrum of the 13C NMR (75 MHz) was thus measured at 1.89 with a scan-recycle delay of 160 and 300 pulse duration. Conversion of triglycerides to related FAMEs was expressed as a percentage of the residual solvent peak.
The various functional groups present in the biodiesel sample were identified using FT-IR spectroscopy. The different stretching and bending vibrations confirm the presence of various functional groups [Citation17]. Infrared spectra for the synthesised biodiesel were obtained using a VARIAN Model-AA280Z FT-IR spectrometer. The spectrometer was equipped with a GTA-120 graphite tube atomiser. Each spectrum was collected using four scans at a resolution of 4 cm−1 in a short period. The spectrometry was done in the 400–4000 cm−1 range. To process all of the findings, spectrum computer software was used.
To identify various FAMEs in the biodiesel sample, a Shimadzu Japan-made QP-2010 Plus GC-MS spectrometer was used. Biodiesel (0.1 µL) was put on the spectrometer. C6H14 was used as a solvent, and vector gas helium was used at a rate of 1.44 mL/min. The injector and detector temperature was set at 250 °C, and the column temperature was set at 50 to 300 °C with a flow rate of 80 °C/min. The mass spectrograph was programmed to scan between 50 and 1000 m/z using electron impact ionisation [Citation37].
Results and discussion
Characterisation of CaO nanoparticles
The synthesised nanoparticles were spherical and closely packed. Furthermore, the nanoparticles have variable diameters with a rough and porous surface (). As a result of nodule formation, CaO-CaO nanoparticles are bonded to one another [Citation38,Citation39]. The uniform and organised structure of particles indicates a better interconnected regular pore distribution [Citation36].
Figure 1. Scanning electron micrographs of synthesised nanoparticles: (a) 0.5 µm × 5000; (b) 0.5 µm × 10,000; (c) 1 µm × 5000; (d) 1 µm × 10,000.
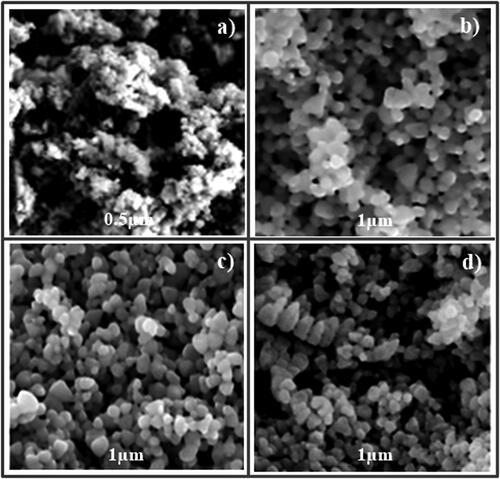
Strong deflection peaks at 2 theta angles of 67.61°, 64.42°, 53.85°, 37.49° and 32.51° were observed in the XRD spectrum of CaO nanoparticles, which correspond to (111), (200), (220), (311) and (222), respectively () (JCDPS No.77-2376) [Citation37]. The crystalline size of the nanoparticles was 28 nm. , obtained from the TGA of synthesised CaO nanoparticles, shows that initially, the nanoparticles lost weight at 370–410 °C. The weight loss indicates the decomposition of Ca(OH)2 formed due to the reaction of nanoparticles with moisture in the air. The breakdown of residual CaCO3 causes a slight weight loss between 580 and 700 °C [Citation40–43]. In the differential scanning calorimetry (DSC) curve, an endothermic peak indicates both decompositions. The infrared spectrum shows a prominent stretching peak at 1413 cm−1, confirming the presence of Ca-O (). The peak at 2924 cm−1 is for CaCO3. It confirms that during the calcination process, CaCO3 was decomposed into CaO and CO2 [Citation40]. The peak at 3432 cm−1 is for O-H stretching vibrations, confirming the presence of a small amount of water molecules. This was due to the physisorbed water molecule on the surface of CaO [Citation36,Citation40]. Almost identical findings have been reported in the literature [Citation35,Citation39].
Oil and FFA content determination
Two methods were used to extract the raw oil: chemical extraction with a Soxhlet apparatus and mechanical extraction. Extracting raw oil using a Soxhlet is recommended by the European Union. It is typically used to determine the oil content of the feedstock but is costly and requires expertise to use [Citation44]. The Soxhlet oil extraction method confirmed a 43% oil content in the feedstock. The FFA content was determined by the acid-base titration technique. The current feedstock has an FFA value of 0.49 mg KOH/g, which is lower than the required level established by ASTM D-6751 [Citation45].
Biodiesel synthesis
For the synthesis of biodiesel, the transesterification technique was adopted. When a suitable type of catalyst is present, the transesterification process proceeds effectively. In this study, we used CaO nanoparticles as a catalyst. A parametric study of the following parameters was conducted to obtain the optimum yield of biodiesel.
Oil to methanol ratio
The molar ratio of oil to methanol is the most important factor influencing the chemical and technical processes for biodiesel synthesis. The stoichiometric calculation for the oil-to-methanol ratio suggests using 3 moles of methanol for 1 mole of oil to produce 3 moles of biodiesel. However, due to the reversible nature of the transesterification process, this ratio is not sufficient to complete the reaction. Therefore, a higher ratio of oil to methanol is required to complete the reaction [Citation46]. Thus, a series of reactions with varying oil-to-methanol ratios (1:3, 1:6, 1:9, 1:12 and 1:15) were carried out to determine the ideal ratio for maximum yield. It is clear from the results that the optimum yield of 96% was obtained at a 1:9 ratio of oil to methanol. According to the literature, the reversible nature of the transesterification process requires using a significant quantity of methanol to break the bonds between fatty acids and glycerin [Citation47]. However, the results of this study show that the yield decreased with higher ratios (). The dilution of the reaction mixture and disruption of the active catalyst site, resulting in less contact between the catalyst and the oil and methanol, can cause a drop in biodiesel yield [Citation48,Citation49]. The high methanol content also hinders the optimisation of biodiesel yield and complicates and increases the cost of the ester recovery process [Citation34]. Furthermore, the separation of esters from glycerol is slowed by oil-to-methanol ratios higher than 1:70 [Citation50].
Catalyst dosage
When an appropriate quantity of catalyst is present, the transesterification reaction proceeds well [Citation48]. According to the literature, the right amount of catalyst increases the yield and reduces cost [Citation51]. We conducted a series of reactions with catalyst doses of 5, 10, 15, 20, and 25 mg to identify the exact quantity of catalyst needed for optimum biodiesel yield. The maximum yield (96%) was achieved at a catalyst dosage of 15 mg (). As the catalyst concentration increases, more active sites become accessible for converting reactants into products, increasing biodiesel yield and reducing reaction time [Citation51]. Furthermore, a sufficient catalyst concentration lowers the cost of the product by using less energy [Citation52]. However, a higher catalyst dose showed a slight decrease in biodiesel yield.
Reaction temperature
The reaction temperature has a noticeable effect on the biodiesel yield, as well as the duration of the reaction, since a high temperature reduces the viscosity of the solution, increases the solubility of the reactants, and accelerates the rate at which reactants are transformed into the final product. To conserve energy, time, and money, a minimum temperature for transesterification is also an important factor in the industrial production of biodiesel [Citation53]. This means that the yield of biodiesel is directly influenced by temperature. Thus, we carried out a series of reactions, keeping the temperature at 45, 50, 55, 60, and 65 °C to determine the lowest temperature at which the highest biodiesel yield may be obtained. The study shows that the biodiesel yield increased to 96% at 55 °C but decreased at higher temperatures (). The evaporation of methanol from the reaction mixture can cause a drop in biodiesel yield at higher temperatures [Citation53,Citation54]. Other studies have reported similar results [Citation44,Citation45].
Reaction time
On an industrial basis, reaction time is important in determining biodiesel production. Longer reaction times should be avoided, as they raise production costs due to greater energy use [Citation55,Citation56]. We kept the reaction duration at 25, 50, 75, 100, and 125 min to determine the lowest ideal reaction time for the highest biodiesel yield. Our findings show that at 50 min of reaction time, the highest yield (96%) was achieved (). A slight decline was seen when the reaction time was extended to more than 50 min. The reverse reaction between the glycerol produced and the biodiesel may cause a decrease in biodiesel yield [Citation57]. Similar findings have also been observed in other studies [Citation44,Citation57].
CaO catalyst reusability
In biodiesel production, the reusability and stability of calcium-based catalysts is one of the major concerns for economic feasibility [Citation36]. The CaO nanoparticles were recovered and reused to study the catalyst activity and stability. The catalyst amount, oil-to-methanol ratio, reaction temperature, and reaction time were kept the same as for the optimum yield. The biodiesel yield decreased in successive cycles (). According to the SEM picture shown in , the surface morphology of CaO changed with repeated use, leading to a drop in biodiesel yield. The agglomeration of other particles (glycerin) with the catalyst and the mixing of the catalyst with the reactants were the causes of the change in the surface morphology. The following factors may be responsible for the lower catalytic activity: (i) blocking of the active site of the catalyst by the reactants; and (ii) partial solubility of CaO nanoparticles in the reaction mixture [Citation36].
Fuel properties of biodiesel
Using standard techniques of the American Society for Testing Materials (ASTM), the fuel properties of biodiesel were assessed and compared to the standards set by ASTM D-6751. Most of the fuel properties were within the ASTM D-6751 standards (). The ASTM has a specified cetane number of 45 for the biodiesel, while the AMB has a cetane number of 48 (). However, adding a small amount of nitric acid iso-octyl can reduce the cetane number [Citation58]. The refractive index of biodiesel is 1.453. The refractive index demonstrates that the conversion of crude oil to biodiesel was successful [Citation59]. The oxidative stability of AMB is 1.78 h. The oxidative stability demonstrates the relative sensitivity of fuel to oxidative degradation [Citation60]. Thus, the susceptibility to oxidation of biodiesel is advantageous for the environment [Citation61]; however, it is certainly a major problem as well as an obstacle in the industrialisation of biodiesel. The physicochemical and tri-biological properties of biodiesel are altered by oxidation during storage or use. The effects of this phenomenon are immediately felt by the characteristics of biodiesel, including its acid number, density, iodine value, kinematic viscosity, polymer content, and peroxide value. However, adding different antioxidants can reduce biodiesel sensitivity to oxidation [Citation62].
According to our analysis, AMB has a water content of 0.017. A critical component of fuel quality is its water content. Biodiesel has a higher potential to absorb moisture than conventional fuels since it is more hygroscopic. High moisture levels in biodiesel encourage the growth of algae in gasoline tanks, which corrodes fuel tanks and produces slime and sludge that clog engine filters and fuel pipes, damaging the fuel injection system [Citation63].
The iodine value for AMB was determined to be 113.7 mg I/100. The iodine value of biodiesel also demonstrates the level of oxidative stability, saturation, and unsaturation of biodiesel [Citation64]. The higher heating value (HHV) for AMB is 40.07 MJ/kg, which is within the acceptable limit given by ASTM D-6751. HHV is a crucial aspect of biodiesel which, like other qualities of fuels, reveals details about the energy content of the fuel as well as its efficiency [Citation65]. The fatty acid composition, iodine concentration, and saponification value are used to calculate it [Citation44]. Biodiesel has a lower HHV (39 to 43 MJ/kg) than fossil fuels (49.65 MJ/kg) [Citation58].
1H-NMR spectroscopy
The singlet peak of the methoxy proton (-OCH3) was observed at 3.479 ppm. It confirms the conversion of raw oil to biodiesel. Peaks for alpha-methylene proton (α-CH2) were observed between 2.027 and 2.069 ppm. These two peaks demonstrate that FAMEs were produced from triglycerides. The terminal methyl proton (CH3) peaks were obtained at 0.856–0.947 ppm. Peaks obtained at 1.281 to 1.641 ppm confirm beta-carbonyl methylene protons. Olefinic hydrogen indicator peaks reached 5.174–5.430 ppm (). These are the confirmatory peaks for the effective conversion of triglycerides to biodiesel [Citation66].
13 C-NMR spectroscopy
Long-chain ethylene carbons (-CH2) were detected at 24.95–34.21 ppm. Carbonyl carbon (-CO) peaks were obtained at 174.05 and 174.07 ppm. Peaks for olefinic carbons were recorded at 129.63 and 130.10 ppm. Furthermore, at 127.07 and 128.17 ppm, the peaks for the vinylic substituent were obtained. The existence of -O-CH3 was confirmed from the peak obtained at 51.19 ppm (). Other researchers reported similar findings in their studies [Citation67].
GC-MS/EI analysis of biodiesel sample
The composition and quantity of the FAMEs in the synthesised biodiesel sample were determined through gas chromatography mass spectrometry/electron ionization (GC-MS/EI). The National Institute of standards and Technology (NIST-02) library match software was used for the identification of FAMEs. The chromatogram shows that the sample has 10 different types of FAMEs (; ). The results indicate that the biodiesel sample is mostly composed of linoleic acid methyl ester, followed by oleic acid methyl ester and lenolenic acid methyl ester (). The high concentration of unsaturated FAMEs in the biodiesel sample indicates that the feedstock is suitable for biodiesel production [Citation68].
Table 2. Reported FAMEs, retention time, and percentage.
Fourier transform infrared analysis of synthesised biodiesel
FT-IR is the most effective and most commonly used analytical method for identifying the macromolecular pool (lipids, proteins, and carbohydrates) [Citation69]. Therefore, FT-IR spectroscopy was used to identify the different functional groups, their associated bonds and the bending and stretching vibrations in the synthesised biodiesel. It has been demonstrated that FAMEs can absorb electromagnetic energy from infrared wavelengths [Citation70]. The different peaks identified in the FT-IR spectrum of biodiesel for various functional groups are given in . explains the FT-IR spectrum of the synthesised biodiesel. According to the the literature, the carbonyl group depends on both the substituent effects and the molecular structure [Citation45]. Two major absorption peaks, one for carbonyl with a band gap of 1725–1750 cm−1 and the other for OC with a band gap of 1000–1300 cm−1, validate the synthesis of esters [Citation58]. Furthermore, the peak obtained between 1230 and 1270 cm−1 confirms the presence of aromatic ether and the synthesis of biodiesel [Citation71]. Transesterification was completed, indicated by the FT-IR spectroscopic absorption bands [Citation72,Citation73].
Table 3. Various functional groups and their peaks identified in the FT-IR spectrum of biodiesel.
Conclusion
One of the presently available sources of clean, renewable energy that could meet future energy needs is biodiesel. In the present work, we synthesised biodiesel using nonedible seed oil of Argemone mexicana. A transesterification procedure was used to create the biodiesel. CaO nanoparticles were first synthesised in the laboratory. The DLS technique was used to determine the size (28 nm). Through the use of FT-IR, SEM, and XRD, the synthesis of nanoparticles was confirmed as well, and their surface morphology and physical properties were studied. Using a 1:9 oil-to-methanol ratio and 15 mg of CaO as a catalyst, and keeping the reaction temperature at 55 °C and the reaction time at 50 min, a maximum biodiesel yield of 96% was achieved. Fuel properties were examined using ASTM methods, and the results were compared with the accepted ranges defined by the ASTM D standard. The synthesis of biodiesel, its composition and the structure of its chemical compounds were confirmed using multiple spectroscopic methods (FT-IR; GC-MS; and 1H and 13C NMR). The feedstock is a competitive source for producing biodiesel on a commercial scale, and the physicochemical characteristics of the synthesised biodiesel demonstrate that it is an environmentally friendly fuel.
Author contributions
Conceptualisation, HAJ; methodology, HAJ, IS, ASA, and AIO; validation, IS, ASA, and AIO; formal analysis, HAJ, and IS; investigation, HAJ, and IS; data curation, HAJ writing – original draft preparation, HAJ; writing – review and editing, HAJ, IS, ASA, RK, and AIO; funding acquisition, ASA and IS. All authors have read and agreed to the published version of the manuscript.
Disclaimer
The views and opinions expressed in this paper do not necessarily reflect those of the European Commission or the Special EU Programs Body (SEUPB).
Acknowledgements
The authors would like to extend their sincere appreciation to the Researchers Supporting Project number (RSP2023R368), King Saud University, Riyadh, Saudi Arabia. The authors acknowledge Aleš Ház for technical support. RK acknowledges Indus University, Ahmedabad, for supporting the research. The authors acknowledge the support of The Bryden Centre project (Project ID VA5048). The Bryden Centre project is supported by the European Union’s INTERREG VA Programme, managed by the Special EU Programmes Body (SEUPB).
Disclosure statement
No potential conflict of interest was reported by the author(s).
Data availability statement
All the data are given in the article.
Additional information
Funding
References
- Gai L, Varbanov PS, Fan YV, et al. Trade-offs between the recovery, exergy demand and economy in the recycling of multiple resources. Resour Conserv Recycl. 2021;167:105428. 105428, ISSN 0921-3449, doi: 10.1016/j.resconrec.2021.105428.
- Moayedi H, Aghel B, Abdullahi MM, et al. Applications of rice husk ash as green and sustainable biomass. J Cleaner Prod. 2019;237:117851. volume ISSN 0959-6526, doi: 10.1016/j.jclepro.2019.117851.
- Amen R, Hameed J, Albashar G, et al. Modelling the higher heating value of municipal solid waste for assessment of waste-to-energy potential: a sustainable case study. J Cleaner Prod. 2021;287:125575. doi: 10.1016/j.jclepro.2020.125575.
- Qadeer MU, Muhammad Ayoub M, Komiyama M, et al. Review of biodiesel synthesis technologies, current trends, yield influencing factors and economical analysis of supercritical process. J Cleaner Prod. 2021;309:127388. doi: 10.1016/j.jclepro.2021.127388.
- Lim XY, Yek PNY, Liew RK, et al. Engineered biochar produced through microwave pyrolysis as a fuel additive in biodiesel combustion. Fuel. 2022;312:122839. doi: 10.1016/j.fuel.2021.122839.
- Yang M, Chen L, Wang J, et al. Circular economy strategies for combating climate change and other environmental issues. Environ Chem Lett. 2023;21(1):55–80. doi: 10.1007/s10311-022-01499-6.
- Sharma V, Hossain AK, Duraisamy G. Experimental investigation of neat biodiesels’ saturation level on combustion and emission characteristics in a CI engine. Energ. 2021;14(16):5203. doi: 10.3390/en14165203.
- Bencheikh K, Atabani AE, Shobana S, et al. Fuels properties, characterizations and engine and emission performance analyses of ternary waste cooking oil biodiesel–diesel–propanol blends. Sus Ener Tech Assess. 2019;35:321–334. doi: 10.1016/j.seta.2019.08.007.
- Boulal A, Atabani AE, Mohammed MN, et al. Integrated valorization of Moringa Oleifera and waste phoenix Dactylifera L. dates as potential feedstocks for biofuels production from Algerian Sahara: an experimental perspective. Biocat Agricul Biotech. 2019;20:101234. doi: 10.1016/j.bcab.2019.101234.
- Mares EKL, Goncalves MA, da Luz PTS, et al. Acai seed ash as a novel basic heterogeneous catalyst for biodiesel synthesis: optimization of the biodiesel production process. Fuel. 2021;299:120887. doi: 10.1016/j.fuel.2021.120887.
- da Conceição LR, da Costa CE, Rocha Filho GND, et al. Ethanolysis optimization of jupati (raphia taedigera mart.) oil to biodiesel using response surface methodology. J Brazil Chem Socie. 2015;26:1321–1330.
- Al-Muhtaseb AH, Osman AI, Murphin Kumar PS, et al. Circular economy approach of enhanced bifunctional catalytic system of CaO/CeO2 for biodiesel production from waste loquat seed oil with life cycle assessment study. Energy Conv Manag. 2021;236:114040. doi: 10.1016/j.enconman.2021.114040.
- Osman AI, Lai ZY, Farghali M, et al. Optimizing biomass pathways to bioenergy and biochar application in electricity generation, biodiesel production, and biohydrogen production. Environ Chem Lett. 2023;21(5):2639–2705. doi: 10.1007/s10311-023-01613-2.
- Arya I, Poona A, Dikshit PK, et al. Current trends and future prospects of nanotechnology in biofuel production. Catalysts. 2021;11(11):1308. doi: 10.3390/catal11111308.
- Brahmachari G, Gorai D, Roy R. Argemone mexicana: chemical and pharmacological aspects. Revista Brasil. de Farma. 2013;23(3):559–575. doi: 10.1590/S0102-695X2013005000021.
- Ashine F, Balakrishnan S, Kiflie Z, et al. Parametric optimization of Argemone mexicana seed oil extraction by Box-Behnken experimental design and the oil characteristics. Results in Chem. 2022;4:100570. doi: 10.1016/j.rechem.2022.100570.
- https://www.worldfloraonline.org/taxon/wfo-0000547034
- Abbasi TU, Ahmad M, Asma M, et al. High efficient conversion of Cannabis sativa L. biomass into bioenergy by using green tungsten oxide nano-catalyst towards carbon neutrality. Fuel, 2023;336:126796. doi: 10.1016/j.fuel.2022.126796.
- Al-Mawali KS, Osman AI, Ala’a H, et al. Life cycle assessment of biodiesel production utilizing waste date seed oil and a novel magnetic catalyst: a circular bioeconomy approach. Renew Energy. 2021;170:832–846. doi: 10.1016/j.renene.2021.02.027.
- Jan HA, Osman AI, Al-Fatesh AS, et al. Biodiesel production from Sisymbrium irio as a potential novel biomass waste feedstock using homemade titania catalyst. Sci Rep. 2023;13(1):11282. doi: 10.1038/s41598-023-38408-y.
- Qamar OA, Jamil F, Hussain M, et al. Advances in synthesis of TiO2 nanoparticles and their application to biodiesel production: a review. Chem. Engin. J. 2023;460:141734. doi: 10.1016/j.cej.2023.141734.
- Chia SR, Ahmad M, Sultana S, et al. Green synthesis of biodiesel from Citrus medica seed oil using green nanoparticles of copper oxide. Fuel, 2022;323:124285. doi: 10.1016/j.fuel.2022.124285.
- Aziz A, Ahmad M, Zafar M, et al. Novel copper oxide phyto-nanocatalyst utilized for the synthesis of sustainable biodiesel from citrullus colocynthis seed oil. Processes. 2023;11(6):1857. doi: 10.3390/pr11061857.
- Arshad S, Ahmad M, Munir M, et al. Assessing the potential of green CdO2 nano-catalyst for the synthesis of biodiesel using non-edible seed oil of Malabar Ebony. Fuel, 2023;333:126492. doi: 10.1016/j.fuel.2022.126492.
- Jamil F, Kumar PSM, Al-Haj L, et al. Heterogeneous carbon-based catalyst modified by alkaline earth metal oxides for biodiesel production: parametric and kinetic study. Energy Conv. and Manag. X. 2021;10:100047.
- Ala’a H, Osman AI, Jamil F, et al. Integrating life cycle assessment and characterization techniques: a case study of biodiesel production utilizing waste Prunus Armeniaca seeds (PAS) and a novel catalyst. J Environ Manag. 2022;304:114319.
- Marinković DM, Avramović JM, Stanković MV, et al. Synthesis and characterization of spherically-shaped CaO/γ-Al2O3 catalyst and its application in biodiesel production. Energy Conv Manag. 2017;144:399–413. doi: 10.1016/j.enconman.2017.04.079.
- Taufiq-Yap YH, Teo SH, Rashid U, et al. Transesterification of Jatropha curcas crude oil to biodiesel on calcium lanthanum mixed oxide catalyst: effect of stoichiometric composition. Energy Conv Manag. 2014;88:1290–1296. doi: 10.1016/j.enconman.2013.12.075.
- Yan B, Zhang Y, Chen G, et al. The utilization of hydroxyapatite-supported CaO-CeO2 catalyst for biodiesel production. Energy Conv Manag. 2016;130:156–164. doi: 10.1016/j.enconman.2016.10.052.
- Tahvildari K, Anaraki YN, Fazaeli R, et al. The study of CaO and MgO heterogenic nano-catalyst coupling on transesterification reaction efficacy in the production of biodiesel from recycled cooking oil. J Environ Health Sci Eng. 2015;13(1):1–9.
- Bharti P, Singh B, Dey RK. Process optimisation of biodiesel production catalyzed by the CaO nanocatalyst using response surface methodology. J Nanostruct Chem. 2019;9(4):269–280. doi: 10.1007/s40097-019-00317-w.
- Babar M, Mubashir M, Mukhtar A, et al. Sustainable functionalised metal-organic framework NH2-MIL-101 (Al) for CO2 separation under cryogenic conditions. Environ Pollut. 2021;279:116924. doi: 10.1016/j.envpol.2021.116924.
- Ozor PA, Aigbodion VS, Sukdeo NI. Modified calcium oxide nanoparticles derived from oyster shells for biodiesel production from waste cooking oil. Fuel Commun. 2023;14:100085. doi: 10.1016/j.jfueco.2023.100085.
- Dawood S, Koyande AK, Ahmad M, et al. Synthesis of biodiesel from non-edible oil (brachychiton populneus) in the presence of nickel oxide nanocatalyst: parametric and optimisation studies. Chemosph. 2021;278:130469. doi: 10.1016/j.chemosphere.2021.130469.
- Safaei-Ghomi J, Ghasemzadeh MA, Mehrabi M. Calcium oxide nanoparticles catalysed one-step multicomponent synthesis of highly substituted pyridines in aqueous ethanol medium. Scientia Iranica. 2013;20(3):549–554.
- Pandit PR, Fulekar MH. Biodiesel production from microalgal biomass using CaO catalyst synthesised from natural waste material. Renew. Energy. 2019;136:837–845. doi: 10.1016/j.renene.2019.01.047.
- Flemmer AC, Franchini MC, Lindström LI. Description of safflower (Carthamus tinctorius) phenological growth stages according to the extended BBCH scale. Ann Appl Biol. 2015;166(2):331–339. doi: 10.1111/aab.12186.
- Fan M, Liu Y, Zhang P, et al. Blocky shapes Ca-Mg mixed oxides as a water-resistant catalyst for effective synthesis of biodiesel by transesterification. Fuel. Pro. Tech. 2016;149:163–168. doi: 10.1016/j.fuproc.2016.03.029.
- Davoodbasha M, Pugazhendhi A, Kim JW, et al. Biodiesel production through transesterification of Chlorella vulgaris: synthesis and characterisation of CaO nanocatalyst. Fuel. 2021;300:121018. doi: 10.1016/j.fuel.2021.121018.
- Margaretha YY, Prastyo HS, Ayucitra A, et al. Calcium oxide from Pomacea sp. shell as a catalyst for biodiesel production. Inter J Energy Environ Engin. 2012;3:1–9.
- Sharma YC, Singh B, Korstad J. Application of an efficient nonconventional heterogeneous catalyst for biodiesel synthesis from pongamia pinnata oil. Energy Fuels. 2010;24(5):3223–3231. doi: 10.1021/ef901514a.
- Al-Sakkari EG, El-Sheltawy ST, Attia NK, et al. Kinetic study of soybean oil methanolysis using cement kiln dust as a heterogeneous catalyst for biodiesel production. App Catal B. 2017;206:146–157. doi: 10.1016/j.apcatb.2017.01.008.
- Ranjbar M, Pardakhty A, Tahmipour B, et al. Novel CaO/polylactic acid nanoscaffold as dental resin nanocomposites and the investigation of physicochemical properties. Luminescence. 2019;34(3):360–367. doi: 10.1002/bio.3617.
- Jan HA, Saqib NU, Aamir A, et al. Aleurites moluccana as a potential nonedible feedstock for industrial-scale biodiesel synthesis using homemade zinc oxide nanoparticles as a catalyst. WBV. 2023;2023:1–15. doi: 10.1007/s12649-023-02238-w.
- Jan HA, Saqib NU, Khusro A, et al. Synthesis of biodiesel from carthamus tinctorius L. oil using TiO2 nanoparticles as a catalyst. J. King Saud Uni. Sci. 2022;34(8):102317. doi: 10.1016/j.jksus.2022.102317.
- Jan HA, Šurina I, Zaman A, et al. Synthesis of biodiesel from Ricinus communis L. Seed oil, a promising Non-Edible feedstock using calcium oxide nanoparticles as a catalyst. Energies. 2022;15(17):6425. doi: 10.3390/en15176425.
- Kumar K. Standardization of nonedible Pongamia pinnata oil methyl ester conversion using hydroxyl content and GC–MS analysis. J Taiw Ins Chem Eng. 2013;45(4):1485–1489. doi: 10.1016/j.jtice.2013.11.002.
- Talukdar A, Deka DC. Preparation and characterisation of a heterogeneous catalyst from water hyacinth (Eichhornia crassipes): catalytic application in the synthesis of bis (indolyl) methanes and bis (pyrrolyl) methanes under solvent-free conditions. Current Catal. 2016;5(1):51–65. doi: 10.2174/2211544705666160310234657.
- Nath B, Das B, Kalita P, et al. Waste to value addition: utilisation of a novel green heterogeneous base catalyst derived from Brassica nigra waste for effective synthesis of biodiesel. J. Cleaner Produc. 2019;239:118112. doi: 10.1016/j.jclepro.2019.118112.
- Miao X, Wu Q. Biodiesel production from heterotrophic microalgal oil. Bioresour Technol. 2006;97(6):841–846. doi: 10.1016/j.biortech.2005.04.008.
- Srilatha K, Lingaiah N, Devi BP, et al. Esterification of free fatty acids for biodiesel production over heteropoly tungstate supported on niobia catalysts. App. Catalysis A. 2009;365(1):28–33. doi: 10.1016/j.apcata.2009.05.025.
- Leung DYC, Guo Y. Transesterification of neat and used frying oil: optimization for biodiesel production. Fuel Process Tech. 2006;87(10):883–890. doi: 10.1016/j.fuproc.2006.06.003.
- Dhawane SH, Karmakar B, Ghosh S, et al. Parametric optimization of biodiesel synthesis from waste cooking oil via Taguchi approach. J Environ Chem Engin. 2018;6(4):3971–3980. doi: 10.1016/j.jece.2018.05.053.
- Niju S, Begum KMS, Anantharaman N. Enhancement of biodiesel synthesis over highly active CaO derived from natural white bivalve clam shell. Arab J Chem. 2016;9(5):633–639. doi: 10.1016/j.arabjc.2014.06.006.
- Barros SDS, Junior WAP, Sá IS, et al. Pineapple (Ananás comosus) leaves ash as a solid base catalyst for biodiesel synthesis. Bioresour Technol. 2020;312:123569. doi: 10.1016/j.biortech.2020.123569.
- Gebremariam SN, Marchetti JM. Economics of biodiesel production. Ener Conver Manag. 2018;168:74–84. doi: 10.1016/j.enconman.2018.05.002.
- Laskar IB, Rokhum L, Gupta R, et al. Zinc oxide supported silver nanoparticles as a heterogeneous catalyst for the production of biodiesel from palm oil. Environ Prog Sust Energy. 2020;39(3):e13369.
- Ullah K, Jan HA, Ahmad M, et al. Synthesis and structural characterisation of biofuel from Cocklebur sp. biofuel, using zinc oxide nanoparticles: a novel energy crop for the bioenergy industry. Front Bioeng Biotechnol. 2020;8:756. doi: 10.3389/fbioe.2020.00756.
- Lugo-Méndez H, Sánchez-Domínguez M, Sales-Cruz M, et al. Synthesis of biodiesel from coconut oil and characterization of its blends. Fuel. 2021;295:120595. doi: 10.1016/j.fuel.2021.120595.
- Pullen J, Saeed K. An overview of biodiesel oxidation stability. Renewable Sust Energ Rev. 2012;16(8):5924–5950. doi: 10.1016/j.rser.2012.06.024.
- Chrysikou LP, Litinas A, Bezergianni S. Assessment of biodiesel stability under long-term storage and dynamic accelerated oxidation: a comparison approach. Clean Techn Environ Policy. 2022;24(8):2583–2593. doi: 10.1007/s10098-022-02335-9.
- Kumar N. Oxidative stability of biodiesel: causes, effects, and prevention. Fuel. 2017;190:328–350. doi: 10.1016/j.fuel.2016.11.001.
- Fregolente PBL, Fregolente LV, Wolf Maciel MR. Water content in biodiesel diesel, and biodiesel–diesel blends. J Chem Eng Data. 2012;57(6):1817–1821. doi: 10.1021/je300279c.
- da Costa Cardoso L, de Almeida FNC, Souza GK, et al. Synthesis and optimization of ethyl esters from fish oil waste for biodiesel production. Renew Ener. 2019;133:743–748. doi: 10.1016/j.renene.2018.10.081.
- Marzban N, Libra JA, Hosseini SH, et al. Experimental evaluation and application of genetic programming to develop predictive correlations for hydrochar higher heating value and yield to optimize the energy content. J Environ Chem Engin. 2022;10(6):108880. doi: 10.1016/j.jece.2022.108880.
- Portela NA, Oliveira EC, Neto AC, et al. Quantification of bio-diesel in petroleum diesel by 1H NMR: evaluation of univariate and multivariate approaches. Fuel. 2016;166:12–18. doi: 10.1016/j.fuel.2015.10.091.
- Ullah K, Ahmad M, Qiu F, et al., Assessing the experimental investigation of milk thistle oil for biodiesel production using base catalyzed transesterification. Ener. 2015;89:887–895. doi: 10.1016/j.energy.2015.06.028.
- Asci F, Aydin B, Akkus GU, et al. Fatty acid methyl ester analysis of as-pergillus fumigatus isolated from fruit pulps for biodiesel production using GC-MS spectrometry. Bioengin. 2020;11(1):408–415. doi: 10.1080/21655979.2020.1739379.
- Miglio R, Palmery S, Salvalaggio M, et al. Microalgae triacylglycerols content by FT-IR spectroscopy. J Appl Phycol. 2013;25(6):1621–1631. doi: 10.1007/s10811-013-0007-6.
- Atabani AE, Shobana S, Mohammed MN, et al. Integrated valorization of waste cooking oil and spent coffee grounds for biodiesel production: blending with higher alcohols, FT–IR, TGA, DSC and NMR characterizations. Fuel. 2019;244:419–430. doi: 10.1016/j.fuel.2019.01.169.
- Siatis NG, Kimbaris AC, Pappas CS, et al. Improvement of biodiesel production based on the application of ultrasound: monitoring of the procedure by FTIR spectroscopy. J Americ Oil Chem Soc. 2006;83(1):53–57. doi: 10.1007/s11746-006-1175-1.
- Andrade TA, Errico M, Christensen KV. Transesterification of castor oil catalyzed by liquid enzymes: optimization of reaction conditions. Comp Aided Chem Engin. 2017;40:2863–2868. doi: 10.1016/B978-0-444-63965-3.50479-7.
- Elango RK, Sathiasivan K, Muthukumaran C, et al. Transesterification of castor oil for biodiesel production: process optimization and characterization. Microchem J. 2019;145:1162–1168. doi: 10.1016/j.microc.2018.12.039.