ABSTRACT
Living organisms experience tissue damage from both, the surrounding environment and from inside their bodies. Tissue repair/regeneration is triggered by local tissue injury to restore an injured, or lost, part of the body. Tissue damage results in a series of responses, not only locally but also systemically in distant tissues. In our recent publication, we established a “dual system” that induces spatiotemporal tissue damage simultaneously with gene manipulation in surrounding tissues. With this system, we demonstrated that appropriate regulation of methionine metabolism in the fat body is required for tissue repair in Drosophila wing discs, thus highlighting the importance of systemic damage response (SDR) in tissue repair. This “Extra View” aims to discuss our recent reports that propose methionine metabolism to be an essential part of SDR, together with related topics in several model organisms.
Introduction
Organisms are inevitably affected by their internal and external environments. In multicellular organisms in particular, numerous tissue interactions establish a complex internal environment and are crucial for protection against various stresses. One of the most severe insults to the body is tissue injury. Tissue injury often occurs from the outside because of physical injury. A wound is defined as a disruption of the outer physical barrier, causing not only a local wound response (LWR) but also a systemic wound response (SWR). Green and Ryan observed that when potato or tomato leaves were injured either physically or by herbivore attack, proteinase inhibitors accumulated not only at the site of injury but also in uninjured leaves.Citation1 Extensive studies in plants have identified systemic defense mechanisms that underlie this wound response. When tomato leaves are injured, the jasmonic acid biosynthetic pathway is locally activated at the wound site. Jasmonic acid then acts as a mobile wound signal and travels to other parts of the body to induce genes that encode plant defensins and anti-microbial peptides, in preparation for the next injury.Citation2
A systemic response upon tissue injury is not only restricted to the wound but also involves a wide range of tissue damage responses as suggested in various reports, including those discussed below. Thus, we will use the terms systemic damage response (SDR) and local damage response (LDR) in the following sections. SDR is a reasonable homeostatic mechanism for multicellular organisms because the influence of local damage is not always confined to the damaged region (). For instance, local tissue injury potentially causes infection, body fluid leakage, or chronic pain, which would require the systemic response in an organism. Thus, investigation of SDR is significant for understanding the physiology of multicellular organisms. To elucidate the molecular mechanisms of SDR, it is essential to analyze damaged tissues and responsible tissues separately. Recent development of genetic tools has enabled us to manipulate genes tissue-specifically and tissue-independently. In our recent paper, we established a “dual system” using Drosophila genetics to study tissue repair/regeneration from the viewpoint of SDR.Citation3 Here, we introduce our recent studies of SDR and methionine metabolism, and discuss related studies and future prospects.
Establishment of a new Drosophila model to study the functional SDR for tissue repair and regeneration
The imaginal discs of Drosophila, epithelial sheets fated to become adult organs, are known to have a remarkable ability to repair after tissue damage.Citation4-6 In our study, we established a temporal cell ablation system in imaginal discs by using a temperature-sensitive form of the diphtheria toxin A domain (DtAts).Citation3,7 DtAts could induce cell death at low temperatures (18°C), but was inactive at high temperatures (29°C) Citation7,8, thus enabling us to control the timing of induction of tissue injury by simply placing DtAts-expressing larvae at 18°C (). In previous studies, a similar system for spatiotemporal genetic ablation was established, based on the Gal4/UAS/Gal80ts system.Citation9,10 The Gal4/UAS system is a budding yeast-based binary expression system for tissue-specific gene manipulation.Citation11 Gal4 binds to UAS (upstream activating sequence) and induces transcription of downstream target gene. Gal80ts, a temperature-sensitive Gal4 suppressor, controls the Gal4/UAS-based gene expression in a temperature dependent manner.Citation12 Tissue injury can be regulated both spatially and temporally by ectopic expression of cell death-inducing genes using these sophisticated genetic tools. However, simple utilization of Gal80ts system does not allow the uncoupling of tissue damage induction and the manipulation of genes of interest (i.e. tissue repair genes) using the currently available genetic resources, such as the UAS-RNAi library. Alternative approaches involve using whole body loss-of-function mutations Citation9,13, but this cannot tell us specifically where in the organism the gene works. Therefore, the new system is required to test the non cell-autonomous function of the genes/pathways in surrounding tissues.
Figure 1. Dual system for studying systemic damage responses during tissue regeneration (A) Schematic view of temporal ablation with DtAts. DtAts induces cell death at low temperatures (18°C) and is inactivated at high temperatures (29°C). Thus, by shifting temperatures, a temporal ablation can be induced. With wing disc specific DtAts expression, local tissue injury and subsequent tissue repair can be observed in wing discs. The extent of wing regeneration can be estimated by observing the adult wing phenotype. DtAts: temperature-sensitive form of the diphtheria toxin A domain. (B) Independent tissue-specific gene manipulation by the combination of binary systems. Combination of Gal4/UAS, LexA, or Q system makes it possible to manipulate gene expression in independent tissues. For instance, tissue X specific gene promoter can induce the expression of QF only in tissue X and QF subsequently binds to QUAS, which results in the expression of gene A with no induction of other extrinsic binary system like Gal4/UAS system. Similarly, tissue Y specific gene promoter regulates the expression of gene B through the tissue specific expression of Gal4 and following binding to UAS. (C) Identifying genes required for systemic damage response. Combining a Gal4-based genetic manipulation and a Q-based DtAts ablation, the systemic factors required for disc repair can be investigated in uninjured and remote tissues. Manipulation of different genes in different tissues can be performed by changing the combination of Gal4 drivers or UAS lines. Together with a UAS-RNAi library, this dual system makes it possible to perform genetic screening for studying the tissue interactions involved in tissue repair.
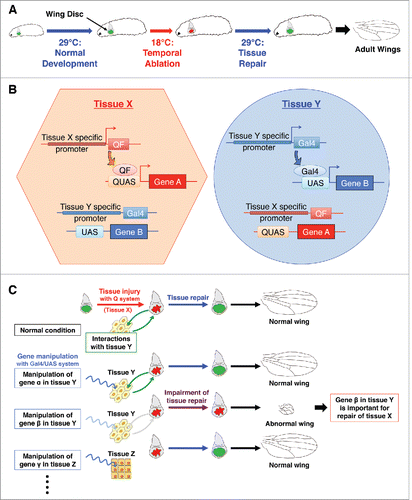
Drosophila has genetic tools available for tissue-specific gene manipulation with several binary genetic systems in addition to the Gal4/UAS system. Systems such as the LexACitation14 or Q systemsCitation15,16 can be utilised independently of Gal4/UAS. The combination of 2 systems makes it possible to control gene A in tissue X while manipulating gene B in tissue Y in the same animal (). In previous studies, the combination of binary genetic systems have been utilised to screen for functional ligand receptors. For example, the Gal4/UAS and LexA systems were combined successfully to identify the receptor of Drosophila insulin-like peptide 8 (Dilp8).Citation17 In our research, we overexpressed DtAts in imaginal discs to control temporal damage using the Q system, while simultaneously manipulating Gal4/UAS-mediated gene expression in non-ablated tissues (). Such a “dual system” enables us to search for non-autonomous factors contributing to local tissue repair, even by genome wide RNAi screening, for example.
Table 1. Selected examples of SDR in Drosophila.
Figure 2. Systemic damage responses of the fat body methionine metabolism (A) Methionine metabolism in Drosophila. Methionine is converted to SAM by Sams. SAM provides a methyl group for various methyltransferases including Gnmt, and becomes SAH. SAH is further metabolized into homocysteine, which is either regenerated into methionine, or converted into cystathionine through the transsulfuration pathway. SAM also provides an aminopropyl group to synthesize polyamines, such as spermidine and spermine. SAM: S-adenosylmethionine, Sams: SAM synthase, Gnmt: glycine n-methyltransferase, SAH: S-adenosylhomocysteine, CBS: cystathionine β synthase, Ahcy: adenosylhomocysteinases. (B) Enhancement of SAM metabolism in the fat body affects energy wasting and aging. Defects in apoptosis cause necrosis that triggers a systemic immune response including Toll activation in the fat body. dFoxO is activated concomitantly, and induces Gnmt that converts SAM into SAH, possibly functioning as an adaptive response against energy wasting. As aging proceeds, Gnmt is upregulated in the fat body however SAM level is also increased. Enhancing the dFoxO-Gnmt axis rescues age-dependent SAM accumulation and extends the lifespan. (C) Fat body methionine metabolism remotely regulates local tissue repair. Local disc injury non-autonomously affects methionine metabolism in the fat body via an unidentified “Help Me” signal. In turn, appropriate regulation of methionine metabolism in the fat body is required for sufficient tissue repair in damaged discs.
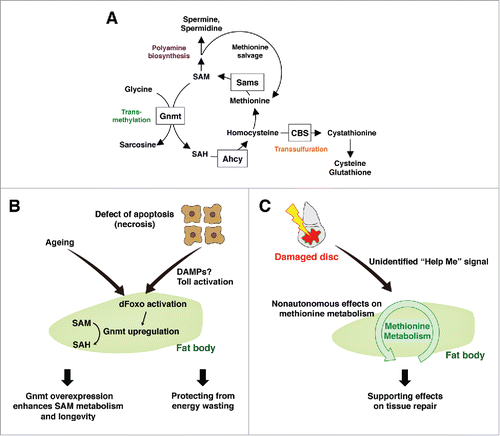
Involvement of methionine metabolism in SDR
Methionine is an essential amino acid, which must be regularly consumed through our diet. In addition to being a protein constituent, methionine is a precursor of S-adenosylmethionine (SAM), a versatile metabolite required for methylation of many substrates, such as nucleic acids, metabolites, lipids, and proteins, including histones. Methionine, together with ATP, is converted to SAM by a highly conserved enzyme called methionine adenosyltransferase (MAT). In Drosophila, only one SAM synthase (sams) functions to produce SAM (). The product of SAM-dependent methylation is S-adenosylhomocysteine (SAH), which in turn inhibits methyltransferase activity. Thus, the SAM/SAH ratio can be regarded as a methylation index. SAH is converted to homocysteine (Hcy) by adenosylhomocysteinases (Ahcy). Hcy is either metabolised to cystathionine through the transsulfuration pathway (TSP) via an enzymatic reaction mediated by cystathionine β synthase (CBS), or recycled into methionine. Cystathionine is further converted to cysteine and contributes to redox homeostasis mainly via de novo glutathione (GSH) production.Citation18,19 A computational estimate suggested that there are approximately 200 putative methyltransferase genes in the human genome.Citation20 Of these, glycine N-methyltransferase (Gnmt) seems to be the predominant consumer of SAM for converting glycine into sarcosine in liver, a tissue where ∼85% of methylation reaction takes place.Citation21 Thus, Gnmt is believed to tightly regulate SAM levels, and the amount of SAM is dramatically increased in Gnmt loss of function mutants.Citation22-24
From transcriptome and metabolome analyses in our previous study, we unexpectedly observed that apoptosis-deficient flies displayed altered methionine metabolism, triggered by necrotic tissue damage in adult wings.Citation24 Necrotic wings induce Gnmt in the fat body, which contributes to a reduction in SAM and induction of SAH, leading to a decreased methylation index. Gnmt induction in apoptosis-deficient mutants is due to FoxO activation in the fat body, in response to the systemic activation of innate immunity by necrosis in wing epidermal cells. Contrary to our initial assumption that Gnmt induction and a reduced methylation index may contribute to the pathological phenotypes, the decreased SAM levels seem to be an adaptive response to suppress the energy wasting in necrotic mutantsCitation24. FoxO-induced Gnmt is not only observed in necrotic models, but also in various conditions including epidermal physical injury (pricking, Obata and Miura, unpublished), starvation,Citation24 and aging.Citation25 Considering that Gnmt is also transcriptionally induced by Nrf2/CncC,Citation25 a transcriptional factor responsible for either oxidative or xenobiotic stress response, Gnmt-mediated changes in methionine metabolism might be a general mechanism as a part of SDR.
To test whether methionine metabolism is also affected by local tissue damage in our new larval model developed to study systemic control of tissue repair, we quantified the amount of methionine metabolites in the larvae expressing DtAts in the imaginal discs. As we expected, we found a decrease in methionine and SAM, and an increase in SAH in the fat body after disc ablation, particularly in the early stage of disc repair (6 h after ablation).Citation3 These data suggest that methionine metabolism in the fat body responds to local tissue damage in wing discs. Notably, Gnmt expression did not change at either the transcript or the protein level following ablation, unlike the necrosis model in adult flies. This implies that SAM consumption by methyltransferases other than Gnmt was up-regulated in the fat body. Instead, DtAts ablation in wing discs upregulated sams in the fat body, probably as an adaptive response against SAM reduction, which is also similar to the adaptive increase of MAT2A expression observed after partial hepatectomy in the rat.Citation26 Interestingly, a decline in SAM and an increase in SAH were also observed during liver regeneration. As for rat liver regeneration, hepatic SAM levels fell to about 40% of baseline at 12 and 24 h following partial hepatectomy (PH), which is coincident with an increase in SAH and a decrease in hepatic DNA methylation.Citation26 This is strikingly similar to the non-autonomous changes seen in the Drosophila fat body during the early stage of disc repair, although mammalian liver cases have been studied in a tissue-autonomous context. The question then arises, how is SAM converted to SAH? It has been reported that methylation of a histone and a cytosolic 20-kDa protein peaked at about 24 h after PH.Citation27,28 The identity of the 20-kDa protein is still unknown, and its contribution to liver regeneration has not been determined, but it has been suggested that the increased methylation is associated with cell proliferation and transformation.Citation27,28 It is unclear whether the fat body simply works as a source of SAM for methylation in regenerating discs or if some methylated factors in the fat body directly or indirectly support disc repair. Therefore, the precise identification and analysis of methylation target(s) or other downstream targets of methionine metabolism are required for further understanding of the process.
Functional analysis of methionine metabolism in tissue repair
Functional contributions to tissue repair could be assessed by combining Q-dependent DtAts-induced wing disc ablation and a fat body Gal4 driver.Citation3 As we reported, both knockdown and overexpression of gnmt or sams in the fat body impaired the repair of wing discs, while these genetic manipulations had no apparent effects on normal wing development. This indicates that appropriate regulation of methionine metabolism in the fat body is only necessary for disc repair. Additionally, overexpression of a dominant negative form of the insulin receptor (InRDN) in the fat body did not affect the adult wing morphology. This negates the idea that simple fat body dysfunction causes the impairment of disc repair and sheds light on the importance of methionine metabolism as a systemic modulator of tissue repair.Citation3
Although it is still unclear how both up- and down-regulation of gnmt or sams impairs disc repair, both MAT 1A−/− and GNMT−/− mutant mice showed impaired liver regeneration.Citation29,30 Therefore, the effect of methionine metabolism on tissue repair must be evolutionally conserved. In the case of MAT 1A−/− mice, S phase hepatocytes were significantly decreased in the injured liver, while hepatic apoptosis was markedly increased in the liver of GNMT−/− mice. Although it has not been determined whether methionine metabolism metabolites work in damaged or uninjured tissues, further studies will reveal the exact molecular mechanisms that underlie tissue regeneration.
Potential trigger of methionine metabolism changes in SDR
In animals, tissue damage leads to the release of damage- (or danger-) associated molecular patterns (DAMPs) and activation of the innate immune response.Citation31,32 DAMPs release is often followed by non-apoptotic cellular damage, including plasma membrane rupture and swelling of cells, which are hallmarks of necrosis and associated with pathological conditions such as traumatic injuryCitation33 and spontaneous tumor formation.Citation34 The larval haemolymph of the apoptosis-deficient mutant contains DAMPs that activate the Toll innate immune pathway.Citation35 In Drosophila, DAMPs activate a proteolytic cascade of haemolymph to generate the mature form of the Toll ligand, Spätzle (Spz).Citation36 Spz activates an innate immune reaction not only in blood cells but also in non-circulating cells, such as the fat body. Activated Spz overexpression leads to induced FoxO-Gnmt in adult flies, suggesting that systemic immune activation is sufficient to alter methionine metabolism.Citation24 However, DtAts-induced tissue damage in the wing discs did not induce immune activation, at least in the fat body (Kashio, Obata, and Miura, unpublished). This is consistent with the fact that Gnmt expression is not induced in these animals. Thus, additional systemic mediator(s) other than DAMPs, may exist and function as “Help Me” signals from damaged discs to other tissues, which would positively regulate SDR in tissue regeneration.
SDR studies and their relevance to methionine metabolism
The Drosophila model has contributed significantly to studies of SDR.Citation37 When integumental wounding by simple pricking was performed in adult Drosophila, an inter-tissue reaction was observed in the intestine and neurons. In the intestine, enterocytes, gut epithelial cells, reacted to integumental wounding. The IL-6-like cytokine Upd-3 was induced, and caspases were activated in the enterocytes. When caspase activation was prevented in enterocytes, animals became susceptible to integumental wounding.Citation38 Another paper demonstrated that wounding of the cuticle induced the production of Upd-2, -3 by haemocytes through JNK activation, which activates JAK/STAT signaling in visceral muscles and intestinal stem cells and regulates gut epithelial renewal.Citation39 These studies showed that SDR in the gut is required for organismal survival against integumental wounding. Integumental wounding activated the local production of reactive oxygen species (ROS) by dual oxidase (DUOX) or systemic ROS production through activation of pro-phenoloxidase by the haemocyte-derived phenoloxidase-activating enzyme Hayan, a CLIP-domain-containing serine protease. Integumental wounding activated neuronal JNK, and phenoloxydase-mediated ROS was required for this JNK activation.Citation40 Hayan mutants are susceptible to wound-induced lethality and can be rescued when the neuronal antioxidant pathway is reduced or JNK activity is enhanced in neurons.Citation40 ROS could be one of the upstream mediators of distant tissue reactions because ROS is also required for caspase activation in enterocytes after wounding.Citation38
Drosophila metastatic tumors (RasV12/scribble−/− clones in imaginal discs) or neoplastic tumors in scribble−/− mutants disrupt the basement membrane.Citation41 As in wounding, JNK and Upd-3 are up-regulated in tumors. Circulating haemocytes adhere to tumors, and Upd-3 is induced in the haemocytes. This represents positive feedback between Upd-3 expression and systemic activation of JAK/STAT signaling. Importantly, the fat body, a tissue distant from the injured site, is also engaged in the amplification loop of JAK/STAT signaling. Haemocytes proliferate via JAK/STAT signaling and attach to tumors to restrict their growth. A pinching method was used to induce physical aseptic tissue damage of the imaginal disc, and haemocyte recruitment to the damage site and amplification of JAK/STAT signaling were initiated.Citation42 Vidal's group also showed that imaginal disc tumors non-autonomously trigger a tumor suppressor response via Toll activation in the fat body.Citation43 These studies of SDR in Drosophila reveal bi-/multi-directional tissue interactions during the tissue damage response and following homeostatic regulation in the host. While it has not been studied whether methionine metabolism is also involved in tumor-inducing SDR, some cancers are reported to have a common feature of an absolute requirement for methionine, known as methionine dependency.Citation44 It would be interesting to determine whether methionine metabolism is altered in tumor-dependent SDR and involved in tumor progression.
Traumatic brain injury (TBI) in Drosophila revealed a secondary, non-mechanical injury in the intestine.Citation45 TBI increased intestinal permeability, and glucose feeding stimulated intestinal permeability, which enhanced lethality after TBI. Leakage of bacteria from the gut lumen then activated an innate immune response. Although the precise role of this immune response in survival after TBI has not been determined,Citation46 this observation provides a clear example of TBI-induced SDR in the gut. Intriguingly, the human patient with TBI had altered amounts of methionine and SAM in their blood,Citation47 which is similar to the case observed in Drosophila disc repair. The direct relevance to methionine metabolism on TBI-induced SDR has not been studied yet, but this suggests the evolutionally conserved significance of methionine metabolism in SDR.
Future directions
Some diseases or metabolic abnormalities cause perturbation of methionine metabolism. In humans, diabetes causes an increase in the amount of Met and SAM.Citation48 Interestingly, diabetic patients also exhibit impaired wound healing. This wound healing defect is reportedly caused by an impairment in nitric oxide (NO)-mediated endothelial progenitor cells homing to the injured site.Citation49 It was also reported that 5-methyltetrahydrofolate, the predominant form of dietary folate, regenerated tetrahydrobiopterin (BH4), a cofactor for producing NO with endothelial nitric oxide synthase (eNOS).Citation50 Although it is unclear whether eNOS function is directly affected by methionine metabolism, it remains likely that a diabetes-induced wound-healing defect is related to disruption of methionine metabolism because methionine metabolism is linked to folate metabolism in mammal. On the contrary, a low nutritional condition induced by a diet lacking in protein also impaired wound healing, but supplementation with Met was able to rescue the formation of connective tissue.Citation51 It is possible that Met regulates the rate of available protein utilization for connective tissue generation, or it may be utilised as a source of SAM, polyamine, or sulfate at the wound site. This report demonstrated the specific role of methionine metabolism in tissue repair and highlighted the potential of methionine metabolism regulation as a clinical target.
A number of reports have revealed the underlying mechanisms and importance of SDR, but many crucial questions remain unanswered. How is SDR induced? Why is SDR required? What are the mediators among the tissues? One major approach for understanding the mediators is to focus on the secreted factors. In a recent study, in vitro screening was used to show that myristoylated alanine-rich C kinase substrate (MARCKS)-like protein, a secreted factor, is required for the initial cell cycle response during axolotl appendage regeneration.Citation52 Mutant zebrafish, lacking most haematopoietic tissues, showed a regeneration defect accompanying apoptosis in regenerating fin after amputation.Citation53 In the tail explant culture, extracts from the bodies of wild-type zebrafish larvae rescued the apoptosis of regenerative cells in the haematopoietic tissue-deficient mutant, implying that diffusible factor(s) from haematopoietic tissue support the survival of regenerative cells.Citation53 These results prompted the functional screening of secretory factors (i.e., the secretomeCitation54) and the use of Drosophila genetics to understand the molecular mechanisms of SDR. Studies that focus on tissue interactions and the internal milieu (i.e., systemic biology) will further reveal the complex systems that multicellular organisms use for survival. Ultimately, such studies will contribute to pharmaceutical progress as well as to understanding the essential homeostatic mechanisms of multicellular organisms.
Abbreviations
LWR | = | local wound response |
SWR | = | systemic wound response |
SDR | = | systemic damage response |
LDR | = | local damage response |
DtAts | = | temperature-sensitive form of the diphtheria toxin A domain |
Dilp8 | = | Drosophila insulin-like peptide 8 |
SAM | = | S-adenosylmethionine |
MAT | = | methionine adenosyltransferase |
sams | = | SAM synthase |
SAH | = | S-adenosylhomocysteine |
Hcy | = | homocysteine |
Ahcy | = | adenosylhomocysteinases |
TSP | = | transsulfuration pathway |
CBS | = | cystathionine β synthase |
GSH | = | glutathione |
Gnmt | = | glycine N-methyltransferase |
PH | = | partial hepatectomy |
InRDN | = | dominant negative form of the insulin receptor |
DAMPs | = | damage- or danger- associated molecular patterns |
Spz | = | Spätzle |
ROS | = | reactive oxygen species |
DUOX | = | dual oxidase |
TBI | = | traumatic brain injury |
VZ | = | ventricular zone |
NPCs | = | neural precursor cells |
NO | = | nitric oxide |
BH4 | = | tetrahydrobiopterin |
eNOS | = | endothelial nitric oxide synthase |
MARCKS | = | myristoylated alanine-rich C kinase substrate |
Upd | = | unpaired |
JNK | = | Jun: c-Jun N-terminal kinase |
JAK/STAT | = | Janus kinase/signal transducer and activator of transcription |
Disclosure of potential conflicts of interest
No potential conflicts of interest were disclosed.
Funding
This work was partially supported by grants from the Japan Society for the Promotion of Science (to S. K., F.O. and M.M.), and AMED-CREST from Japan Agency for Medical Research and Development, AMED (to M.M.).
References
- Green TR, Ryan CA. Wound-Induced Proteinase Inhibitor in Plant Leaves: A Possible Defense Mechanism against Insects. Science 1972; 175:776-7; PMID:17836138; http://dx.doi.org/10.1126/science.175.4023.776
- Schilmiller AL, Howe GA. Systemic signaling in the wound response. Curr Opin Plant Biol 2005; 8:369-77; PMID:15939667; http://dx.doi.org/10.1016/j.pbi.2005.05.008
- Kashio S, Obata F, Zhang L, Katsuyama T, Chihara T, Miura M. Tissue nonautonomous effects of fat body methionine metabolism on imaginal disc repair in Drosophila. Proc Natl Acad Sci U S A 2016; 113:1835-40; PMID:26831070; http://dx.doi.org/10.1073/pnas.1523681113
- Hadorn E, Buck D. On the differentiation of transplanted wing imaginal disc fragments of Drosophila melanogaster. Rev Suisse Zool 1962; 69:302-10
- Worley MI, Setiawan L, Hariharan IK. Regeneration and transdetermination in Drosophila imaginal discs. Annu Rev Genet 2012; 46:289-310; PMID:22934642; http://dx.doi.org/10.1146/annurev-genet-110711-155637
- Kashio S, Obata F, Miura M. Interplay of cell proliferation and cell death in Drosophila tissue regeneration. Dev Growth Differ 2014; 56:368-75; PMID:24819984; http://dx.doi.org/10.1111/dgd.12139
- Bellen HJ, D'Evelyn D, Harvey M, Elledge SJ. Isolation of temperature-sensitive diphtheria toxins in yeast and their effects on Drosophila cells. Development 1992; 114:787-96; PMID:1618142; http://www.ncbi.nlm.nih.gov/pubmed/1618142
- Lee JW, Cho E, Aghaian E, Aghaian E, Der J, Wisnieski BJ. Characterization of a cloned temperature-sensitive construct of the diphtheria toxin A domain. Biochemistry 2005; 44:2555-65; PMID:15709768; http://dx.doi.org/10.1021/bi048317p
- Smith-Bolton RK, Worley MI, Kanda H, Hariharan IK. Regenerative growth in Drosophila imaginal discs is regulated by Wingless and Myc. Dev Cell 2009; 16:797-809; PMID:19531351; http://dx.doi.org/10.1016/j.devcel.2009.04.015
- Bergantinos C, Corominas M, Serras F. Cell death-induced regeneration in wing imaginal discs requires JNK signalling. Development 2010; 137:1169-79; PMID:20215351; http://dx.doi.org/10.1242/dev.045559
- Brand AH, Perrimon N. Targeted gene expression as a means of altering cell fates and generating dominant phenotypes. Development 1993; 118:401-15; PMID:8223268; http://www.ncbi.nlm.nih.gov/pubmed/8223268
- McGuire SE, Le PT, Osborn AJ, Matsumoto K, Davis RL. Spatiotemporal rescue of memory dysfunction in Drosophila. Science 2003; 302:1765-68; PMID:14657498; http://dx.doi.org/10.1126/science.1089035
- Schuster KJ, Smith-Bolton RK. Taranis Protects Regenerating Tissue from Fate Changes Induced by the Wound Response in Drosophila. Dev Cell 2015; 34:119-28; PMID:26096735; http://dx.doi.org/10.1016/j.devcel.2015.04.017
- Lai SL, Lee T. Genetic mosaic with dual binary transcriptional systems in Drosophila. Nat Neurosci 2006; 9:703-09; PMID:16582903; http://dx.doi.org/10.1038/nn1681
- Potter CJ, Tasic B, Russler EV, Liang L, Luo L. The Q system: a repressible binary system for transgene expression, lineage tracing, and mosaic analysis. Cell 2010; 141:536-48; PMID:20434990; http://dx.doi.org/10.1016/j.cell.2010.02.025
- Riabinina O, Luginbuhl D, Marr E, Liu S, Wu MN, Luo L, Potter CJ. Improved and expanded Q-system reagents for genetic manipulations. Nat Methods 2015; PMID:25581800; http://dx.doi.org/10.1038/nmeth.3250
- Colombani J, Andersen DS, Boulan L, Boone E, Romero N, Virolle V, Texada M, Leopold P. Drosophila Lgr3 Couples Organ Growth with Maturation and Ensures Developmental Stability. Curr Biol 2015; 25:2723-9; PMID:26441350; http://dx.doi.org/10.1016/j.cub.2015.09.020
- Mosharov E, Cranford MR, Banerjee R. The quantitatively important relationship between homocysteine metabolism and glutathione synthesis by the transsulfuration pathway and its regulation by redox changes. Biochemistry 2000; 39:13005-11; PMID:11041866; http://dx.doi.org/doi/10.1021/Bi001088w
- Eriksson S, Prigge JR, Talago EA, Arner ESJ, Schmidt EE. Dietary methionine can sustain cytosolic redox homeostasis in the mouse liver. Nat Commun 2015; 6: PMID:25790857; http://dx.doi.org/10.1038/Ncomms7479
- Petrossian TC, Clarke SG. Uncovering the human methyltransferasome. Mol Cell Proteomics 2011; 10:M110 000976; PMID:20930037; http://dx.doi.org/10.1074/mcp.M110.000976
- Lu SC, Mato JM. S-adenosylmethionine in liver health, injury, and cancer. Physiol Rev 2012; 92:1515-42; PMID:23073625; http://dx.doi.org/10.1152/physrev.00047.2011
- Liu SP, Li YS, Chen YJ, Chiang EP, Li AFY, Lee YH, Tsai TF, Hsiao M, Hwang SF, Chen YMA. Glycine N-methyltransferase−/− mice develop chronic hepatitis and glycogen storage disease in the liver. Hepatology 2007; 46:1413-25; PMID:17937387; http://dx.doi.org/10.1002/hep.21863
- Martinez-Chantar ML, Vazquez-Chantada M, Ariz U, Martinez N, Varela M, Luka Z, Capdevila A, Rodriguez J, Aransay AM, Matthiesen R, et al. Loss of the glycine N-methyltransferase gene leads to steatosis and hepatocellular carcinoma in mice. Hepatology 2008; 47:1191-99; PMID:18318442; http://dx.doi.org/10.1002/bep.22159
- Obata F, Kuranaga E, Tomioka K, Ming M, Takeishi A, Chen CH, Soga T, Miura M. Necrosis-driven systemic immune response alters SAM metabolism through the FOXO-GNMT axis. Cell reports 2014; 7:821-33; PMID:24582964; http://dx.doi.org/10.1016/j.celrep.2014.03.046
- Obata F, Miura M. Enhancing S-adenosyl-methionine catabolism extends Drosophila lifespan. Nat Commun 2015; 6:8332; PMID:26383889; http://dx.doi.org/10.1038/ncomms9332
- Huang ZZ, Mao Z, Cai J, Lu SC. Changes in methionine adenosyltransferase during liver regeneration in the rat. Am J Physiol 1998; 275:G14-21; PMID:9655679; http://www.ncbi.nlm.nih.gov/pubmed/9655679
- Tidwell T, Allfrey VG, Mirsky AE. The methylation of histones during regeneration of the liver. J Biol Chem 1968; 243:707-15; PMID:5638586; http://www.ncbi.nlm.nih.gov/pubmed/5638586
- Kwon SY, Kim S, Lee K, Kim TJ, Lee SH, Lee KM, Park GH. Increased methylation of the cytosolic 20-kD protein is accompanied by liver regeneration in a hepatectomized rat. Exp Mol Med 2004; 36:85-92; PMID:15031676
- Chen L, Zeng Y, Yang H, Lee TD, French SW, Corrales FJ, Garcia-Trevijano ER, Avila MA, Mato JM, Lu SC. Impaired liver regeneration in mice lacking methionine adenosyltransferase 1A. FASEB J 2004; 18:914-6; PMID:15033934; http://dx.doi.org/10.1096/fj.03-1204fje
- Varela-Rey M, Fernandez-Ramos D, Martinez-Lopez N, Embade N, Gomez-Santos L, Beraza N, Vazquez-Chantada M, Rodriguez J, Luka Z, Wagner C, et al. Impaired liver regeneration in mice lacking glycine N-methyltransferase. Hepatology 2009; 50:443-52; PMID:19582817; http://dx.doi.org/10.1002/hep.23033
- Gallucci S, Matzinger P. Danger signals: SOS to the immune system. Curr Opin Immunol 2001; 13:114-9; PMID:11154927; http://dx.doi.org/10.1016/S0952-7915(00)00191-6
- Matzinger P. The danger model: a renewed sense of self. Science 2002; 296:301-5; PMID:11951032; http://dx.doi.org/10.1126/science.1071059
- Gadani SP, Walsh JT, Lukens JR, Kipnis J. Dealing with Danger in the CNS: The Response of the Immune System to Injury. Neuron 2015; 87:47-62; PMID:26139369; http://dx.doi.org/10.1016/j.neuron.2015.05.019
- Coussens LM, Werb Z. Inflammation and cancer. Nature 2002; 420:860-7; PMID:12490959; http://dx.doi.org/10.1038/nature01322
- Ming M, Obata F, Kuranaga E, Miura M. Persephone/Spatzle Pathogen Sensors Mediate the Activation of Toll Receptor Signaling in Response to Endogenous Danger Signals in Apoptosis-deficient Drosophila. J Biol Chem 2014; 289:7558-68; PMID:24492611; http://dx.doi.org/10.1074/jbc.M113.543884
- Welchman DP, Aksoy S, Jiggins F, Lemaitre B. Insect immunity: from pattern recognition to symbiont-mediated host defense. Cell host & microbe 2009; 6:107-14; PMID:19683677; http://dx.doi.org/10.1016/j.chom.2009.07.008
- Lee WJ, Miura M. Mechanisms of systemic wound response in Drosophila. Curr Top Dev Biol 2014; 108:153-83; PMID:24512709; http://dx.doi.org/10.1016/B978-0-12-391498-9.00001-2
- Takeishi A, Kuranaga E, Tonoki A, Misaki K, Yonemura S, Kanuka H, Miura M. Homeostatic Epithelial Renewal in the Gut Is Required for Dampening a Fatal Systemic Wound Response in Drosophila. Cell Rep 2013; 3:919-30; PMID:23523355; http://dx.doi.org/10.1016/j.celrep.2013.02.022
- Chakrabarti S, Dudzic JP, Li X, Collas EJ, Boquete JP, Lemaitre B. Remote Control of Intestinal Stem Cell Activity by Haemocytes in Drosophila. PLoS Genet 2016; 12:e1006089; PMID:27231872; http://dx.doi.org/10.1371/journal.pgen.1006089
- Nam HJ, Jang IH, You H, Lee KA, Lee WJ. Genetic evidence of a redox-dependent systemic wound response via Hayan Protease-Phenoloxidase system in Drosophila. Embo J 2012; 31:1253-65; PMID:22227521; http://dx.doi.org/10.1038/emboj.2011.476
- Pagliarini RA, Xu T. A genetic screen in Drosophila for metastatic behavior. Science 2003; 302:1227-31; PMID:14551319; http://dx.doi.org/10.1126/science.1088474
- Pastor-Pareja JC, Wu M, Xu T. An innate immune response of blood cells to tumors and tissue damage in Drosophila. Dis Model Mech 2008; 1:144-54; discussion 153; PMID:19048077; http://dx.doi.org/10.1242/dmm.000950
- Parisi F, Stefanatos RK, Strathdee K, Yu Y, Vidal M. Transformed epithelia trigger non-tissue-autonomous tumor suppressor response by adipocytes via activation of Toll and Eiger/TNF signaling. Cell Rep 2014; 6:855-67; PMID; http://dx.doi.org/10.1016/j.celrep.2014.01.039
- Cavuoto P, Fenech MF. A review of methionine dependency and the role of methionine restriction in cancer growth control and life-span extension. Cancer Treat Rev 2012; 38:726-36; PMID:22342103; http://dx.doi.org/10.1016/j.ctrv.2012.01.004
- Katzenberger RJ, Chtarbanova S, Rimkus SA, Fischer JA, Kaur G, Seppala JM, Swanson LC, Zajac JE, Ganetzky B, Wassarman DA. Death following traumatic brain injury in Drosophila is associated with intestinal barrier dysfunction. Elife 2015; 4: PMID:25742603; http://dx.doi.org/10.7554/eLife.04790
- Katzenberger RJ, Ganetzky B, Wassarman DA. The gut reaction to traumatic brain injury. Fly 2015; 9:68-74; PMID:26291482; http://dx.doi.org/10.1080/19336934.2015.1085623
- Dash PK, Hergenroeder GW, Jeter CB, Choi HA, Kobori N, Moore AN. Traumatic Brain Injury Alters Methionine Metabolism: Implications for Pathophysiology. Front Syst Neurosci 2016; 10:36; PMID:27199685; http://dx.doi.org/10.3389/fnsys.2016.00036
- Glanville NT, Anderson GH. Altered methionine metabolism in streptozotocin-diabetic rats. Diabetologia 1984; 27:468-71; PMID:6391990; http://www.ncbi.nlm.nih.gov/pubmed/6391990
- Brem H, Tomic-Canic M. Cellular and molecular basis of wound healing in diabetes. J Clin Invest 2007; 117:1219-22; PMID:17476353; http://dx.doi.org/10.1172/JCI32169
- Antoniades C, Shirodaria C, Warrick N, Cai S, de Bono J, Lee J, Leeson P, Neubauer S, Ratnatunga C, Pillai R, et al. Five-methyltetrahydrofolate rapidly improves endothelial function and decreases superoxide production in human vessels: effects on vascular tetrahydrobiopterin availability and endothelial nitric oxide synthase coupling. Circulation 2006; 114:1193-201; PMID:16940192; http://dx.doi.org/10.1161/CIRCULATIONAHA.106.612325
- Pereztamayo R, Ihnen M. The Effect of Methionine in Experimental Wound Healing - a Morphologic Study. Am J Pathol 1953; 29:233-49; PMID:13030690
- Sugiura T, Wang H, Barsacchi R, Simon A, Tanaka EM. MARCKS-like protein is an initiating molecule in axolotl appendage regeneration. Nature 2016; 531:237-40; PMID:26934225; http://dx.doi.org/10.1038/nature16974
- Hasegawa T, Nakajima T, Ishida T, Kudo A, Kawakami A. A diffusible signal derived from hematopoietic cells supports the survival and proliferation of regenerative cells during zebrafish fin fold regeneration. Dev Biol 2015; 399:80-90; PMID:25533245; http://dx.doi.org/10.1016/j.ydbio.2014.12.015
- Owusu-Ansah E, Perrimon N. Stress Signaling Between Organs in Metazoa. Annu Rev Cell Dev Bi 2015; 31:497-522; PMID:26393775; http://dx.doi.org/10.1146/annurev-cellbio-100814-125523