ABSTRACT
Electrospun nanofiber matrices sufficiently mimic the structural morphology of natural extracellular matrix. In this study, we aimed to examine the effects of agar/polyvinyl alcohol nanofiber (PVA) scaffold on the proliferation efficiency and differentiation potential of neonate mouse spermatogonial stem cells (SCCs). Testicular cells were isolated from testes of 40 mouse pups and were seeded in: 1) 2D cell culture plates in the absence (2D/−GF) or presence (2D/+GF) of growth factors and 2) onto agar/PVA scaffold in the absence (3D/−GF) or presence (3D/+GF) of growth factors. The cells were subsequently cultured for 4 weeks. First 2 weeks were dedicated to proliferative phase, whereas the next 2 weeks emphasized the differentiation phase. The identity of the SCCs was investigated at different time-points by flow cytometry and quantitative reverse transcription PCR (qRT-PCR) analyses against the germ cell markers, including PLZF, Id-4, Gfrα-1, Tekt-1, and Sycp-3. After 2 weeks of culture, the 3D/+GF group showed the highest percentage of PLZF-positive cells among culture systems (P < 0.05). The expression levels of pre-meiotic markers (Id-4 and Gfrα-1) decreased significantly in all groups, particularly in 3D/+GF group after 28 days of culture. Additionally, the cells in the 3D/+GF group displayed the highest expression of meiotic (Sycp-3) and post-meiotic markers (Tekt-1) 14 days after differentiation induction. Seemingly, the combination of the agar/PVA scaffold and growth factor-supplemented medium synergistically increased the differentiation rate of mouse SSCs into meiotic and post-meiotic cells. Thus, agar/PVA nanofiber scaffolds may have the potential for applications in the restoration of infertility, especially in azoospermic males.
Abbreviations
2D: two dimentional; 3D: three dimentional; bFGF: basic fibroblast growth factor; BMP-4: bone morphogenetic protein 4; DMEM: Dulbecco’s modified Eagle’s medium; ECM: extracellular matrix; FCS: fetal calf serum; FTIR: Fourier-transform infrared spectroscopy; GDNF: glial cell line-derived neurotrophic factor; GF: growth factors; Gfrα-1, GDNF family co-receptor α1; Id-4, Inhibitor of DNA Binding 4; MTT: methylthiazoltetrazolium; PLZF: promyelocytic leukemia zinc finger; PVA: polyvinyl alcohol; qRT-PCR: quantitative reverse transcription PCR; RA: retinoic acid; SACS: soft agar culture system; SD: standard deviation; SEM: scanning electron microscope; SSCs: spermatogonial stem cells; Sycp-3, Synaptonemal complex protein 3; Tekt-1, Tektin 1.
Introduction
Spermatogonial stem cells (SSCs), which are primitive diploid cells in the testes, have the potential for infinite self-renewal in the seminiferous tubules and generate differentiated germ cells that are committed to developing into sperm (Dym et al. Citation2009; Hermann et al. Citation2010; Yoshida Citation2010, Citation2012). Recent investigations have confirmed that neonatal SSCs possess pluripotency (Lim et al. Citation2013). Thus, these cells can efficaciously be used in fertility restoration (Hermann et al. Citation2012; de Michele et al. Citation2017), transgenic sperm production (Sato et al. Citation2013; Forbes et al. Citation2018) and cell-based organ regeneration therapy (Fagoonee et al. Citation2011; Barnes et al. Citation2016). SSCs of mammals lie within microenvironments, or niches, that are formed largely by the Sertoli cells, basement membrane, and seminiferous tubule interstitial cells (Shetty and Meistrich Citation2007; Khanehzad et al. Citation2016). While the somatic Sertoli cells provide the essential growth factors for the expansion of SSCs (e.g., basic fibroblast growth factor (bFGF) and glial cell line-derived neurotrophic factor (GDNF)) (Kubota et al. Citation2004b; Koruji et al. Citation2009), interstitial and peritubular cells supply directing signals that are necessary for the localization of undifferentiated spermatogonia (Yoshida et al. Citation2007). Sertoli cells are also allegedly involved in the regulation of SSC differentiation in murine juvenile testes by providing retinoic acid (RA) and bone morphogenetic protein 4 (BMP-4) to spermatogonia (Mark et al. Citation2008; Boyer et al. Citation2012). Meanwhile, the basement membrane, which is mainly comprised of collagen IV, laminin, entactin, and perlecan, provides for anchorage (Shams et al. Citation2017). All in all, the testicular niche plays imperative roles in the maintenance, proliferation, self-renewal, expansion, and differentiation of SSCs (Kostereva and Hofmann Citation2008).
Currently, male infertility is a global public health issue that affects approximately 7% of the male population worldwide (Miyamoto et al. Citation2015). A plethora of efforts have been made over the past decades attempting to generate functional spermatids especially for azoospermic individuals who have no options to conceive biological children. In vitro SSC culture has opened up a new effective strategy for treating infertility in azoospermic patients and restoring fertility in cancer survivors (Goossens et al. Citation2013; Picton et al. Citation2015). In order to provide an appropriate condition for the ex vivo proliferation of SSCs, it is essential to develop convenient cell culture systems that can effectively imitate not only the compositional and physiological properties but also the structural and architectural characteristics of the native SSC microenvironment (Dan et al. Citation2006; Shakeri et al. Citation2013). Indeed, recent investigations on the development of SSC culture systems have chiefly been focused on the preparation of new physicochemical (Lee et al. Citation2013; Antoni et al. Citation2015) and physiological (Kubota et al. Citation2004a; Gholami et al. Citation2018b) microenvironments that can adequately promote self-renewal and differentiation of stem cells. However, no satisfactorily efficient culture model has so far been identified by these studies (Park et al. Citation2017). Thus, there is an increasing interest for the application of three-dimensional (3D) cell culture systems mimicking the SSC niche (for review see (Shams et al. Citation2017)).
Nanofiber-based cell culture scaffolds sufficiently mimic the structural morphology and architecture of native extracellular matrix (ECM) and are therefore capable of recapitulating the intricacies of natural tissue microenvironment (Jiang et al. Citation2012). These scaffolds are known to offer more biomimicking topographical cues that facilitate cell attachment, ease cell spreading and promote cell proliferation and differentiation (Gholami et al. Citation2018a; Ganjibakhsh et al. Citation2019). In addition, the porous structure of 3D culture systems provides more space for seeded cells and allows cell reorientation and tissue remodeling (Vacanti and Langer Citation1999). Numerous electrospun nanofibrillar surfaces have been developed recently for culturing various types of stem cells (Nur et al. Citation2006; Li et al. Citation2015; Talebi et al. Citation2019). Polyvinyl alcohol (PVA) is considered to be one of the most desirable polymers for biomedical applications due to a variety of advantages, including low cost, biodegradability, biocompatibility, lack of carcinogenicity, and non-toxicity (Entezam et al. Citation2017). Since PVA also possesses an exceptional spinnability, it is continuously utilized as a co-blending polymer in electrospinning experiments to construct new 3D cell cultures (Greiner and Wendorff Citation2007; Sousa et al. Citation2015). Previous studies have shown that the electrospun PVA nanofibrous scaffold or PVA hydrogel provides an appropriate environment for the differentiation of various cell types such as adipose-derived stem cells (Enderami et al. Citation2018; Ojaghi et al. Citation2019), pre-osteoblastic cells (da Silva et al. Citation2019), human induced pluripotent stem cells (Muduli et al. Citation2017; Abazari et al. Citation2018), and embryonic stem cells (Muduli et al. Citation2017). Thus, it seems that PVA-based scaffold play a role during the differentiation. There are also studies evaluating the impact of 3D soft agar culture system (SACS) on the development of mouse testicular germ cells (Stukenborg et al. Citation2008; Mahmoud Citation2012; Eslahi et al. Citation2013). Recently, human testicular germ cells have been successfully induced to reach the final stages of spermatogenesis by embedding single-cell suspension from testicular tubules in soft agar (Mohammadzadeh et al. Citation2019). It has been claimed that soft agar culture system produces a microenvironment that resembles the natural niche of SSCs in the seminiferous tubules and therefore reduces the risk of ischemia in a long-term testicular tissue culture (Weaver et al. Citation1997; Zhang Citation2004). However, the application of soft agar matrix or PVA-based scaffold for in vitro cell culturing has its own drawbacks. Cultured cells in SACS can only be detected after removing the agar layer and transferring the cells to slides. This causes a serious problem for the application of SACS in assisted reproductive treatments (Abu Elhija et al. Citation2012). Besides, electrospinning of a pure agar solution is quite challenging, much due to the repulsive electrostatic interactions which exist between agar molecules. On one hand, this issue is usually circumvented by adding a co-blending polymer such as PVA (Sousa et al. Citation2015). On the other hand, neat PVA has a low mechanical stiffness (Azadian et al. Citation2019). Therefore, in order to improve its strength, PVA is often combined with other biocompatible materials and subjected to nano modifications in biomedicine (Enayati et al. Citation2018).
We hypothesized that the PVA-based scaffold can improve the differentiation capacity of neonate mouse spermatogonial stem-like cells into germ cell line. Therefore, in this study, we aimed to explore the combined effect of electrospun agar/PVA nanofibers and growth factor-supplemented medium on the differentiation of mouse SSCs.
Results
As shown in , animals were randomly allocated in this study into two major groups (i.e., 2D and 3D culture groups) of 20 mice each. Isolated testicular cells from each group were cultured in the absence (−GF) or presence (+GF) of growth factors (see materials and methods for details) ().
Figure 1. Study flow chart summarizing the overall protocol and different culture groups. bFGF, basic fibroblast growth factor; BMP-4, bone morphogenetic protein 4; Dif, designating the post-differentiation groups; GDNF, glial cell line-derived neurotrophic factor; GF, growth factors; RA, retinoic acid.
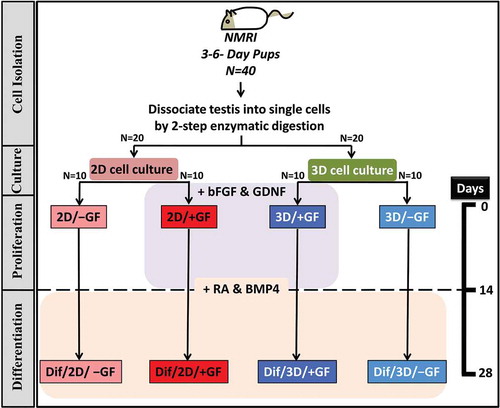
Characterization of the fabricated scaffold
The scanning electron microscope (SEM) images and Fourier-transform infrared spectroscopy (FTIR) spectra confirmed that electrospun scaffolds were successfully fabricated with 30:70 mass ratio of agar/PVA (Supplementary Figure S1 and S2). Fabricated nanofibers had an average diameter of 613.5 ± 139.0 nm with homogeneous and bead-free fiber morphology. These scaffolds comprised a uniform interwoven network, composed of a stacked web of randomly oriented nanofibers.
Cell viability
The cytocompatibility of agar/PVA 3D culture, as the experimental group, has been compared with that of 2D cell culture, as the control group, in . The viability of SSCs on 2D and 3D cultures was determined via MTT (methylthiazoltetrazolium) assay at 24 and 72 h after seeding. No significant difference was detected between 2D and 3D groups at either time points (P > 0.05 for both time points) in terms of MTT reducing activity ().
Flow cytometry
To evaluate the percentage of promyelocytic leukemia zinc finger (PLZF)-expressing cells, a flow cytometry analysis was carried out after two-step enzymatic digestion. According to our findings, 23.4% of all cells expressed PLZF after isolation. PLZF-expressing cells were also quantified after the emergence of spermatogonial stem-like cell colonies (i.e., after 24 h of cultivation) (), as well as at the end of 2 weeks of culture (). The relative frequency of PLZF-expressing cells increased significantly from 23.4% in freshly isolated testicular cells to 64.8% and 72.1% in 2D and 3D culture models, respectively (P < 0.001, for both), after the appearance of SSC colonies.
The percentage of PLZF-positive cells slightly increased thereafter and reached to more than 3- to 4-fold of freshly isolated cells at the end of the 14-day culture in all study groups (69.0% in 2D/−GF, 81.8% in 2D/+GF, 80.9% in 3D/−GF, and 93.6% in 3D/+GF). There were more PLZF-positive cells in 3D group than in 2D group after the emergence of colonies (P = 0.034) (). Flow cytometry analysis also revealed that the percentage of PLZF-positive cells was higher in 3D/+GF system in comparison to the 2D/+GF culture group 2 weeks post-seeding (P = 0.030) (). Similarly, in the absence of growth factors, 3D-cultured group (3D/−GF) had more PLZF-positive cells when compared with the 2D/−GF group, 2 weeks after cell seeding (P = 0.032) (). In addition, compared with growth factor-deprived cells, the percentage of PLZF-expressing cells was statistically higher among the growth factor-stimulated cells in both 2D and 3D culture models (P = 0.029 for 2D/+GF vs. 2D/–GF, and P = 0.018 for 3D/+GF vs. 3D/–GF) ().
Expression levels of pre-meiotic markers before differentiation induction
The capability of the agar/PVA 3D scaffold for the maintenance of undifferentiated mouse SSCs was explored by quantifying the expression levels of Id-4 (Inhibitor of DNA Binding 4) and Gfrα-1 (GDNF family co-receptor α1) which are self-renewal-related or pre-meiotic genes specific to undifferentiated pluripotent SSCs (Guo et al. Citation2014; Fayomi and Orwig Citation2018). After 2 weeks of culture, the expression of both pre-meiotic markers was up-regulated in almost all culture groups when compared with that of the freshly isolated testicular cells (,). The qRT-PCR analysis at the 14th day after seeding showed that the relative expression of Id-4 was statistically comparable between 3D and 2D cultures in the presence of growth factors (3D/+GF vs. 2D/+GF, P = 0.767) (). The expression level of Id-4 in unstimulated cells was also found to be statistically similar between 2D and 3D groups (3D/−GF vs. 2D/−GF, P = 0.903) (). Besides, cultured cells in 2D/+GF medium expressed ~3.8-fold more ID-4 mRNA than did cells obtained from 2D/–GF medium (P = 0.014). Furthermore, the ratio of ID-4 mRNA level in 3D/+GF group was about 6.2-fold of that in 3D/–GF group (P = 0.002) ().
Figure 4. Quantitative gene expression analysis by real-time PCR for (A) Id-4 and (B) Gfrα-1 genes in cultured mouse undifferentiated SSCs at the 14th day of culture. The expression levels of target genes in the experimental groups were investigated in relation to the expression rates of the same gene in the freshly isolated testicular cells. The experiments were replicated at least three times and expression levels were normalized according to housekeeping gene β-actin.*, **, and ***, respectively, denote significant differences at P < 0.05, P < 0.01 and P < 0.001 levels compared to the freshly isolated testicular cells. Data represent the mean (± SD) of 2−△△Ct.
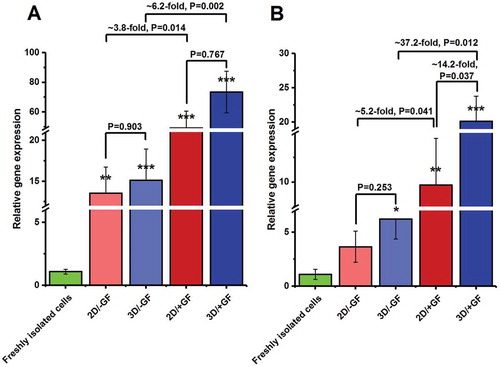
After 2 weeks of culture, the relative expression of Gfrα-1 was higher in the 3D/–GF group than in the 2D/–GF group; however, the difference was not significant (P = 0.253) (). On the contrary, Gfrα-1 was more frequently expressed (more than 14-fold) in 3D/+GF medium than in 2D/+GF (P = 0.037). GFRα-1 transcript also appeared to be more elevated in growth-factor primed media (i.e., 2D/+GF and 3D/+GF) as compared with the corresponding unsupplemented culture groups (i.e., 2D/–GF and 3D/–GF, respectively) ().
Expression levels of pre-meiotic, meiotic and post-meiotic markers after the differentiation induction
We next measured the expression levels of pre-meiotic (i.e., ID-4 and GFRα-1), meiotic (Synaptonemal complex protein 3, SYCP-3), and post-meiotic (i.e., Tektin 1, TEKT-1) markers in the cells that had been induced to differentiate by RA and BMP-4 (i.e., ‘Dif’ media) ( and ). As shown in , the expression of Id-4 was down-regulated ~2.8-fold, ~3.3-fold, ~22.3-fold, and ~40.3-fold in Dif/2D/–GF, Dif/3D/–GF, Dif/2D/+GF, and Dif/3D/+GF groups, respectively, when compared with the corresponding pre-differentiation groups (i.e., 2D/–GF, 3D/–GF, 2D/+GF, and 3D/+GF, respectively). There was no significant difference in terms of Id-4 gene expression between 2D and 3D cultures supplemented with or without growth factors after the differentiation induction (P = 0.073 for Dif/2D/–GF vs. Dif/3D/–GF, and P = 0.998 for Dif/2D/+GF vs. Dif/3D/+GF) (). However, the relative mRNA levels of ID-4 were found to be about ~75.4-fold lower in the Dif/2D/+GF group as compared to the Dif/2D/–GF group (P = 0.001). A comparable difference was detected between Dif/3D/–GF versus Dif/3D/+GF group. In fact, cells from Dif/3D/+GF group had approximately 69-fold lower levels of ID-4 transcript than cells from Dif/3D/–GF group (P = 0.018) ().
Figure 5. Relative gene expression profile of pre-meiotic markers, (A) Id-4 and (B) Gfrα-1, at the end of the experiment period. The expression levels of target genes (at the 28th day of culture) in each experimental group are presented in relation to the expression rates of the same gene in the same group before differentiation induction (i.e., at the 14th day of culture). The experiments were replicated at least three times and expression levels were normalized according to housekeeping gene β-actin. Data represent the mean ± SD.
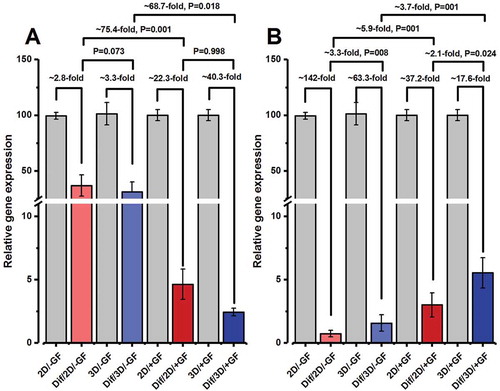
Figure 6. Relative gene expression profile of meiotic and post-meiotic markers, (A) Sycp-3 and (B) Tekt-1, at the end of the experiment period (i.e., at the end of 4 weeks of culture). The expression levels of target genes in different experimental groups were investigated in relation to the expression rate of the same gene in the Dif/2D/–GF group. The experiments were replicated at least three times and expression levels were normalized according to housekeeping gene β-actin. *, **, and ***, respectively, denote significant differences at P < 0.05, P < 0.01 and P < 0.001 levels compared to the Dif/2D/–GF group. Data represent the mean (± SD) of 2−△△Ct.
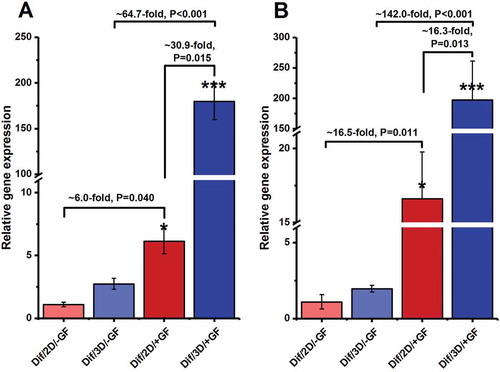
Like Id-4, significant decreases in the expression of Gfrα-1were detected in all media groups after 2 weeks of culture under differentiation condition (i.e., at 28th day of culture), as compared with before the addition of differentiating factors (i.e., at 14th day of culture) (paired t-test P < 0.05 for all comparisons) (). Among the differentiated groups and at the end of the 4th week of cultivation, the expression of Gfrα-1 gene was statistically higher in both Dif/2D/+GF and Dif/3D/+GF groups when compared with their corresponding un-supplemented media (i.e., Dif/2D/–GF and Dif/3D/–GF, respectively) (). The most pronounced elevation was observed in 3D-cultured cells that had been stimulated by growth factors (Dif/3D/+GF group) during the pre-differentiation phase of the experiment. In fact, a more than 2-fold elevation was observed in the mRNA level of GFRα-1 in the Dif/3D/+GF group compared to the Dif/2D/+GF group (P = 0.024) ().
Similar trends were also seen in the gene expression levels of meiotic (SYCP-3) and post-meiotic markers (TEKT-1) (,). Growth factor-stimulated cells, irrespective of being cultured in 2D or 3D medium, showed higher relative expression of meiotic and post-meiotic genes than growth factor-deprived cells after 2 weeks of culture in the presence of RA and BMP-4 (P < 0.05 for all comparisons). The highest expression levels for genes coding SYCP-3 and TEKT-1 were observed in Dif/3D/+GF group (,).
Discussion
SSCs are undifferentiated spermatogonia that not only preserve their self-renewal potential but also maintain their capacity to differentiate into committed germ cells (Kanatsu-Shinohara and Shinohara Citation2013). These features guarantee the stem cell pool maintenance on the one hand, and fulfill the proliferative demand of the male reproductive gonads to generate large quantities of sperms per ejaculate, on the other. Therefore, SSCs are regarded as an attractive model to identify the molecular mechanisms involved in the regulation of self-renewal and differentiation of stem cells (Guo et al. Citation2014). However, SSCs comprise only 0.02–0.03% of total germ cells in rodent testes and no exclusive surface marker uniquely specific for these cells has been identified so far (Tegelenbosch and de Rooij Citation1993). In fact, the only decisive way to identify an SSC is by observing its capability to maintain spermatogenesis homeostasis using transplantation or lineage tracing (Fayomi and Orwig Citation2018). Accordingly, developing efficient culture systems that can preserve the self-renewal capacity of SSCs and facilitate their differentiation has attracted broad attention within the stem cell research community. In the present study, we aimed to examine the impact of an electrospun 3D nanofibrous scaffold on mouse SSC fate under self-renewing or differentiating condition. Overall, the results obtained here demonstrated a promising performance for agar/PVA nanofiber scaffold in supporting SSC survival, promoting cell proliferation, maintaining self-renewal potential and assisting the differentiation of mouse spermatogonial stem-like cells towards more differentiated cell lineages.
Since spermatogenesis begins shortly after birth in rodents, the testicular cells of 3–6-day-old mice were used in this research work. During this period, a population of undifferentiated spermatogonial stem cells arises from a subset of gonocytes which are located within the center of seminiferous tubules (Drumond et al. Citation2011). These undifferentiated cells are called type Asingle spermatogonia and have classically been considered as the SSC population (Ehmcke et al. Citation2006). Mitotic division of Asingle produces committed progenitor spermatogonia (i.e., Apaired and Aaligned) which, along with Asingle cells, are collectively regarded as undifferentiated A-spermatogonia according to morphological characteristics (Clermont and Bustos-Obregon Citation1968; Fayomi and Orwig Citation2018). Further mitotic divisions of undifferentiated A-spermatogonia generate a series of differentiating spermatogonia, including A1, A2, A3, A4, intermediate, and B spermatogonia. Eventually, type B spermatogonia differentiate into primary spermatocytes that will undergo two meiotic divisions to generate round spermatids. Morphological differentiation (spermiogenesis) of these haploid cells leads to the development of mature sperms.
In the current investigation, enzymatic digestion was used to isolate mouse neonatal testicular cells (Azizollahi et al. Citation2016; Shabani et al. Citation2016). In order to evaluate the combined effect of agar/PVA nanofiber scaffold and growth factors on the SSCs, we cultured mouse testicular cells in the presence and absence of GDNF/bFGF. GDNF is distinguished as the most crucial factor in SSC proliferation (Hofmann Citation2008), survival (Oatley et al. Citation2007), and self-renewal (Oatley et al. Citation2006). This paracrine factor, secreted mostly by Sertoli cells, seems to induce the proliferation of SSCs by binding to their GFRα-1/RET receptors (Iwamori Citation2014). bFGF is also known to stimulate self-renewal of undifferentiated SSCs through mitogen-activated protein kinase (MAPK) signaling pathways (Simon et al. Citation2007). Cell viability analysis performed after 24 and 72 h post-seeding failed to reveal any significant difference between 2D and 3D culture systems that had been supplemented with growth factors.
The percentage of SSCs was determined by a marker of undifferentiated spermatogonia, PLZF, at three time points: at baseline (i.e., at the end of the isolation process), after the emergence of spermatogonial stem-like cell colonies (i.e., after 24 h of cultivation), and after 2 weeks of culture. PLZF (also called ZBTB16 or ZFP145) is a transcriptional regulator that inhibits stem cell differentiation and is expressed in undifferentiated spermatogonia (i.e., Asingle, Apaired, and Aaligned) (Aponte et al. Citation2005). The percentage of PLZF-positive cells increased in all groups over time, indicating that the number of undifferentiated spermatogonia is increased in all culture systems, albeit to a different extent. This increasing trend was more pronounced in 3D group than in 2D group. Among the 3D-cultured cells, growth factor-primed group (3D/+GF) showed a higher percentage of PLZF-positive cells than the growth factor-deprived medium (3D/−GF) at both follow-up times. It seems that the combination of agar/PVA scaffold and growth factor-supplemented medium can enhance the proliferation rate of SSCs and progenitor cells more efficiently than other culture systems.
Although no specific biochemical marker has been identified for SSCs, monitoring the expression of genes with a restricted pattern of expression can provide critical information about spermatogonial cell types. Multiple molecular markers have been identified for undifferentiated and differentiated spermatogonia. qRT-PCR analysis was undertaken here to explore the expression pattern of Id-4 and Gfrα-1 at three time-points: before seeding (i.e., in freshly isolated testicular cells), at the end of 2 weeks of culture, and after 4 weeks of culture. While ID-4 appears to have a restricted pattern of expression in Asingle spermatogonia (Oatley et al. Citation2011), GFRα-1 has expression limited to Asingle, Apaired, and short chains (i.e., four cell cohorts) of Aaligned spermatogonia (Meng et al. Citation2000). qRT-PCR findings revealed that the growth factor-stimulated cells in both culture models (2D or 3D) had higher mRNA expression of ID-4 and GFRα-1, relative to their unstimulated counterparts at the end of the second week. This is in line with the results of previous research works, which showed that the expression levels of Id-4and Gfrα-1 are up-regulated by GDNF signaling (Oatley et al. Citation2009; Grasso et al. Citation2012; Helsel et al. Citation2017). ID-4 is a transcription repressor that plays a critical role in the regulation of balance between self-renewal and differentiation of SSCs. In vitro down-regulation of ID-4 contributes to proliferation inhibition of SSCs and leads to progressive germ cell depletion in mice (Oatley et al. Citation2011). Our data also demonstrated that the ID-4 transcript level was statistically similar between 2D and 3D cultures supplemented with growth factors after 2 weeks of culture. On the other hand, Gfrα-1 expression rate was found to be statistically elevated in the 3D/+GF medium as compared with the 2D/+GF medium after 14 days of cultivation. A similar trend was also detected for the percentage of PLZF-expressing cells. Based on these findings, it seems that the developmental path of undifferentiated spermatogonia is going to be shifted towards the committed progenitor cells and late undifferentiated spermatogonia in 3D/+GF system after 2 weeks of culture.
To induce the differentiation in mouse SSC differentiation, RA and BMP-4 were added to the cultured cells. The BMP-4 is known to suppress the expression of somatic genes and thus prompts the up-regulation of differentiation genes in the germ cells (Hayashi et al. Citation2018). In addition, RA is believed to stimulate meiosis mainly through stem cell factor (SCF)/c-Kit signaling pathway (Li et al. Citation2014). Compared with the pre-differentiation media, the expression levels of Id-4 and Gfrα-1 genes dropped significantly in all culture systems 14 days after the differentiation induction (at the 28th day of the experiment). Id-4 expression reduction was more pronounced in growth factor-stimulated cells, particularly in 3D culture model. In fact, ID-4 mRNA level showed a more than 40-fold reduction in the Dif/3D/+GF system compared to the 3D/+GF system. In comparison, Gfrα-1 expression reduction was more pronounced in growth factor-deprived cells at the end of the culture period. The relative expression of Gfrα-1 was about two-fold higher in Dif/3D/+GF group when compared with Dif/2D/+GF system. Taken together, it seems that SSC differentiation is favored over proliferation when the testicular cells are cultured in the presence of growth factors, particularly in 3D culture medium.
SYCP-3 is a meiotic marker whereas TEKT-1 is a post-meiotic male germ cell marker. SYCP-3 is required for synaptonemal complex assembly and is expressed during meiotic prophase I in spermatocytes from the leptotene to the pachytene stage (Izadyar et al. Citation2003; Bisig et al. Citation2012; Azizollahi et al. Citation2016). TEKT-1 is assumed to be involved in the development of the sperm axoneme and flagellum. In mice, Tekt-1 expression begins around the pachytene stage of meiosis and continues up until the haploid round spermatid stage (Larsson et al. Citation2000; Yamauchi et al. Citation2009). Two weeks after differentiation induction (i.e., at the 28th day of the experiment), the expression levels of both of these markers were drastically higher in growth factor-stimulated cells when compared to growth factor-deprived cells. Cells from the Dif/3D/+GF medium displayed the highest relative expression of both Sycp-3 and Tekt-1 genes. These findings further indicate that our 3D/+GF system efficiently promotes the differentiation of mouse SSCs into spermatids.
The exact molecular mechanism by which agar/PVA scaffold promotes mouse SSC differentiation was not investigated in the current research work. Nanofiber culture systems have shown encouraging results in differentiating germ cells (Shams et al. Citation2017). This capability has been attributed mainly to high surface-to-volume ratio of nanofiber scaffolds, which offers large surface area, allows an improved cell adhesion, enhances the number of cells in the culture medium, promotes inter-cellular communication, facilitates mass transport, and boosts paracrine crosstalk between cells (Mahmoud Citation2012; Antoni et al. Citation2015; Sankar et al. Citation2017; Shams et al. Citation2017). Other properties of nanostructured scaffolds, such as stiffness, topography and chemistry, are also believed to be important in contributing to the effectiveness of 3D nanofiber scaffolds as a proper supporting material for in vitro stem cell culture (Higuchi et al. Citation2013; Donnelly et al. Citation2018).
As a type of biodegradable and non-toxic polymer with high water solubility, PVA has been approved by U.S. Food and Drug Administration (FDA) for medical use (Gaaz et al. Citation2015). PVA has been used, as hydrogel or nanofibrous scaffold, for culturing stem cells in vitro. However, the inherent property of PVA has hindered its application in stem cell culture. Previous studies have shown that stem cells cultured in PVA hydrogel cannot proliferate efficiently and secret sufficient levels of growth factors due to the incapability of forming stable focal adhesions (Gao et al. Citation2019). As indicated previously, because of the low rigidity of PVA, it is commonly electrospun with other polymers for use in biomedical purposes. Electrospinning is indeed a versatile process for producing the desired polymeric scaffolds with required morphology and porosity, and resulting electrospun nanofibers have multiple advantages such as improved cell adhesion, proliferation, differentiation, and migration (Greiner and Wendorff Citation2007; Sankar et al. Citation2017; Shams et al. Citation2017). Agar/PVA combination was used in this study to fabricate a porous nanofiber scaffold according to previously reported method (Sousa et al. Citation2015). Agar is a biocompatible, complex polysaccharide extracted from red sea weeds and was first used by Lin and colleagues (Lin et al. Citation1975) to establish a 3D cell culture model (i.e., SACS) for characterizing clonal expansion of bone marrow cells. Since then several research groups have utilized SACS to investigate proliferation and differentiation of male germ cells in vitro (Stukenborg et al. Citation2008, Citation2009; Abu Elhija et al. Citation2012; Reda et al. Citation2016; Navid et al. Citation2017; Gholami et al. Citation2018a, Citation2018b; Mohammadzadeh et al. Citation2019). Application of SACS for in vitro spermatogenesis, however, was not devoid of pitfalls. A considerable proportion of murine SSCs (40–70%) was found to be lost from the upper gel phase of SACS during the initial 24 h of culture (Stukenborg et al. Citation2009), probably due to the loss of cellular interaction and subsequent triggering of apoptotic cascades (Gholami et al. Citation2018b). SACS also demonstrated an extremely low performance in promoting the differentiation of rat germ cells (Reda et al. Citation2014). Furthermore, the presence of somatic cells (such as Leydig cells and Sertoli cells) in the upper layer of SACS seems to be critical for efficient proliferation of stem cells (Sato et al. Citation2012). There is even evidence suggesting that human spermatogenic cells are unable to efficiently complete the full period of spermatogenesis in soft agar matrix (Gholami et al. Citation2018b).
Agar/PVA scaffold offers two major advantages over the PVA hydrogel or SACS. First, unlike the PVA-based hydrogel culture system or SACS, cells efficiently proliferate after seeding on the agar/PVA scaffold and no major cell loss was noted during the initial stages of culture (i.e., from 24 to 72 h of culture). Second, in contrast to soft agar culture model, the viability of spermatogenic cells in our 3D culture is not dependent on Sertoli cells.
These early data suggest that mouse SSCs are preferentially differentiated into meiotic and post-meiotic cells after 4 weeks of culture in the presence of agar/PVA nanofiber scaffold and growth factors. 3D nanofibrous-based scaffolds create a high surface area to volume ratio and therefore simulate a testis-like niche in which homing and differentiation are promoted. More investigations, however, are needed to validate our results. Particularly that, the majority of conclusions speculated here are mostly based on the expression pattern of pre-meiotic, meiotic and post-meiotic markers at the mRNA level. The expression levels of additional meiotic and post-meiotic markers should be quantified, both at mRNA and protein levels, to confirm the findings of the present research project. Moreover, the differentiation of spermatogonial stem-like cells has to be confirmed by further experiments (e.g., morphology and immunohistochemistry data). Besides, the safety and functionality of the developed sperm-like cells have to be thoroughly examined by further studies. Ultimately, our findings can be considered as the stepping stone for the development of stem cell-based treatment strategies for male infertility in patients with azoospermia.
Material and methods
Fabrication of electrospun agar/PVA nanofibers
The electrospun agar/PVA nanofibers were prepared according to the procedure of Sousa et al. (Sousa et al. Citation2015) (see Supplementary information for details). The structure and morphology of nanofibers were examined with an SEM. FTIR was used to analyze the compositions of electrospun scaffolds (see Supplementary information).
Isolation of murine spermatogonial stem cells
The testes of mouse pups were collected for the preparation of cell suspension as described previously (Eslahi et al. Citation2013). In brief, the testes were decapsulated, cut into small pieces and finally suspended in Dulbecco’s modified Eagle’s medium (DMEM; Gibco Invitrogen, Carlsbad, CA, USA), which had been supplemented with 1.37 g/L sodium bicarbonate (NaHCO3; Merck, Darmstadt, Germany), single-strength nonessential amino acids (Sigma-Aldrich), gentamycin (40 µg/mL), streptomycin (100 µg/m), and penicillin (100 IU/mL) (all from Life Technologies, Inc., Grand Island, NY, USA).
Testicular cells were separated according to van Pelt et al.’s method with slight modifications (van Pelt et al. Citation1996). Briefly, the minced testicular tissues were suspended in DMEM containing 0.5 mg/mL Trypsin (Sigma-Aldrich), 0.5 mg/mL collagenase/dispase (Roche, Basel, Switzerland), and 0.05 mg/mL DNAse (Roche), and incubated at 37°C for 30 min (with gently shaking and periodical pipetting). Interstitial cells were subsequently removed by centrifugation at 1200 rpm for 1 min. In order to isolate the single cells, a second enzymatic digestion was achieved in DMEM by adding a fresh enzyme solution (0.5 mg/mL Trypsin, 0.5 mg/mL collagenase/dispase, and 0.05 mg/mL DNAse) into the seminiferous cord fragments under the same condition. An additional centrifugation step (at 400 rpm for 1 min) was performed to eliminate undigested pieces. The resulting suspension mainly contained spermatogonial cells, Sertoli cells, and residual interstitial cells. The cell suspension was then filtered through a 70-µm nylon filter (Becton, NJ, USA) to remove cell clumps. In the next step, the spermatogonia were further enriched by overnight differential plating in DMEM containing 5% (v/v) fetal calf serum (FCS; Gibco Invitrogen). After 24 h of incubation, Sertoli and myoid cells attached to culture dish, whereas spermatogonial cells remained in the suspension. Fresh SSCs were finally collected and seeded in 2D or 3D (agar/PVA) culture condition () (see below for details).
Spermatogonial cell culture
In order to culture SSC, fresh testicular cells (~5 × 105 cells) were seeded with (3D or experimental group) or without (2D or control group) the nanofiber scaffold after the two-step enzymatic digestion. In the case of 3D group, a layer of agar/PVA nanofiber was placed on the dishes and testicular cells were cultured onto nanofibrillar surfaces for 2 weeks in DMEM containing 5% (v/v) FCS with or without growth factors (i.e., 10 ng/mL bFGF (Sigma-Aldrich) and 10 ng/mL GDNF (Invitrogen, Baltimore, MD, USA)). The same cell culture dishes albeit with no agar/PVA nanofiber scaffold were used as the 2D or control group. The cells were incubated at 32°C in a humidified atmosphere of 5% CO2 in air. The medium was refreshed every ~48 h.
The identity of the cultured cells was further investigated by the flow cytometry analysis and quantitative expression assessment of spermatogonial pre-meiotic markers at mRNA levels (vide infra).
In vitro differentiation of spermatogonial stem cells
In order to evaluate the effect of nanofiber scaffolds on the differentiation of SSCs, cultured testicular cells were induced to differentiate by RA and BMP-4 in the presence (3D group) and absence (2D or control group) of agar/PVA nanofibers. For the induction of differentiation of the SSCs, 1 μM RA (Sigma-Aldrich) and 50 ng/ml BMP4 (Sigma-Aldrich) were added to cultured testicular cells. The medium was changed every 2 days.
The identity of the cultured cells was finally determined by quantitative expression analysis of spermatogonial pre-meiotic, meiotic and post-meiotic markers at mRNA level (vide infra). The overall protocol of the investigation is depicted in the study flow chart in .
Quantitative reverse transcription polymerase chain reaction (qRT-PCR)
Total RNA was extracted from the cultured cells (~1 × 105 cells) at different time points of cultivation using a standard RNA extraction kit (Qiagen, Hilden, Germany). Complementary DNA (cDNA) was then synthesized from ~1 μg of extracted RNA by QuantiTect Reverse Transcription Kit (Qiagen) according to the manufacturer’s instructions. Subsequently, qRT-PCR was carried out using SYBR Premix Ex TaqII (TliRNaseH Plus, RR820A; Takara) on an iCycler apparatus (Bio-Rad, Hercules, CA, USA). All primer sequences () were designed using Oligo software (ver. 4.0, National Biosciences Inc., Plymouth, MN). The amplification protocol was started by an initial denaturation step of 95°C for 5 min. This stage was followed by 40 cycles of melting (30 s at 95°C), specific annealing (40 s at 55°C), and extension (30 s at 72°C). The expression of β-actin, a well-known housekeeping gene, was also measured as an internal control to normalize for differences in sample concentration. The relative mRNA quantification of each specific gene was calculated by using the comparative 2–ΔΔCt method. Three replicate analyses were carried out for each culture group. In addition, all PCR reactions were performed at least in triplicate.
Table 1. The sequence of the designed primers used for qRT-PCR.
Flow cytometry analysis
The identity of the isolated SSCs was explored by flow cytometry method using a PLZF antibody. Retrieved cells (~2 × 105 cells) from 2D or 3D cell cultures were initially fixed in 4% paraformaldehyde (Merck) in phosphate-buffered saline (PBS; Invitrogen) (pH 7.4) for 20 min. Cells were then permeabilized in 0.3% Triton X-100 (Merck) in PBS for 30 min prior to blocking in 10% goat serum (Sigma-Aldrich) in PBS for half an hour. The samples were subsequently incubated with primary antibody solution (1:100, anti-PLZF antibody; Sigma-Aldrich) overnight at 4°C. Cells were washed twice with PBS in the following day and incubated with the secondary antibody (goat anti-mouse IgG-FITC (fluorescein isothiocyanate); 1:200; Sigma-Aldrich) for 1 h. Flow cytometric analysis was eventually conducted with a BD FACSCalibur flow cytometer (BD Biosciences, Sydney, Australia). The experiments were performed three times and the acquired data were analyzed using WinMDI software (ver. 2.9, TSRI, La Jolla, CA, USA).
MTT analysis
The viability of testicular cells, which were grown in 2D or 3D cell cultures, was assessed using MTT (methylthiazoltetrazolium, Sigma-Aldrich) assay at different time intervals of incubation period. For this purpose, 300 μl of the cell cultures (containing a density of ~30 × 103 cells) were transferred into a 24-well culture plate and treated with 100 µL MTT solution for 3 h at 37°C. In the next step, the medium was replaced with 1 ml dimethylsulfoxide (DMSO; 1.4 M; Merck), and the cells were incubated again at 37°C for another 20 min. Finally, the optical density (OD) of each well was read at 570 nm by a microplate reader (Bio-Rad Hercules, CA, USA) 24 h and 72 h after seeding. Three replicate analyses were performed for each culture system. All measurements were expressed as mean ± standard deviation (SD).
Statistical analysis
All statistical analyses were performed using Origin 8.0 (Origin Lab, MA, USA). The results were expressed as mean ± SD. All experiments were performed at least three times. The Student t-test, paired t-test and analysis of variance (ANOVA) (followed by Tukey post-hoc tests) were utilized to determine the statistical significance of the data. The results were assumed significant when P ˂ 0.05.
Ethics statement
Study animals were handled in accordance with the Animal Care and Use Guideline of Iran University of Medical Sciences. Male mouse pups (NMRI strain, 3–6-day-old, N=40) were purchased from the animal laboratory of Iran University of Medical Sciences (Tehran, Iran).
Authors’ contributions
Conception and design of the study, analyzed data, obtained funding, and supervised the project: FM, MK; Conducted SEM analysis and participated in the design and preparation of nanofibers: ZB; Took specimens, performed qRT-PCR and flow cytometry analyses, contributed in data interpretation, and wrote the first draft: MZK; Interpreted flow cytometry and qRT-PCR data and helped in manuscript evaluation: HRA, MN. All authors read and revised the manuscript. Additionally, all authors confirmed the final version of the manuscript to be submitted.
Supplemental Material
Download MS Word (692.2 KB)Acknowledgments
This study was financially supported by the Deputy of Research of Iran University of Medical Sciences (IUMS), Tehran, Iran (grant number 30476).
Disclosure statement
No potential conflict of interest was reported by the authors.
Supplementary material
Supplemental data for this article can be accessed here.
Additional information
Funding
References
- Abazari MF, Soleimanifar F, Nouri Aleagha M, Torabinejad S, Nasiri N, Khamisipour G, Amini Mahabadi J, Mahboudi H, Enderami SE, Saburi E, et al. 2018. PCL/PVA nanofibrous scaffold improve insulin-producing cells generation from human induced pluripotent stem cells. Gene. 671:50–57. Eng.
- Abu Elhija M, Lunenfeld E, Schlatt S, Huleihel M. 2012. Differentiation of murine male germ cells to spermatozoa in a soft agar culture system. Asian J Androl. 14(2):285–293. eng.
- Antoni D, Burckel H, Josset E, Noel G. 2015. Three-dimensional cell culture: a breakthrough in vivo. Int J Mol Sci. 16(3):5517–5527. Eng.
- Aponte PM, van Bragt MP, de Rooij DG, van Pelt AM. 2005. Spermatogonial stem cells: characteristics and experimental possibilities. APMIS. 113(11–12):727–742. Eng.
- Azadian E, Arjmand B, Ardeshirylajimi A, Hosseinzadeh S, Omidi M, Khojasteh A. 2019. Polyvinyl alcohol modified polyvinylidene fluoride-graphene oxide scaffold promotes osteogenic differentiation potential of human induced pluripotent stem cells. J Cell Biochem. Eng. 1–12
- Azizollahi S, Aflatoonian R, Sadighi Gilani MA, Behnam B, Tajik N, Asghari-Jafarabadi M, Asgari HR, Koruji M. 2016. Alteration of spermatogenesis following spermatogonial stem cells transplantation in testicular torsion-detorsion mice. J Assist Reprod Genet. 33(6):771–781.
- Barnes CJ, Distaso CT, Spitz KM, Verdun VA, Haramati A. 2016. Comparison of stem cell therapies for acute kidney injury. Am J Stem Cells. 5(1):1–10. Eng.
- Bisig CG, Guiraldelli MF, Kouznetsova A, Scherthan H, Hoog C, Dawson DS, Pezza RJ. 2012. Synaptonemal complex components persist at centromeres and are required for homologous centromere pairing in mouse spermatocytes. PLoS Genet. 8(6):e1002701. Eng.
- Boyer A, Yeh JR, Zhang X, Paquet M, Gaudin A, Nagano MC, Boerboom D. 2012. CTNNB1 signaling in sertoli cells downregulates spermatogonial stem cell activity via WNT4. PLoS One. 7(1):e29764. Eng.
- Clermont Y, Bustos-Obregon E. 1968. Re-examination of spermatogonial renewal in the rat by means of seminiferous tubules mounted “in toto”. Am J Anat. 122(2):237–247. eng.
- da Silva TN, Goncalves RP, Rocha CL, Archanjo BS, Barboza CAG, Pierre MBR, Reynaud F, de Souza Picciani PH. 2019. Controlling burst effect with PLA/PVA coaxial electrospun scaffolds loaded with BMP-2 for bone guided regeneration. Mater Sci Eng C Mater Biol Appl. 97:602–612. Eng.
- Dan YY, Riehle KJ, Lazaro C, Teoh N, Haque J, Campbell JS, Fausto N. 2006. Isolation of multipotent progenitor cells from human fetal liver capable of differentiating into liver and mesenchymal lineages. Proc Natl Acad Sci U S A. 103(26):9912–9917. Eng.
- de Michele F, Vermeulen M, Wyns C. 2017. Fertility restoration with spermatogonial stem cells. Curr Opin Endocrinol Diabetes Obes. 24(6):424–431. Eng.
- Donnelly H, Salmeron-Sanchez M, Dalby MJ. 2018. Designing stem cell niches for differentiation and self-renewal. J R Soc Interface. 15(145):20180388.
- Drumond AL, Meistrich ML, Chiarini-Garcia H. 2011. Spermatogonial morphology and kinetics during testis development in mice: a high-resolution light microscopy approach. Reproduction. 142(1):145–155. Eng.
- Dym M, Kokkinaki M, He Z. 2009. Spermatogonial stem cells: mouse and human comparisons. Birth Defects Res C Embryo Today. 87(1):27–34. Eng.
- Ehmcke J, Wistuba J, Schlatt S. 2006. Spermatogonial stem cells: questions, models and perspectives. Hum Reprod Update. 12(3):275–282. Eng.
- Enayati MS, Behzad T, Sajkiewicz P, Rafienia M, Bagheri R, Ghasemi-Mobarakeh L, Kolbuk D, Pahlevanneshan Z, Bonakdar SH. 2018. Development of electrospun poly (vinyl alcohol)-based bionanocomposite scaffolds for bone tissue engineering. J Biomed Mater Res A. 106(4):1111–1120. Eng.
- Enderami SE, Soleimani M, Mortazavi Y, Nadri S, Salimi A. 2018. Generation of insulin-producing cells from human adipose-derived mesenchymal stem cells on PVA scaffold by optimized differentiation protocol. J Cell Physiol. 233(5):4327–4337. Eng.
- Entezam M, Daneshian H, Nasirizadeh N, Khonakdar HA, Jafari SH. 2017. Hybrid hydrogels based on Poly (vinyl alcohol)(PVA)/Agar/Poly (ethylene glycol)(PEG) prepared by high energy electron beam irradiation: investigation of physico‐mechanical and rheological properties. Macromol Mater Eng. 302(2):1600397.
- Eslahi N, Hadjighassem MR, Joghataei MT, Mirzapour T, Bakhtiyari M, Shakeri M, Pirhajati V, Shirinbayan P, Koruji M. 2013. The effects of poly L-lactic acid nanofiber scaffold on mouse spermatogonial stem cell culture. Int J Nanomed. 8:4563–4576.
- Fagoonee S, Pellicano R, Silengo L, Altruda F. 2011. Potential applications of germline cell-derived pluripotent stem cells in organ regeneration. Organogenesis. 7(2):116–122. Eng.
- Fayomi AP, Orwig KE. 2018. Spermatogonial stem cells and spermatogenesis in mice, monkeys and men. Stem Cell Res. 29:207–214.
- Forbes CM, Flannigan R, Schlegel PN. 2018. Spermatogonial stem cell transplantation and male infertility: current status and future directions. Arab J Urol. 16(1):171–180. Eng.
- Gaaz TS, Sulong AB, Akhtar MN, Kadhum AA, Mohamad AB, Al-Amiery AA. 2015. Properties and applications of polyvinyl alcohol, halloysite nanotubes and their nanocomposites. Molecules. 20(12):22833–22847. Eng.
- Ganjibakhsh M, Mehraein F, Koruji M, Aflatoonian R, Farzaneh P. 2019. Three-dimensional decellularized amnion membrane scaffold as a novel tool for cancer research; cell behavior, drug resistance and cancer stem cell content. Mater Sci Eng C Mater Biol Appl. 100:330–340.
- Gao T, Jiang M, Liu X, You G, Wang W, Sun Z, Ma A, Chen J. 2019. Patterned polyvinyl alcohol hydrogel dressings with stem cells seeded for wound healing. Polymers (Basel). 11(1):171. Eng.
- Gholami K, Pourmand G, Koruji M, Ashouri S, Abbasi M. 2018a. Organ culture of seminiferous tubules using a modified soft agar culture system. Stem Cell Res Ther. 9(1):249.
- Gholami K, Pourmand G, Koruji M, Sadighigilani M, Navid S, Izadyar F, Abbasi M. 2018b. Efficiency of colony formation and differentiation of human spermatogenic cells in two different culture systems. Reprod Biol. 18(4):397–403.
- Goossens E, Van Saen D, Tournaye H. 2013. Spermatogonial stem cell preservation and transplantation: from research to clinic. Hum Reprod. 28(4):897–907. Eng.
- Grasso M, Fuso A, Dovere L, de Rooij DG, Stefanini M, Boitani C, Vicini E. 2012. Distribution of GFRA1-expressing spermatogonia in adult mouse testis. Reproduction. 143(3):325–332. Eng.
- Greiner A, Wendorff JH. 2007. Electrospinning: a fascinating method for the preparation of ultrathin fibers. Angew Chem Int Ed Engl. 46(30):5670–5703. Eng.
- Guo Y, Hai Y, Gong Y, Li Z, He Z. 2014. Characterization, isolation, and culture of mouse and human spermatogonial stem cells. J Cell Physiol. 229(4):407–413. Eng.
- Hayashi M, Kawaguchi T, Durcova-Hills G, Imai H. 2018. Generation of germ cells from pluripotent stem cells in mammals. Reprod Med Biol. 17(2):107–114. Eng.
- Helsel AR, Yang QE, Oatley MJ, Lord T, Sablitzky F, Oatley JM. 2017. ID4 levels dictate the stem cell state in mouse spermatogonia. Development. 144(4):624–634. Eng.
- Hermann BP, Sukhwani M, Hansel MC, Orwig KE. 2010. Spermatogonial stem cells in higher primates: are there differences from those in rodents? Reproduction. 139(3):479–493. Eng.
- Hermann BP, Sukhwani M, Winkler F, Pascarella JN, Peters KA, Sheng Y, Valli H, Rodriguez M, Ezzelarab M, Dargo G, et al. 2012. Spermatogonial stem cell transplantation into rhesus testes regenerates spermatogenesis producing functional sperm. Cell Stem Cell. 11(5):715–726. Eng
- Higuchi A, Ling Q-D, Chang Y, Hsu S-T, Umezawa A. 2013. Physical cues of biomaterials guide stem cell differentiation fate. Chem Rev. 113(5):3297–3328.
- Hofmann MC. 2008. Gdnf signaling pathways within the mammalian spermatogonial stem cell niche. Mol Cell Endocrinol. 288(1–2):95–103. Eng.
- Iwamori N. 2014. Regulation of spermatogonial stem cell compartment in the mouse testis. Fukuoka Igaku Zasshi. 105(1):1–10. Eng.
- Izadyar F, den Ouden K, Creemers LB, Posthuma G, Parvinen M, de Rooij DG. 2003. Proliferation and differentiation of bovine type A spermatogonia during long-term culture. Biol Reprod. 68(1):272–281.
- Jiang X, Cao HQ, Shi LY, Ng SY, Stanton LW, Chew SY. 2012. Nanofiber topography and sustained biochemical signaling enhance human mesenchymal stem cell neural commitment. Acta Biomater. 8(3):1290–1302. Eng.
- Kanatsu-Shinohara M, Shinohara T. 2013. Spermatogonial stem cell self-renewal and development. Annu Rev Cell Dev Biol. 29:163–187.
- Khanehzad M, Abolhasani F, Koruji SM, Ragerdi Kashani I, Aliakbari F. 2016. The roles of sertoli cells in fate determinations of spermatogonial stem cells [Original Article]. Tehran Univ Med J. 73(12):878–887. eng %@ 1683-1764 %[2016.
- Koruji M, Movahedin M, Mowla SJ, Gourabi H, Arfaee AJ. 2009. Efficiency of adult mouse spermatogonial stem cell colony formation under several culture conditions. In Vitro Cell Dev Biol Anim. 45(5–6):281–289. Eng.
- Kostereva N, Hofmann MC. 2008. Regulation of the spermatogonial stem cell niche. Reprod Domest Anim. 43(Suppl 2):386–392. Eng.
- Kubota H, Avarbock MR, Brinster RL. 2004a. Culture conditions and single growth factors affect fate determination of mouse spermatogonial stem cells. Biol Reprod. 71(3):722–731. Eng.
- Kubota H, Avarbock MR, Brinster RL. 2004b. Growth factors essential for self-renewal and expansion of mouse spermatogonial stem cells. Proc Natl Acad Sci U S A. 101(47):16489–16494. Eng.
- Larsson M, Norrander J, Graslund S, Brundell E, Linck R, Stahl S, Hoog C. 2000. The spatial and temporal expression of Tekt1, a mouse tektin C homologue, during spermatogenesis suggest that it is involved in the development of the sperm tail basal body and axoneme. Eur J Cell Biol. 79(10):718–725. Eng.
- Lee WY, Park HJ, Lee R, Lee KH, Kim YH, Ryu BY, Kim NH, Kim JH, Moon SH, Park JK, et al. 2013. Establishment and in vitro culture of porcine spermatogonial germ cells in low temperature culture conditions. Stem Cell Res. 11(3):1234–1249. Eng
- Li Y, Guo G, Li L, Chen F, Bao J, Shi YJ, Bu H. 2015. Three-dimensional spheroid culture of human umbilical cord mesenchymal stem cells promotes cell yield and stemness maintenance. Cell Tissue Res. 360(2):297–307. Eng.
- Li Y, Wang X, Feng X, Liao S, Zhang D, Cui X, Gao F, Han C. 2014. Generation of male germ cells from mouse induced pluripotent stem cells in vitro. Stem Cell Res. 12(2):517–530. Eng.
- Lim JJ, Kim HJ, Kim KS, Hong JY, Lee DR. 2013. In vitro culture-induced pluripotency of human spermatogonial stem cells. Biomed Res Int. 2013:143028. Eng.
- Lin HS, Kuhn C, Kuo T. 1975. Clonal growth of hamster free alveolar cells in soft agar. J Exp Med. 142(4):877–886. Eng.
- Mahmoud H. 2012. Concise review: spermatogenesis in an artificial three-dimensional system. Stem Cells. 30(11):2355–2360. Eng.
- Mark M, Jacobs H, Oulad-Abdelghani M, Dennefeld C, Feret B, Vernet N, Codreanu CA, Chambon P, Ghyselinck NB. 2008. STRA8-deficient spermatocytes initiate, but fail to complete, meiosis and undergo premature chromosome condensation. J Cell Sci. 121(Pt19)):3233–3242. Eng.
- Meng X, Lindahl M, Hyvonen ME, Parvinen M, de Rooij DG, Hess MW, Raatikainen-Ahokas A, Sainio K, Rauvala H, Lakso M, et al. 2000. Regulation of cell fate decision of undifferentiated spermatogonia by GDNF. Science (New York, NY). 287(5457):1489–1493. Eng
- Miyamoto T, Minase G, Okabe K, Ueda H, Sengoku K. 2015. Male infertility and its genetic causes. J Obstet Gynaecol Res. 41(10):1501–1505. Eng.
- Mohammadzadeh E, Mirzapour T, Nowroozi MR, Nazarian H, Piryaei A, Alipour F, Modarres Mousavi SM, Ghaffari Novin M. 2019. Differentiation of spermatogonial stem cells by soft agar three-dimensional culture system. Artif Cells Nanomed Biotechnol. 47(1):1772–1781. Eng.
- Muduli S, Chen L-H, Li M-P, Heish Z-W, Liu C-H, Kumar S, Alarfaj AA, Munusamy MA, Benelli G, Murugan K. 2017. Stem cell culture on polyvinyl alcohol hydrogels having different elasticity and immobilized with ECM-derived oligopeptides. J Poly Eng. 37(7):647–660.
- Navid S, Abbasi M, Hoshino Y. 2017. The effects of melatonin on colonization of neonate spermatogonial mouse stem cells in a three-dimensional soft agar culture system. Stem Cell Res Ther. 8(1):233. Eng.
- Nur EKA, Ahmed I, Kamal J, Schindler M, Meiners S. 2006. Three-dimensional nanofibrillar surfaces promote self-renewal in mouse embryonic stem cells. Stem Cells. 24(2):426–433. Eng.
- Oatley JM, Avarbock MR, Brinster RL. 2007. Glial cell line-derived neurotrophic factor regulation of genes essential for self-renewal of mouse spermatogonial stem cells is dependent on Src family kinase signaling. J Biol Chem. 282(35):25842–25851. Eng.
- Oatley JM, Avarbock MR, Telaranta AI, Fearon DT, Brinster RL. 2006. Identifying genes important for spermatogonial stem cell self-renewal and survival. Proc Natl Acad Sci U S A. 103(25):9524–9529. Eng.
- Oatley JM, Oatley MJ, Avarbock MR, Tobias JW, Brinster RL. 2009. Colony stimulating factor 1 is an extrinsic stimulator of mouse spermatogonial stem cell self-renewal. Development. 136(7):1191–1199. Eng.
- Oatley MJ, Kaucher AV, Racicot KE, Oatley JM. 2011. Inhibitor of DNA binding 4 is expressed selectively by single spermatogonia in the male germline and regulates the self-renewal of spermatogonial stem cells in mice. Biol Reprod. 85(2):347–356. Eng.
- Ojaghi M, Soleimanifar F, Kazemi A, Ghollasi M, Soleimani M, Nasoohi N, Enderami SE. 2019. Electrospun poly-l-lactic acid/polyvinyl alcohol nanofibers improved insulin-producing cell differentiation potential of human adipose-derived mesenchymal stem cells. J Cell Biochem. 120(6):9917–9926. Eng.
- Park JE, Park MH, Kim MS, Park YR, Yun JI, Cheong HT, Kim M, Choi JH, Lee E, Lee ST. 2017. Porcine spermatogonial stem cells self-renew effectively in a three dimensional culture microenvironment. Cell Biol Int. 41(12):1316–1324. Eng.
- Picton HM, Wyns C, Anderson RA, Goossens E, Jahnukainen K, Kliesch S, Mitchell RT, Pennings G, Rives N, Tournaye H, et al. 2015. A European perspective on testicular tissue cryopreservation for fertility preservation in prepubertal and adolescent boys. Hum Reprod. 30(11):2463–2475. Eng
- Reda A, Hou M, Landreh L, Kjartansdottir KR, Svechnikov K, Soder O, Stukenborg JB. 2014. In vitro spermatogenesis - optimal culture conditions for testicular cell survival, germ cell differentiation, and steroidogenesis in rats. Front Endocrinol (Lausanne). 5:21. Eng.
- Reda A, Hou M, Winton TR, Chapin RE, Soder O, Stukenborg JB. 2016. In vitro differentiation of rat spermatogonia into round spermatids in tissue culture. Mol Hum Reprod. 22(9):601–612. Eng.
- Sankar S, Sharma CS, Rath SN, Ramakrishna S. 2017. Electrospun fibers for recruitment and differentiation of stem cells in regenerative medicine. Biotechnol J. 12(12):1700263.
- Sato T, Katagiri K, Kubota Y, Ogawa T. 2013. In vitro sperm production from mouse spermatogonial stem cell lines using an organ culture method. Nat Protoc. 8(11):2098–2104. Eng.
- Sato Y, Taniguchi M, Otoi T. 2012. Studying spermatogenesis by using in vivo and in vitro models: advantages and disadvantages of these models for practical use. J Vet Sci Technol. 3:3.
- Shabani R, Ashtari K, Behnam B, Izadyar F, Asgari H, Asghari Jafarabadi M, Ashjari M, Asadi E, Koruji M. 2016. In vitro toxicity assay of cisplatin on mouse acute lymphoblastic leukaemia and spermatogonial stem cells. Andrologia. 48(5):584–594.
- Shakeri M, Kohram H, Shahverdi A, Shahneh AZ, Tavakolifar F, Pirouz M, Shahrebabak HM, Koruji M, Baharvand H. 2013. Behavior of mouse spermatogonial stem-like cells on an electrospun nanofibrillar matrix. J Assist Reprod Genet. 30(3):325–332. Eng.
- Shams A, Eslahi N, Movahedin M, Izadyar F, Asgari H, Koruji M. 2017. Future of spermatogonial stem cell culture: application of nanofiber scaffolds. Curr Stem Cell Res Ther. 12(7):544–553. eng.
- Shetty G, Meistrich ML. 2007. The missing niche for spermatogonial stem cells: do blood vessels point the way? Cell Stem Cell. 1(4):361–363. Eng.
- Simon L, Ekman GC, Tyagi G, Hess RA, Murphy KM, Cooke PS. 2007. Common and distinct factors regulate expression of mRNA for ETV5 and GDNF, Sertoli cell proteins essential for spermatogonial stem cell maintenance. Exp Cell Res. 313(14):3090–3099. Eng.
- Sousa AM, Souza HK, Uknalis J, Liu SC, Goncalves MP, Liu L. 2015. Electrospinning of agar/PVA aqueous solutions and its relation with rheological properties. Carbohydr Polym. 115:348–355. Eng.
- Stukenborg JB, Schlatt S, Simoni M, Yeung CH, Elhija MA, Luetjens CM, Huleihel M, Wistuba J. 2009. New horizons for in vitro spermatogenesis? An update on novel three-dimensional culture systems as tools for meiotic and post-meiotic differentiation of testicular germ cells. Mol Hum Reprod. 15(9):521–529. Eng.
- Stukenborg JB, Wistuba J, Luetjens CM, Elhija MA, Huleihel M, Lunenfeld E, Gromoll J, Nieschlag E, Schlatt S. 2008. Coculture of spermatogonia with somatic cells in a novel three-dimensional soft-agar-culture-system. J Androl. 29(3):312–329. Eng.
- Talebi A, Gilani MAS, Koruji M, Ai J, Rezaie MJ, Navid S, Salehi M, Abbasi MJGMJ. 2019. Colonization of mouse spermatogonial cells in modified soft agar culture system utilizing nanofibrous scaffold: a new approach. Galen Med J. 8. 1319.
- Tegelenbosch RA, de Rooij DG. 1993. A quantitative study of spermatogonial multiplication and stem cell renewal in the C3H/101 F1 hybrid mouse. Mutat Res. 290(2):193–200. Eng.
- Vacanti JP, Langer R. 1999. Tissue engineering: the design and fabrication of living replacement devices for surgical reconstruction and transplantation. Lancet. 354(Suppl 1):SI32–34. Eng.
- van Pelt AM, Morena AR, van Dissel-emiliani FM, Boitani C, Gaemers IC, de Rooij DG, Stefanini M. 1996. Isolation of the synchronized A spermatogonia from adult vitamin A-deficient rat testes. Biol Reprod. 55(2):439–444. Eng.
- Weaver VM, Petersen OW, Wang F, Larabell CA, Briand P, Damsky C, Bissell MJ. 1997. Reversion of the malignant phenotype of human breast cells in three-dimensional culture and in vivo by integrin blocking antibodies. J Cell Biol. 137(1):231–245. Eng.
- Yamauchi K, Hasegawa K, Chuma S, Nakatsuji N, Suemori H. 2009. In vitro germ cell differentiation from cynomolgus monkey embryonic stem cells. PLoS One. 4(4):e5338. Eng.
- Yoshida S. 2010. Stem cells in mammalian spermatogenesis. Dev Growth Differ. 52(3):311–317. Eng.
- Yoshida S. 2012. Elucidating the identity and behavior of spermatogenic stem cells in the mouse testis. Reproduction. 144(3):293–302. Eng.
- Yoshida S, Sukeno M, Nabeshima Y. 2007. A vasculature-associated niche for undifferentiated spermatogonia in the mouse testis. Science (New York, NY). 317(5845):1722–1726. Eng.
- Zhang S. 2004. Beyond the Petri dish. Nat Biotechnol. 22(2):151–152. Eng.