Abstract
Excessive production of nitric oxide (NO) during the lactation period of cows leads to oxidative stress and inflammatory reactions. Chitosan has enhanced effects on antioxidant and immune function, but the mechanism of this effect is unclear. The current study investigated the underlying mechanism of the protective effect chitosan oligosaccharides (COS) exert against oxidative damage of peripheral blood mononuclear cells (PBMCs) in dairy cows. PBMCs were allocated to receive one of treatments: CTR: control treatment without COS addition; COS40, COS80, COS160, and COS320 received 40, 80, 160, and 320 μg/mL of COS; PBMCs were then treated with 200 μmol/L diethylenetriamine/nitric oxide (DETA/NO) for 4 h. Compared to the CTR group, the cell viability of the COS pre-treated group increased with increasing COS concentration. The activities of antioxidant enzymes including superoxide dismutase, catalase, and glutathione peroxidase and the thioredoxin reductase content showed an upward trend in a dose-dependent manner and COS at a concentration of 160 μg/mL exhibited the strongest effect. COS decreased the content and gene expressions of interleukin-1β and tumour necrosis factor-α, the mRNA and protein expression and activity of the inducible nitric oxide synthase, and NO production compared with the values observed in the CTR group. The expression of genes and proteins related to the nuclear factor kappa-B (NF-κB) pathway also showed a downward trend. Based on these indicators, we concluded COS has a protective effect against oxidative damage caused by DETA/NO, and we speculated that COS may play a protective role by inhibiting the NF-κB pathway.
In vitro experiments simulated changes in antioxidant and immune functions caused by increased NO in dairy cows’ PBMCs in vivo.
We showed that chitosan has a protective effect against oxidative damage and a regulatory effect on immune function in vitro.
The regulation of chitosan against oxidative stress and immune responses may be related to the NF-KB signalling pathway.
Highlights
Introduction
High yielding cows may experience oxidative stress during lactation period (Castillo et al. Citation2006). During calving and high mobilisation of body fat, cows with high body condition scores had more pronounced changes in their oxidative state, making them more susceptible to oxidative stress (Bernabucci et al. Citation2005). During the high-energy metabolism process, the body is prone to the effects of excess free radicals such as nitric oxide (NO), and the accumulation of free radicals exacerbates the inflammatory response in dairy cows (Sordillo and Aitken Citation2009). NO which is catalysed and produced mainly by inducible nitric oxide synthase (iNOS) is an active nitrogen radical with a simple structure, a small molecular weight, and has a bidirectionally regulated function. Studies have shown that NO produced by macrophages is involved in neuronal signalling, and has antibacterial and immune effects in vivo and in vitro (Duffield Citation2003). However, excessive increase in NO content causes inflammation and immune disorders in bighorn sheep (Sacco et al. Citation2006), and cause oxidative damage to bovine mammary epithelial cells (Shi et al. Citation2018). NO is produced by macrophages as a cytotoxic agent during the inflammatory response, and NO scavengers block the cytostatic effect of macrophages (Coleman Citation2001; Dedon and Tannenbaum Citation2004). Peripheral blood mononuclear cells (PBMCs) are an important class of immune effector cells that monitor infection and disease in the body, and they play an important role in mediating inflammation and pathogen clearance (Van der Meide and Schellekens Citation1996; Mastrogiovanni et al. Citation2019). In addition, certain biological properties of PBMCs are associated with disease resistance (Barnes et al. Citation1993). Therefore, reducing the excessive NO production of PBMC is one of the main methods used to relieve cellular oxidative stress.
Chitosan is a polycationic polymer composed mainly of glucosamine units and is known for its biocompatibility, biodegradability and low toxicity. However, the water insolubility of chitosan is a major limiting factor for industrial applications (Feng et al. Citation2009). Chitosan oligosaccharides (COS) are the enzymatic hydrolysates of chitosan and have a variety of biological activities such as water soluble, antioxidant, antihypertensive and antibacterial activity in human and mice (Muanprasat and Chatsudthipong Citation2017; Naveed et al. Citation2019). In addition, COS had anti-inflammatory effects in lipopolysaccharide (LPS)-treated RAW264.7 mouse macrophages in vitro; COS suppressed the production of proinflammatory cytokines such as tumour necrosis factor-α (TNF-α), interleukin-1β (IL-1β) and interleukin-6 (IL-6) induced by LPS (Cho et al. Citation2011). The protection by sulphated chitooligosaccharide (COS-S) against hydrogen peroxide-induced oxidative damage in the mouse pancreatic β-cell line MIN6 has been reported, which showed that COS-S significantly inhibits NO production, as well as iNOS activity and the mRNA and protein expression of nuclear factor kappa-B p65 (NF-κBp65) (Lu et al. Citation2013).
Diethylenetriamine/nitric oxide (DETA/NO) is a synthetic nitric oxide adduct that can spontaneously release NO at an expected rate without the catalytic action of an enzyme. It is an ideal NO donor and one of the NO adducts with the longest half-life. It can release NO stably for a long time, making it suitable for long-term in vitro observation experiments (Keefer et al. Citation1996; Shi et al. Citation2018). Previous studies have established the oxidative damage model of PBMCs using DETA/NO as an NO donor (Zheng et al. Citation2018). The current experiment mainly investigated the pre-protective effects of COS against oxidative damage of dairy cows’ PBMCs after treated with DETA/NO and the possible regulatory mechanisms.
Material and methods
The protocol of the present experiment was approved by the Animal Care and Use Committee (Inner Mongolia Agricultural University, Hohhot, China).
PBMCs isolation and culture
After collecting blood from the tail vein of three healthy Holstein dairy cows (200 ± 15d DIM, 34.8 ± 4.95 kg/d of milk yield, 3rd parity, 571.2 ± 63.6 kg of body weight, 3.0 ± 0.05 for BCS) in EDTA anticoagulant tubes, PBMCs were separated within 12 h. The health status of the cows was checked by a veterinarian at the ShaLiang Farm (Hohhot, China). The PBMC separation and culture methods were according to the researches of English and Andersen (Citation1974) and Ferrante and Thong (Citation1980). Briefly, the steps were as follows: PBMCs were isolated by single density gradient centrifugation (Ficol) with a bovine PBMC separation kit (Tianjin Haoyang Biological Products Technology Co., Ltd, Tianjin, China), and the separation operation was according to manufacturer’s instruction. The PBMCs layer was aspirated and washed with PBS. The isolated cells were cultured in 25 mL culture flasks (Corning Incorporated Costar, Corning, NY) using a Roswell Park Memorial Institute 1640 (RPMI-1640) medium supplemented with 10% foetal bovine serum (FBS). An aliquot of the cells were stained with trypan blue (Gibco, Carlsbad, CA) and counted to ensure a viability >95%.
Experimental design
The current experiment involved a completely random design. After 48 h of incubation in RPMI-1640 medium, the cells were collected and randomly divided into five treatment groups at 6 × 106 cells/mL with six replicates for each treatment group. Cells were allocated to receive one of treatments: CTR: control treatment without COS addition; COS40, COS80, COS160, and COS320 received 40, 80, 160, and 320 μg/mL of COS (Jinan Haidebei Marine Bioengineering Co., Ltd., Jinan, China; degree of deacetylation 90.27%, molecular weight <3000) respectively for 24 h after 48 h incubation. All the groups were cultured in a RPMI-1640 medium with 200 μmol/L of DETA/NO (Sigma, St. Louis, MO) for 4 h. The COS concentration and processing time were determined based on our previous studies by Zheng et al. (Citation2018). Cells and cell-free supernatant were collected from each treatment group to perform specific assays, as described in the following sections.
Measurement of relative cell viability
For relative cell viability measurement, 100 μL of PBMCs from each group have been inoculated into a 96 wells plate and added with 10 μL of cell counting kit-8 (CCK-8) incubation solution, according with manufacturer’s instruction. The optical density at 450 nm (OD) for each well have been measured through CCK-8 reader (Beyotime, Beijing, China), and the relative cell viability for each treatment well have been calculated dividing its OD value by the OD value found in the control well.
Measurement of enzyme activity
The catalase (CAT), superoxide dismutase (SOD), and glutathione peroxidase (GPx) activities and thioredoxin reductase (TrxR) concentration in the cell supernatant were measured by a spectrophotometer (UN2CO-WFT2100; Aoyi Co., Ltd., Shanghai, China) using spectrophotometric diagnostic kits (Nanjing Jiancheng Biotechnology Institute, Nanjing, China) according to the manufacturer’s instructions.
Enzyme-linked immunosorbent assays (ELISA)
The activities of inducible nitric oxide synthase (iNOS) and the contents of reactive oxygen species (ROS), NO, interleukin-1β (IL-1β), interleukin-6 (IL-6), and tumour necrosis factor-α (TNF-α) in the culture supernatants were determined by ELISA kits (Nanjing Jiancheng Biotechnology Institute, China); a standard curve was calculated, and the absorbance at 450 nm was measured according to the manufacturer’s instructions. The optical density was detected by using a microplate reader (BioTek Synergy H1, Winooski, VT).
RNA extraction and RT-PCR
RNA extraction and reverse transcription were performed using the RNAiso Plus, PrimeScriptTM RT reagent kit and SYBR premix EX TaqTM II (Takara Bio Inc., Beijing, China), according to the manufacturer’s instructions. Primers for bovine were designed from sequences in the GenBank database using Primer 3 (ver 4.0.0, Free Software Foundation Inc., Boston, MA). Primers are shown in Table . All primers were synthesised commercially (Sangon Biotech, Co., Ltd, Shanghai, China). The cDNA synthesis reaction was carried out at 37 °C for 15 min and 85 °C for 5 s. The RT products (cDNA) were stored at –20 °C for real-time PCR assay. PCR was carried out in a LightCycler 480 real-time PCR machine (Roche Diagnostics Ltd, Forrentrasse, Switzerland). Target genes were normalised by using two stable reference genes, β-actin and glyceraldehyde-3-phosphate dehydrogenase (GAPDH), which were evaluated based on their expression stability (M) and pairwise variation (V) values (V2/3 < 0.15) using GeNorm software (Vandesompele et al. Citation2002). The specificity of the PCR products were assessed by the melt curve analysis and subsequent gel agarose electrophoresis, and quantitative real-time PCR data were analysed using the 2−ΔΔCt method (Livak and Schmittgen, Citation2001).
Table 1. Primers sequences used in real-time PCR.
Western blot
PBMCs were collected, and proteins were extracted from the samples by radio immunoprecipitation assay (RIPA) lysis buffer (Beyotime, Beijing, China). After centrifugation at 12,000 g for 5 min the supernatants were collected to measure the protein concentration by using a BCA assay kit (P0012, Beyotime, Beijing, China). The collected protein was mixed with the loading buffer (P0015, Beyotime, China) and denatured at 100 °C for 5 min, followed by storage at 4 °C. SDS-PAGE was used to separate the total protein (30 μg) which was then electrophoretically transferred from the gel to polyvinylidene difluoride membranes (Merck Millipore, Billerica, CA). The membranes were incubated in the following primary antibodies: primary polyclonal rabbit anti-iNOS antibody (NBP1-97471, Novus Biological, USA), rabbit anti-IL-1β (Cell Signalling Technology, Boston, MA), anti-IKKβ, monoclonal rat anti-IκBα, monoclonal rabbit anti-NF-κBp65 antibody(NF-κB pathway Sampler Kit 9963,CST, MA), anti-phospho-IKKβ, anti-phospho-IκBα, and anti-phospho-NF-κBp65 (NF-κB pathway Sampler Kit 9963, CST, MA). The secondary antibody used goat anti-rat IgG (7074P2, KPL, MD). The basal levels of the proteins were normalised by analyse the level of β-actin (Rabbit mAb; 4790; CST, MA)protein. Relative protein levels were determined by ImageJ software (NIH Image J system, Bethesda, MD).
Data analysis
Data were analysed using variance statistics (GLM) of SAS (version 9.4, SAS Institute Inc., Cary, NC). The fixed effects in statistic model include treatment, linear and quadratic. Pairwise comparison has been done by the Duncan multiple comparisons test to determine the significance of the difference between the means. Statistical significance was declared at p < .05 and statistical trends at .05 ≤ p < .1.
Results and discussion
Effect of COS on cell viability treated with DETA/NO in PBMCs
Compared with the DETA/NO CTR group, the cell viability of all COS treatment groups increased significantly (p < .01), indicating that COS had protective effects in PBMCs against DETA/NO; the cell viability was increased in response to COS in a quadratic dose-dependent manner (p < .01) (Figure ). The cell viability in the COS160 group was significantly higher than that in the other groups.
Figure 1. Relative cell viability for peripheral blood mononuclear cells incubated with 40, 80, 160, or 320 μg/mL of chitosan oligosaccharides (COS40, COS80, COS160, and COS320). Values are expressed as an optical density ratio between treated and control (CTR) wells. The different letter superscripts mean significant difference (p < .05).
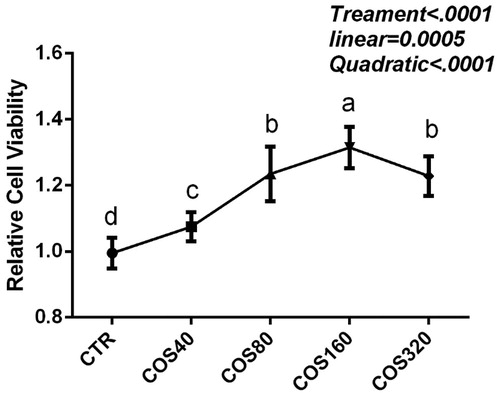
Effect of COS on the activity of antioxidant enzymes treated with DETA/NO in PBMCs
In our results, the activity of CAT increased linearly (p < .01) with increasing COS concentration. The SOD, TrxR, and GPx activities of the COS-treated groups were significantly higher than that of the CTR group (p < .05), and increased in response to COS addition in a quadratic dose-dependent manner (p < .01) (Table ). COS 160 exhibited the strongest effect in SOD and GPx. The effects of reactive oxygen species are controlled in vivo by a variety of enzymatic and non-enzymatic defence mechanisms. The activity of SOD, CAT and GPx reflects the antioxidant capacity to cope with oxidative stress (İnal et al. Citation2001). These results suggested that COS can enhance the antioxidant function of PBMCs and reduce oxidative stress. Oxidative stress may alter physiological functions and lead to pathological changes. Studies have shown that during prepartal and postpartum periods, exposure to severe physiological changes and metabolic stress has an important impact on the subsequent lactation performance and health of cows (Bernabucci et al. Citation2005).
Table 2. Effect of chitosan oligosaccharides on the antioxidant enzyme activity and concentration in peripheral blood mononuclear cells.
Effects of COS on inflammatory cytokines, NO, and ROS content and iNOS activity of PBMCs treated with DETA/NO
Our results showed that compared with the CTR group, the IL-1β content of the COS treatment group decreased significantly (p < .01), and was decreased in response to COS addition in a quadratic dose-dependent manner (p < .01) (Table ). The content of IL-1β, TNF-α and IL-6 in COS160 group was significantly lower than in the other groups. Level of these cytokines in COS320 group was higher than that of the COS160 group; however, it was still significantly lower than that in the control group. To further investigate the protective effects of COS, TNF-α, IL-1β, and IL-6 were measured at the transcriptional level. Compared with the CTR group, the transcription of IL-1β decreased significantly in a dose-dependent manner in all of the COS treatment groups, and the transcript levels of TNF-α and IL-6 showed a similar trend (Table ). The gene expression of TNF-α, IL-1β, and IL-6 decreased with increased COS additive. Compared with the CTR group, the levels of free radicals such as NO and ROS in the COS treatment groups were decreased in the COS80 and COS320 groups. The content was the lowest in the COS160 group, and showed a quadratic linear dose-dependent effect (Table ). The activity of iNOS decreased significantly (p < .01) and showed a linear decline with increasing dose of COS. These results indicated that COS can relieve oxidative stress and reduce inflammatory cytokine production, iNOS activity, and NO production.
Table 3. Effect of chitosan oligosaccharides on the inflammatory cytokines content and in peripheral blood mononuclear cells.
Table 4. Effect of chitosan oligosaccharides on genes expression of cytokines, inducible nitric oxide synthase and NF-κB pathway.
Research had shown that DETA/NO induced the increase expression of IL-8 and TNF-α, and simultaneously induced apoptosis in PBMC (Mühl et al. Citation1999). Excessive production of NO is accompanied by an increase in oxidative stress in the body and the production of many pro-inflammatory cytokines such as TNF-α, IL-1β, IL-6. Proinflammatory cytokines are mainly produced by activated macrophages and are involved in the up-regulation of inflammatory factors. These pro-inflammatory cytokines activate other immune cells and can cause inflammatory diseases such as rheumatoid arthritis and endotoxin-induced multiple organ damage (Sacco et al. Citation2006; Kim et al. Citation2014).
COS inhibit the expression of iNOS via the NF-κB pathway
We examined the gene expression of p50 and p65 as well as the protein expression of IKKβ, IKBα, and p65. Compared with the CTR group, the gene expression levels of p50 and p65 in all COS treatment groups were significantly decreased (p < .05), and showed a quadratic linear dose-dependent effect (Tables and and Figure ). The protein phosphorylation levels of IKBα, IKKβ and p65 decreased in response to COS addition in a quadratic dose-dependent manner (p < .01).
Figure 2. Effects of chitosan oligosaccharideon on the phosphorylation level of NF-kB pathway, the protein expression level of nitric oxide synthase (iNOS) and interleukin-1β (IL-1β). Expressions of IL-1β(A); phosphorylated IκB kinase β (P-IKKβ) (B); phosphorylated inhibitor of nuclear factor kappa-Bα (P-IκBα) (C); phosphorylated nuclear factor kappa-Bp65(P-NF-κBp65) (D); iNOS (E), protein levels were detected by western blotting and normalised to beta-actin (β-Actin) levels. CTR = control treatment, without chitosan oligosaccharides addition; COS40, COS80, COS160, and COS320= treated with 40, 80, 160, and 320 μg/mL chitosan oligosaccharides respectively.
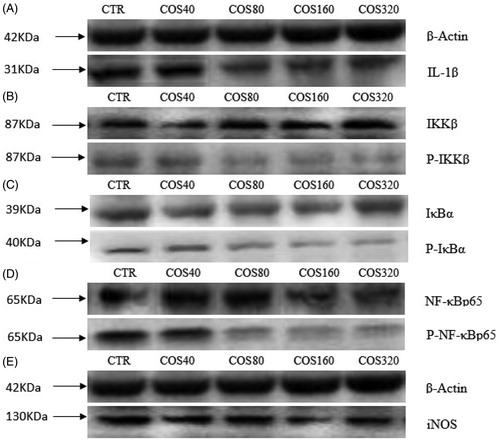
Table 5. Effect of chitosan oligosaccharides on inducible nitric oxide synthase, interleukin-1β protein expression, and phosphorylation of NF-κB pathways in PBMC.
NF-κB/Rel binds to specific DNA elements present on the promoter of the target gene, triggering transcription of various inflammatory cytokines, adhesion molecules, and chemokines (Galli et al. Citation1993). At least five different genes belong to the NF-κB family: NF-κB1 (p105/50), NF-κB2 (p100/p52), RelA (p65), RelB, and c-Rel. Most commonly, the NF-κB dimer consists of the Rel A (p65) and NF-κB 1 (p50) subunits. Phosphorylation plays an important role in regulating the NF-κB pathway (Mori et al. Citation1997). NF-κB is a family of heterodimeric transcription factors that are highly involved in a variety of physiological and pathological processes, including inflammation, immune response, and cell survival. In unstimulated cells, NF-κB binds to the inhibitory protein of the kappa B family (IκB) and is sequestered in the cytoplasm. Upon stimulation, IκB is phosphorylated by the IκB kinase (IKK) complex, which consists of two kinases, IKKα and IKKβ, and the necessary regulatory subunit (NEMO, also known as IKKγ or IKKAP). Phosphorylated IκB is subsequently ubiquitinated and degraded by the 26S proteasome, thereby translocating NF-κB to the nucleus and regulating the expression of numerous genes in the nucleus (Chen and Chen Citation2013).
Studies in which rat skeletal muscle myoblasts were incubated with inflammatory cytokines and iNOS expression was assessed by Western blotting showed that the stimulatory effect of IL-1β and IFN-γ in vitro induced iNOS expression in skeletal muscle by activating NF-κB (Teng et al. Citation2002). IL-1β stimulated the production of prostaglandins (PG) by the transcriptional activation of cyclooxygenase-2 (COX-2), which may involve the NF-κB signalling pathway (Cho et al. Citation2011).
The current research found that the IL-1β content and transcript and protein level declined under the action of COS. Combined with the gene expression and protein level of iNOS, we speculated that IL-1β may be involved in mediating the expression of iNOS. COS may inhibit the production of iNOS and the expression of gene and proteins of the NF-κB pathway, thereby inhibiting the production of NO and exerting an anti-inflammatory effect and promoting anti-oxidation.
Conclusions
In conclusion, these results showed that the addition of COS leads to enhanced antioxidant enzyme activity, reduced IL-1β production and gene and protein expression, and inhibition of the NF-κB signalling pathway activation on dose-dependent manner after treated with DETA/NO. It has been suggested that the mechanism of action may involve the inhibition of the NF-κB pathway and NO production.
Ethical Approval
The experimental protocol was approved by the Institutional Animal Care and Use Committee of Inner Mongolia Agricultural University.
Disclosure statement
The authors are as follows: Yaguang Zheng, Sumei Yan, Jingyu Qi, Yanli Zhao, Xiaoyu Guo and Binlin Shi declare that there are no conflicts of interest. The authors alone are responsible for the content and writing of this article.
Additional information
Funding
References
- Barnes PF, Lu S, Abrams JS, Wang E, Yamamura M, Modlin RL. 1993. Cytokine production at the site of disease in human tuberculosis. Infect Immun. 61(8):3482–3489.
- Bernabucci U, Ronchi B, Lacetera N, Nardone A. 2005. Influence of body condition score on relationships between metabolic status and oxidative stress in periparturient dairy cows. J Dairy Sci. 88(6):2017–2026.
- Castillo C, Hernandez J, Valverde I, Pereira V, Sotillo J, Alonso ML, Benedito JL. 2006. Plasma malonaldehyde (MDA) and total antioxidant status (TAS) during lactation in dairy cows. Res Vet Sci. 80(2):133–139.
- Chen J, Chen ZJ. 2013. Regulation of NF-κB by ubiquitination. Curr Opin Immunol. 25(1):4–12.
- Cho Y-S, Lee S-H, Kim S-K, Ahn C-B, Je J-Y. 2011. Aminoethyl-chitosan inhibits LPS-induced inflammatory mediators, iNOS and COX-2 expression in RAW264. 7 mouse macrophages. Process Biochem. 46(2):465–470.
- Coleman JW. 2001. Nitric oxide in immunity and inflammation. Int Immunopharmacol. 1(8):1397–1406.
- Dedon PC, Tannenbaum SR. 2004. Reactive nitrogen species in the chemical biology of inflammation. Arch Biochem Biophys. 423(1):12–22.
- Duffield JS. 2003. The inflammatory macrophage: a story of Jekyll and Hyde. Clin Sci. 104(1):27–38.
- English D, Andersen BR. 1974. Single-step separation of red blood cells, granulocytes and mononuclear leukocytes on discontinuous density gradients of Ficoll-Hypaque. J Immunol Methods. 5(3):249–252.
- Feng T, Haijun R, Binlin S. 2009. The effect of chitosan on milk production performance, number of breast cells and serum immunoglobulin in dairy cows. Feed Industry. 30(5):31–33.
- Ferrante A, Thong YH. 1980. Optimal conditions for simultaneous purification of mononuclear and polymorphonuclear leucocytes from human blood by the Hypaque-Ficoll method. J Immunol Methods. 36(2):109–117.
- Galli SJ, Zsebo KM, Geissler EN. 1993. The kit ligand, stem cell factor. Advances in immunology. London: Academic Press, 55: p. 1–96.
- İnal ME, Kanbak G, Sunal E. 2001. Antioxidant enzyme activities and malondialdehyde levels related to aging. Clin Chim Acta. 305(1–2):75–80.
- Keefer LK, Nims RW, Davies KM, Wink, DA. 1996. NONOates”(1-substituted diazen-1-ium-1, 2-diolates) as nitric oxide donors: convenient nitric oxide dosage forms[M]//Methods in enzymology. London: Academic Press, 268: p. 281–293.
- Kim J-H, Kim Y-S, Hwang J-W, Han Y-K, Lee J-S, Kim S-K, Jeon Y-J, Moon S-H, Jeon B-T, Bahk YY, et al. 2014. Sulfated chitosan oligosaccharides suppress LPS-induced NO production via JNK and NF-κB inactivation. Molecules. 19(11):18232–18247.
- Livak K J, Schmittgen T D. 2001. Analysis of Relative Gene Expression Data Using Real-Time Quantitative PCR and the 2−ΔΔCT Method. Methods. 25(4):402–408. doi:10.1006/meth.2001.1262.
- Lu X, Guo H, Sun L, Zhang L, Zhang Y. 2013. Protective effects of sulfated chitooligosaccharides with different degrees of substitution in MIN6 cells. Int J Biol Macromol. 52:92–98.
- Mastrogiovanni F, Romani A, Santi L, Lacetera N, Bernini R. 2019. Anti-proliferative effect of pomegranate peel extracts on bovine peripheral blood mononuclear cells (PBMCs). Nat Prod Res.33:1–6.
- Mori T, Okumura M, Matsuura M, Ueno K, Tokura S, Okamoto Y, Minami S, Fujinaga T. 1997. Effects of chitin and its derivatives on the proliferation and cytokine production of fibroblasts in vitro. Biomaterials. 18(13):947–951.
- Muanprasat C, Chatsudthipong V. 2017. Chitosan oligosaccharide: biological activities and potential therapeutic applications. Pharmacol Ther. 170:80–97.
- Mühl H, Nold M, Chang JH, Frank S, Eberhardt W, Pfeilschifter J. 1999. Expression and release of chemokines associated with apoptotic cell death in human promonocytic U937 cells and peripheral blood mononuclear cells. Eur J Immunol. 29(10):3225–3235.
- Naveed M, Phil L, Sohail M, Hasnat M, Baig MMFA, Ihsan AU, Shumzaid M, Kakar MU, Mehmood Khan T, Akabar MD, et al. 2019. Chitosan oligosaccharide (COS): an overview. Int J Biol Macromol. 129:827–843.
- Robinson TL, Sutherland IA, Sutherland J. 2007. Validation of candidate bovine reference genes for use with real-time PCR. Veterinary Immunology and Immunopathology. 115(1-2):160–165. doi:10.1016/j.vetimm.2006.09.012.
- Sacco RE, Waters WR, Rudolph KM, Drew ML. 2006. Comparative nitric oxide production by LPS-stimulated monocyte-derived macrophages from Ovis canadensis and Ovis aries. Comp Immunol Microbiol Infect Dis. 29(1):1–11.
- Shi HY, Yan SM, Guo YM, Zhang BQ, Guo XY, Shi BL. 2018. Vitamin A pretreatment protects NO-induced bovine mammary epithelial cells from oxidative stress by modulating Nrf2 and NF-κB signaling pathways. J Anim Sci. 96(4):1305–1316.
- Sordillo LM, Aitken SL. 2009. Impact of oxidative stress on the health and immune function of dairy cattle. Vet Immunol Immunopathol. 128(1–3):104–109.
- Teng X, Zhang H, Snead C, Catravas JD. 2002. Molecular mechanisms of iNOS induction by IL-1β and IFN-γ in rat aortic smooth muscle cells. Am J Physiol-Cell Physiol. 282(1):C144–C152.
- Van der Meide PH, Schellekens H. 1996. Cytokines and the immune response. Biotherapy. 8(3–4):243–249.
- Vandesompele J, De Preter K, Pattyn F, Poppe B, Van Roy N, De Paepe A, Speleman F. 2002. Accurate normalization of real-time quantitative RT-PCR data by geometric averaging of multiple internal control genes. Genome Biol. 3(7):research0034–1.
- Zheng Y, Jingyu Q, Sumei Y, Binlin S. 2018. Establishment of oxidative damage model of peripheral blood mononuclear cell induced by diethylenetriamine/nitric oxide adduct. Chinese J Anim Nutr. 9:021.