ABSTRACT
Cryopreservation can induce damage in human spermatozoa through reactive oxygen species (ROS) generation. To reduce the potential risk of oxidative stress-induced sperm DNA damage, addition of different epigallocatechin-3-gallate (EGCG) concentrations were performed to determine the optimum concentration which was beneficial for IVF outcome for both fresh and frozen-thawed sperm. Next, the mouse sperm model exhibiting oxidative stress-induced DNA damage by exogenously treating with H2O2 but overcoming the low fertilization rate of frozen-thawed sperm was used to investigate the effect of EGCG on the embryonic development and the potential EGCG-mediated effects on ataxia telangiectasia mutated (ATM) pSer-1981 in zygotes, the latter was known for leading to the activation of major kinases involved in the DNA repair pathway and the cell cycle checkpoint pathway. We found the fertilization and embryonic development of embryos inseminated with frozen-thawed sperm was impaired compared to fresh sperm. EGCG promoted the development of embryos inseminated with both types of sperm at optimum concentration. In embryos inseminated with the H2O2 sperm, fertilization, embryonic development, and the time at which the cleavage rate of one-cell embryos reached ≥95% were not affected by EGCG treatment. However, the EGCG-treated group required less time to achieve 50% cleavage rate of one-cell embryos, and the EGCG-treated zygotes showed enhanced expression of ATM (pSer-1981) than the untreated group. EGCG at optimum concentrations may exert beneficial effects by modulating the ATM activation and moving up the time to enter into mitotic (M) phase.
Abbreviations
ROS: reactive oxygen species; EGCG: epigallocatechin-3-gallate; ATM: ataxia telangiectasia mutated; M: mitotic
Introduction
Cryopreservation of human semen is a widely used procedure in both research and reproductive technology. This procedure enables the preservation of fertility among male patients with anejaculation, azoospermia, severe oligospermia, and malignancy. The cryopreservation technique for human spermatozoa has markedly improved over the last few decades (Agarwal and Tvrda Citation2017). The cryopreservation of human spermatozoa allows the patients to father children through intrauterine insemination (IUI), in vitro fertilization (IVF), or intra-cytoplasmic sperm injection (ICSI). However, the effect of cryopreservation on sperm deoxyribonucleic acid (DNA) damage remains controversial. Ribas-Maynou et al. (Citation2014) reported that although cryopreservation results in oxidative stress-induced DNA damage, cryopreservation did not exhibit any effect on single- and double-stranded DNA fragmentation, which were previously reported to be associated with oxidative damage. Some studies propose that the detrimental effects of the cryopreservation process include structural and functional alterations in the spermatozoa that result in congenital malformations or health problems (Ozmen et al. Citation2007). Enhanced DNA damage was reported in sperm after cryopreservation (Alkmin et al. Citation2013; Paoli et al. Citation2014). The cryopreservation process impairs the viability, motility, and mitochondrial membrane potential (MMP) of sperm. Additionally, cryopreservation enhances apoptosis, DNA damage, and reactive oxygen species (ROS) levels in sperm (Li et al. Citation2010). Enhanced ROS levels in sperm result in oxidative stress, which is an imbalance between oxidation and reduction. Oxidative stress is reported to be a major factor that contributes to DNA damage, apoptosis, lipid peroxidation, and reduced motility in sperm, subsequently, oxidative stress in sperm increases the risk of male infertility/subfertility and birth defects (Chen et al. 2013b). One of the main disadvantages of assisted reproductive technology (ART) is that the natural selection barriers are bypassed. The natural selection barriers act on suboptimal sperm during its journey through the female reproductive tract and prevent the penetration of weak spermatozoa into the oocyte. ART enables the penetration of spermatozoa carrying DNA damage into the oocyte (Tarozzi et al. Citation2019). Mature sperm has very little ability to respond to DNA damage and cannot process the oxidative lesion. Hence, the DNA repair process mostly occurs in the oocyte (Hamatani et al. Citation2004; Aitken et al. Citation2014).
The detrimental effects of oxidative stress-induced DNA damage associated with the sperm cryopreservation can be mitigated with the addition of an antioxidant to the embryo culture system (Wang et al. 2013b). Li et al. (Citation2010) demonstrated that supplementing the cryoprotective medium with appropriate concentrations of ascorbate or catalase decreases the ROS levels and prevents the cryodamage to the sperm. Epigallocatechin-3-gallate (EGCG), which is the principal active ingredient of green tea, is reported to be a powerful antioxidant and free radical scavenger that protects the cells and DNA (Qi Citation2010). Administration of EGCG could ameliorate the ethanol-induced oxidative stress-related growth retardation by inhibiting the increase in hydrogen peroxide (H2O2) and malondialdehyde (MDA) (Long et al. Citation2010). Treating the embryo culture system with EGCG promotes embryonic development. Wang et al (2013b) demonstrated that supplementing in vitro maturation medium and in vitro culture medium with green tea polyphenols improves the pregnancy rate, which was accompanied with enhanced mRNA expression of antioxidant enzyme genes and a decrease in the apoptotic index (AI) in bovine blastocysts. However, the underlying mechanism involved in these changes mediated by EGCG treatment is not well understood.
In somatic cells, DNA damage triggers a complex set of responses, including cell cycle arrest, re-localization of DNA repair factors, and apoptosis depending on the severity of DNA damage (Krenning et al. Citation2019). One of the first cellular responses to DNA damage is the phosphorylation of H2AX at serine 139 located in the unique carboxyl-terminal tail, known as γH2AX (Pouliliou and Koukourakis Citation2014). This phosphorylation is catalyzed by the members of the phosphatidylinositol 3-kinase (PI3 K)-like family, which is a family of lipid kinases that mediate cellular functions, such as cell growth, proliferation, and DNA repair. The PI3 K-like family includes ataxia telangiectasia mutated (ATM) protein, ATM and Rad3-related (ATR) protein, and DNA protein kinase catalytic subunits (PKcs) (Kuo and Yang Citation2008). In its inactive form, ATM is sequestered as a dimer or higher-order multimer with the kinase domain bound to a region surrounding serine 1981. DNA damage induces rapid intermolecular auto-phosphorylation of serine 1981, which leads to dimer dissociation and initiation of cellular ATM kinase activity (Bakkenist and Kastan Citation2003). The activated ATM, a major kinase involved in the phosphorylation of H2AX (Suzuki et al. Citation2011), activates the effector kinases, checkpoint kinase 1 (Chk1) and checkpoint kinase 2 (Chk2) through a class of checkpoint factors named checkpoint mediators to initiate the cell cycle checkpoint pathway depending on different types of DNA damage (Sancar et al. Citation2004). However, there are very few studies that have evaluated DNA damage repair in embryos.
In this study, we investigated the effect of EGCG on the embryonic development. The effect of EGCG was examined on the fertilization and cleavage rates of embryos inseminated with fresh or frozen-thawed sperm. Additionally, we investigated the effect of EGCG on the one-cell cleavage rate and ATM activation in the embryos inseminated with sperm containing oxidative stress-induced DNA damage. ATM activation is an indirect indicator of ATM-related DNA damage repair mechanism (Wang et al. 2013a).
Figure 1. Effects of 10 μg/mL EGCG in different duration time on in vitro embryonic development of fresh sperm, EGCG was added to the FM only (the time duration of EGCG was only approximately 4–6 hours), or EM only (the time duration of EGCG started from 4–6 hours post insemination), or both (the time duration of EGCG started from insemination), the EGCG-induced embryonic development was similar between the different duration time, but it seemed all the rates in the group which EGCG was added from insemination were higher than others (P > 0.05).
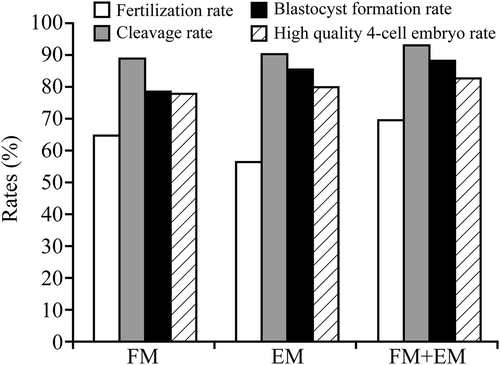
Figure 2. Concentration-dependent effects of EGCG on zygotes. Embryos (A-D, 2-cell; B-E, 4-cell) and blastocytes (C-F) with 10 μg/mL EGCG in FM and EM (A, B, C) or without EGCG (D, E, F), optimum concentrations of EGCG were associated with higher quality embryos and better subsequent embryonic development, compared to the control group.
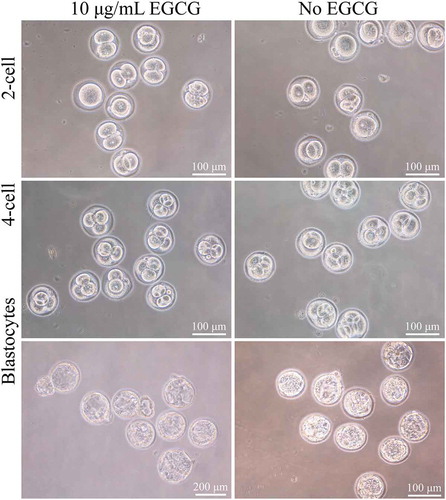
Figure 3. Effect of different EGCG concentrations in Both FM and EM in vitro embryonic development of frozen-thawed sperm. A similar pattern of EGCG-induced effects was observed with the frozen-thawed sperm (P < 0.05); however, the rates of all four features peaked at both 10 μg/mL and 17.5 μg/mL and the lowest rates were reached at 50 μg/mL. Specifically, the rates of fertilization and high quality four-cell embryo were significantly different than those measured in the untreated group (P < 0.05) at 10 and 17.5 μg/mL, especially remarkable for 17.5 μg/mL.

Figure 4. Formation of the pSer1981-ATM focuses in three groups. ATM (pSer-1981) was activated in both the treated group and the untreated group, but no ATM (pSer-1981) signal was detected in the control group. Fluorescence analysis indicated that the signal intensity was significantly stronger in the treated group than in the untreated group (0.0196 ± 0.0103 vs. 0.0083 ± 0.0066, P < 0.05).
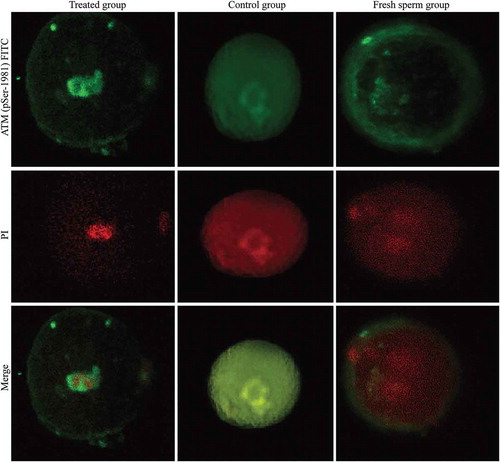
Results
Fertilization and embryo development rates following in vitro fertilization using either fresh or frozen-thawed sperm
As shown in , the rates of fertilization, cleavage, and blastocyst formation in embryos inseminated with frozen-thawed sperm were significantly lower than those in embryos inseminated with fresh sperm (P < 0.05). While the rate of high quality four-cell embryo formation in embryos inseminated with frozen-thawed sperm was numerically lower than that for embryos inseminated with fresh sperm, this difference did not reach the level of statistical significance (P > 0.05).
Table 1. Rates of in vitro fertilization and embryonic development achieved with fresh sperm and frozen-thawed sperm.
Effect of ECGC treatment on fertilization and embryo development rates following in vitro fertilization with either fresh or frozen-thawed sperm
The optimal EGCG concentration was determined for fresh sperm by evaluating the effects of various concentrations of EGCG (0, 5, 10, 15, 20, and 25 μg/mL) in FM, EM or in both EM and FM on the rates of fertilization, one-cell cleavage, high quality four-cell embryo formation, and blastocyst formation. As shown in –, the rates of fertilization, one-cell cleavage, high quality four-cell embryo formation, and blastocyst formation peaked at 10 μg/mL EGCG, except the rates of fertilization and cleavage in embryos inseminated with fresh sperm and treated with EGCG in EM only. However, there was no significant (P > 0.05) difference in the rates of fertilization and cleavage between the EGCG-treated group and the untreated group. The rates of high quality four-cell embryo formation and blastocyst formation in the EGCG-treated groups were significantly higher than those in the untreated group (P < 0.05). Increasing the EGCG concentration higher than 10 μg/mL resulted in a steady decrease in the rates of fertilization, one-cell cleavage, high quality four-cell embryo formation, and blastocyst formation. The lowest rates of fertilization, one-cell cleavage, high quality four-cell embryo formation, and blastocyst formation were observed at 25 μg/mL EGCG, except the fertilization rate of the embryos treated with EGCG in EM only. Furthermore, the EGCG-induced embryonic development was similar between the different treatment duration times. Although there were no significant differences between the three EGCG treatment duration times, the rates of fertilization, one-cell cleavage, high quality four-cell embryo formation, and blastocyst formation in the embryos treated with EGCG in both FM and EM were higher than those in the embryos treated with EGCG in FM or EM only (P > 0.05; ). Treatment with optimal concentrations of EGCG resulted in higher quality embryos and subsequently improved embryonic development when compared to the control group (). A similar pattern of EGCG-induced effects (0, 10, 17.5, 25, 50 μg/mL) was observed with the frozen-thawed sperm (P < 0.05). However, the highest rates of fertilization, one-cell cleavage, high quality four-cell embryo formation, and blastocyst formation were observed at both 10 μg/mL and 17.5 μg/mL EGCG, whereas the lowest rates were observed at 50 μg/mL EGCG (). Specifically, the rates of fertilization and high quality four-cell embryo formation in embryos treated with 10 μg/mL and 17.5 μg/mL EGCG were significantly higher than those in the untreated group (P < 0.05), especially 17.5 μg/mL. These data implied that EGCG at optimum concentrations is beneficial for IVF outcome for both fresh and frozen-thawed sperm.
Table 2. Effect of different EGCG concentrations in FM in vitro embryonic development of fresh sperm.
Table 3. Effect of different EGCG concentrations in EM in vitro embryonic development of fresh sperm.
Table 4. Effect of different EGCG concentrations in Both FM and EM in vitro embryonic development of fresh sperm.
Table 5. Effect of different EGCG concentrations in Both FM and EM in vitro embryonic development of frozen-thawed sperm.
Effect of EGCG on fertilization and embryo development rates following in vitro fertilization with H2O2 treated sperm
The murine model of 1 mM H2O2-induced DNA damage in spermatozoa was used to simulate the oxidative stress-induced DNA damage observed in the cryopreserved sperm. However, the low fertilization rate observed in the frozen-thawed sperm was mitigated in the H2O2-treated sperm. As shown in , the treatment with EGCG did not significantly affect (P > 0.05) the rates of fertilization (the treated group: 44.5% vs. the control group: 45.2%), one-cell embryo cleavage (97.8% vs. 100.0%), and two-cell embryo cleavage (71.3% vs. 61.5%). Additionally, EGCG treatment did not affect the time at which the cleavage rate of one-cell embryos reached ≥95% (22.7 ± 1.0 hpi vs. 22.5 ± 1.1 hpi). However, there was a significant difference (P < 0.05) in the cleavage rates of one-cell embryos between the EGCG-treated and untreated groups at the 17.5 hpi (70% vs. 34%) and 18.5 hpi (83% vs. 68%) time points (). Additionally, the EGCG-treated group required less time to achieve 50% cleavage (17.1 ± 0.0280 hpi vs. 18.25 ± 0.0598 hpi) when compared to the control group. This indicated that the EGCG-treated group entered mitosis approximately one hour earlier than the untreated group.
Table 6. Rates of in vitro fertilization and cleavage for one- and two-cell embryos achieved in the treated group and untreated group.
Enhanced expression of ATM (pSer-1981) in EGCG-treated zygotes fertilized with H2O2-treated sperm
We detected ATM (pSer-1981) activation in both EGCG-treated and untreated groups. However, the activation of ATM (pSer-1981) was not observed in the control group. Interestingly, only a faint ATM (pSer-1981) signal was detected in the nuclei of the untreated group, whereas enhanced ATM (pSer-1981) nuclear signal was observed in the EGCG-treated group. The fluorescence signal intensity in the EGCG-treated group was significantly stronger than that in the untreated group (0.0196 ± 0.0103 vs. 0.0083 ± 0.0066, P < 0.05; ).
Discussion
Semen cryopreservation is an important procedure in ART. A recent study has speculated that cryopreservation could cause sperm DNA damage (Esteves Citation2019). Previously, we had reported that cryopreservation of semen resulted in decreased viability, motility, and MMP as well as increased apoptosis, DNA damage, and ROS levels in the sperm (Li et al. Citation2010). This indicated that cryopreservation results in sperm DNA damage. Generally, low ROS levels play an important role in driving the tyrosine phosphorylation cascades during sperm capacitation. However, oxidative stress is established when the ROS production exceeds the limited antioxidant capacity of spermatozoa. The rates of fertilization, one-cell cleavage, and blastocyst formation in embryos inseminated with frozen-thawed sperm were markedly lower than those in embryos inseminated with fresh sperm. This indicated that the oxidative stress produced during the cryopreservation impairs the fertilizing potential of frozen-thawed sperm (Martins et al. Citation2019). Hence, DNA damage in spermatozoa is correlated with a higher miscarriage rate and lower good-quality embryo rate (Deng et al. Citation2019). These insights into the pathophysiology of defective sperm function have clear implications for the diagnosis and treatment of male infertility, particularly for antioxidant therapy. Supplementing the embryo culture system with antioxidants is reported to be an effective clinical approach for protecting the embryos against sperm-associated oxidative damage. Tatemoto et al. (Citation2001) reported that supplementing maturation medium with ascorbic acid 2-O-α-glycoside promotes the porcine oocyte cytoplasmic maturation, which is responsible for developmental competence after fertilization. Additionally, they demonstrated that this effect occurs by mitigating the oxidative stress in porcine oocytes. Thus, antioxidant supplementation is a potential strategy to reduce ROS levels and consequently prevent cryodamage (Li et al. Citation2010). EGCG exhibited a potential protective effect against oxidative stress-induced DNA damage and apoptosis (Saito et al. Citation2014). EGCG treatment was reported to promote both nuclear and cytoplasmic maturation of bovine oocytes and subsequent developmental competence of embryos embryonic development (Huang et al. Citation2018). However, the mechanism underlying the protective effect of EGCG is unknown
A previous study provided evidence that in vivo administration of the antioxidant EGCG could increase the rate of early cleaved embryos and blastocyst formation (Roth et al. Citation2008). Our results were consistent with the above, and we also found the optimal concentration of EGCG for frozen-thawed sperm is higher than that for fresh sperm. The difference in the EGCG concentration may be due to the enhanced ROS production in the frozen-thawed sperm induced during semen cryopreservation. One study proposed that the protective effect of EGCG can be attributed to its ROS scavenging activity rather than metal chelating activity (An et al. Citation2014). Thus, the beneficial effects of EGCG may be due to the scavenging of extra ROS produced during the cryopreservation process and IVF procedure that would otherwise damage the resultant embryos. In the present study, EGCG showed a dose-dependent affect on embryonic development. A similar dose dependent phenomenon of EGCG on bovine oocytes matured in vitro was reported by Huang et al. (Citation2018). But the optimal concentration of EGCG was 50 μg/mL, which was higher than 17.5 μg/mL in our study. It was presumably due to the difference between experimental subjects. Shirakami and Shimizu (Citation2018) reviewed the anti-oxidant and pro-oxidant activities of green tea catechins (GTCs) and summarized that GTCs can generate reactive oxygen species (ROS), which are essential for the induction of apoptosis and lead to the inhibition of cancer cell growth. The pro-oxidant activity might be the reason that EGCG adversely affected embryonic development when its concentration exceeded the optimal concentration.
A murine model of H2O2-induced sperm DNA damage had been established by Xiao et al. (Citation2012) and provided the basis of our follow up experiments. In this model, the sperm exhibited the same level of DNA damage (evaluated using γH2AX level) as observed in the frozen-thawed sperm. However, the low fertilization rate observed in the frozen-thawed sperm was mitigated in the H2O2-treated sperm. The expression of γH2AX was detected in one- and four-celled embryos that were fertilized with 1-mM H2O2-treated sperm. However, the expression of γH2AX was not detected in the embryos inseminated with fresh spermatozoa (Xiao et al. Citation2012). Additionally, Wang et al (2013a) observed that the zygotes fertilized with H2O2-treated sperm exhibited G2/M cell cycle arrest. The expression of ATM (pSer-1981) and Chk1 (pSer-345) was detected in the zygotes fertilized with the H2O2-treated sperm. These results suggested that the DNA damage repair and the cell cycle checkpoints were functionally effective in the embryos inseminated with H2O2-treated sperm. ATM was activated and functioned as the co-upstream factor of DNA damage repair and cell cycle checkpoint mechanism to resolve the genomic stability in the sperm exhibiting H2O2-induced DNA damage. Hence, we investigated the potential protective mechanism of EGCG on embryos inseminated with H2O2-treated sperm by examining the effect of EGCG treatment on embryonic cleavage time and activation of ATM.
At optimum concentrations, EGCG did not exhibit any positive effects on the development of embryos inseminated with H2O2-treated sperm. This may be due to the specific characteristic of this novel model. In the present study, EGCG treatment advanced the cleavage timing by approximately 1 h than the untreated embryos inseminated with H2O2-treated sperm. Moreover, the one-cell cleavage timing was significantly different between the EGCG-treated (17.5 h) and untreated (18.5 h) embryos inseminated with H2O2-treated sperm (P < 0.05), the timepoints were in accordance with the previous study demonstrated that the onset of G2-M phase was 17.0 ± 0.8 hpi for fresh sperm and 17.7 ± 0.6 hpi for H2O2 treated sperm, and that the endpoints of M phase were 20.0 and 22.5 h for the fresh sperm group and the H2O2-treated sperm group, respectively (Wang et al. 2013a). This suggested that although EGCG did not affect the time to complete the first mitosis of one-cell embryos, it may have exerted its protective effect by modulating the cell cycle, which has been demonstrated in several recent studies. Aktas et al. (Citation2004) demonstrated that EGCG abrogated human myelin-specific CD4 + T cell proliferation by inducing G1/S cell cycle arrest and downregulating the cyclin-dependent kinase 4. Additionally, EGCG-treated HCT116 colon cancer cells was reported to exhibit accumulation of p53 and p21 in the G1 phase. This indicated that EGCG can induce p53-dependent p21-mediated cell cycle arrest and apoptosis (Thakur et al. Citation2010). Similarly, Chen et al (2013a) demonstrated that co-treatment with EGCG and sulforaphane can arrest the ovarian cancer cells in both G2/M and S phase, whereas sulforaphane treatment arrests the cells in G2/M phase. It may be possible that EGCG modulates the cell cycle to provide enough time for DNA damage repair. Our preliminary study had revealed that Chk1 (pSer-345) was activated in embryos inseminated with H2O2-treated sperm (Wang et al. 2013a). Further studies are needed to determine the activation of Chk1, onset of G1 phase, onset and endpoint of S phase, and endpoint of M phase in the EGCG-treated embryos inseminated with H2O2-treated sperm.
EGCG-treated pronuclear embryos inseminated with 1 mM H2O2-treated sperm exhibited enhanced expression of activated ATM (pSer1981-ATM) nuclear signal. This suggested that DNA damage repair is the potential cellular mechanism underlying the protective effect of EGCG on inseminated embryos with oxidative stress-induced DNA damage sperm. Consistent with our results, Chen et al (2013a) reported that EGCG can potentiate the expression of H2AX in ovarian cancer cells treated with sulforaphane. Farooqi et al. (Citation2015) summarized that ATM was activated by EGCG. As ATM activation and H2AX expression are reported to initiate DNA damage repair mechanism, further studies are needed to confirm whether the DNA repair mechanism is functional in one-cell embryos treated with EGCG.
Animal studies have suggested that EGCG treatment does not result in embryo or fetal toxicity or teratogenicity (Isbrucker et al. Citation2006a,b). However, supplementation of bioflavonoid during pregnancy is associated with an increased risk of infant and early childhood leukemia (Lanoue et al. Citation2010), which has raised concerns on the safety of EGCG supplementation in humans. Mouse blastocysts treated with 25–50 μM of EGCG exhibited a marked increase in apoptosis and a concomitant decrease in the total cell number. Notably, pretreatment with EGCG resulted in lower success rate of blastocyst implantation, increased resorption of post-implantation embryos, and decreased fetal weight (Fan and Chan Citation2014). Contrastingly, Wang et al (2013b) reported that supplementing in vitro maturation medium and in vitro culture medium with 15 μM of green tea polyphenols resulted in improved pregnancy rates. This improved pregnancy rate appears to be associated with the increase in the mRNA expression of antioxidant enzyme genes and the decrease in apoptotic index (AI) in bovine blastocysts. However, treatment with the highest dose (25 μM) of green tea polyphenols resulted in an increase in AI when compared to the treatment with 15 μM of green tea polyphenols. Similarly, a study on porcine oocytes revealed that high EGCG concentrations could negatively affect in vitro maturation and fertilization (Spinaci et al. Citation2008). We also observed a dose-dependent effect on the parameters of embryonic development (rates of fertilization, one-cell cleavage, high quality four-cell embryo formation, and blastocyst formation). EGCG exhibits both beneficial and harmful effects depending on the treatment dosage. In early 2006, a study was conducted to evaluate the safety of EGCG administration to pregnant rats during organogenesis and development. The overall no-observed adverse effect level (NOAEL) was equivalent to 200 mg/kg/day EGCG preparation (Isbrucker et al. Citation2006b).
It is important to note that our study was restricted to preimplantation embryos in mice and that the observed effects and safety of EGCG as well as the optimum concentrations may not be representative of those features at later embryo stages or in humans. Future studies are needed to investigate the longer-term effects of EGCG supplementation to embryo culture system. Moreover, it is important to note that the aged oocytes have an impaired ability to repair the sperm DNA (Govindaraj et al. Citation2015). Hence, further studies are need to determine the beneficial effect of EGCG supplementation for these patients. Nevertheless, this study indicates that EGCG supplementation at certain concentrations promote the development of embryos inseminated with oxidative stress-induced DNA damage sperm. The beneficial effects of EGCG may be due to the activation of ATM and due to the early entry into the M phase. EGCG may be an effective protective agent for cryopreserved sperm used in IVF.
Materials and methods
Mice
All mice were obtained from the animal center of Shantou University Medical College (Shantou, China). All procedures involving mice complied with the Guide for the Care of Use of Laboratory Animals published by the US National Institutes of Health (NIH Publication No. 85–23, revised 1996) and the National Animal Protection legislation of China. The experimental protocols were approved by the Laboratory Animal Ethics Committee of Shantou University Medical College (No. SUMC 2011–107). The study was approved by the Institutional Animal Care and Use Committee of Shantou University Medical College.
Collection of fresh sperm
160 male Kunming mice (KM; weighing 30–45 g) aged 3–6 months old were sacrificed by cervical dislocation and the spermatozoa were collected from the caudal epididymis. The sperm sample was subjected to capacitation by incubating the sample with fresh capacitating agent [1.5% (w/v) bovine serum albumin (BSA; JieRui, China) prepared in human tubal fluid (HTF; SAGE, USA) medium] at 5% carbon dioxide (CO2) and 37°C incubator (Thermo Electron, USA) for 1–1.5 h for subsequent use in IVF.
Sperm cryopreservation and thawing
R18S3 cryoprotectant was prepared as a 6% solution of 1.8 g skim milk powder in 30 mL milli-Q water and centrifuged at 15,000 g for 1 h at 4°C. The resultant supernatant (20 mL) was mixed with 12.5 mL milli-Q water and 7.2 g raffinose. The volume of the mixture was made up to 40 mL using milli-Q water. The mixture was agitated to solubilize the raffinose. Next, the mixture was filtered, aliquoted, and stored at −20°C. The processed spermatozoa were gently mixed with 1 mL of R18S3 cryoprotectant and incubated for 10–15 min at 37°C and 5% CO2. Next, 100 μL of the samples were first suspended in liquid nitrogen vapor (10 cm above the level of liquid nitrogen at −80°C) for 10 min and then fully submersed in liquid nitrogen (−196°C) for storage until use. After seven days of storage, the cryovials containing the sperm samples were removed from the liquid nitrogen and immediately immersed in a 37°C circulating water bath. The samples thawed completely within 1–2 minutes. From each of the thawed samples, 20 μL aliquot was diluted with 80 μL of HTF-HEPES buffer (pH 7.2–7.4). The diluted semen solutions were incubated at 5% CO2 and 37°C for 1 h and the concentrations were adjusted for subsequent analyses.
H2O2-induced DNA damage in spermatozoa
Previously, our laboratory has successfully established a murine model for H2O2-induced DNA damage in spermatozoa (Xiao et al. Citation2012). Briefly, the spermatozoa were incubated with capacitation agent containing 0, 0.1, 0.5 or 1 mM H2O2 (Hengjian pharmaceutical co., China) at 37°C in a 5% CO2 incubator for 1.5 h. The motility and viability percentages of sperm were calculated. Additionally, immunocytochemistry was used to detect the presence of γH2AX, which is a DNA damage repair marker. We observed that the DNA damage level (determined based on γH2AX level) in 1 mM H2O2-treated sperm was similar to that in the cryopreserved sperm. Furthermore, the motility and viability percentages of sperm treated with 1 mM H2O2 were higher than those of cryopreserved sperm. Thus, we chose 1 mM H2O2 to simulate the oxidative stress-induced DNA damage observed in cryopreserved sperm for further experiments.
Collection of oocytes and in vitro fertilization
The ovulated oocytes arrested at metaphase II were collected 13 ~ 15 hours (hs) after human chorionic gonadotropin (hCG; Ningbo Second Hormone Factory, China) administration from the oviducts of 4–8 weeks old female KM mice (weighing 25–35 g, 160 mice). Prior to the oocyte collection, superovulation was induced by treating the mice with 10 IU of pregnant mare’s serum gonadotropin (PMSG; Ningbo Second Hormone Factory, China) for 48 h, followed by treatment with 10 IU of HCG. The harvested oocytes were cultured in microdrops (10 ~ 15 oocytes per microdrop) and then suspended in fertilization medium (FM, HTF medium containing 0.4% BSA) and inseminated with the three processed spermatozoan samples (range: 1–2.5 × 106/mL): fresh sperm, frozen-thawed sperm, or H2O2-treated sperm. Four to six hours post-insemination (hpi), the putative zygotes were washed thrice with HTF containing 0.4% BSA, and incubated in embryo culture medium (EM, HTF containing 0.4% BSA) at 5% CO2 and 37°C.
Fertilization and embryo development rates following in vitro fertilization using either fresh or frozen-thawed sperm
As reported previously (Howlett and Bolton Citation1985), the onset of G1 phase was determined to be the time point at which the second polar body released from the zygote. The embryos inseminated with fresh sperm and frozen-thawed sperm were comparatively collected and observed under a microscope once every hour from the G1 phase (16.5 hpi) to 23.5 hpi to evaluate the rates of fertilization and one-cell cleavage, then the rates of high quality 4-cell embryo formation and blastocyst formation were evaluated every 12 hours to 48hpi and the finish time was 3 ~ 4 days after insemination. Preimplantation embryos were divided into four grades based on the cell count and presence of DNA fragments (Rijnders and Jansen Citation1998). Grade I and II embryos were defined as high-quality embryos and only the rate of high quality four-cell embryo formation was calculated in this study.
Effect of ECGC treatment on fertilization and embryo development rates following in vitro fertilization with either fresh or frozen-thawed sperm
To evaluate the effects of EGCG with oocytes inseminated using fresh sperm, EGCG (Nanchang Beta Biotechnology Co., China) was added to the fertilization media (FM, the duration of EGCG treatment was approximately 4–6 h), embryonic development media (EM, the EGCG treatment was started from 4–6 h post-insemination), or both FM and EM (the EGCG treatment started from insemination) at concentrations ranging from 0 to 25 μg/mL (0, 5, 10, 15, 20, and 25 μg/mL). To evaluate the effects of EGCG with oocytes inseminated using frozen-thawed sperm, EGCG was added to the EM, FM, or both EM and FM as mentioned above at concentrations ranging from 0 to 50 μg/mL (0, 10, 17.5, 25, and 50 μg/mL). The rates of fertilization, one-cell cleavage, high-quality four-cell embryo formation, and blastocyst formation were comparatively evaluated between the fresh sperm-fertilized and frozen-thawed sperm-fertilized embryos.
Effect of EGCG on fertilization and embryo development rates following in vitro fertilization with H2O2 treated sperm
The murine model of 1 mM H2O2-induced DNA damage in spermatozoa was used in further experiments. The optimum concentration of EGCG was determined by treating the embryos inseminated with H2O2-treated sperm at various concentrations (0, 10, 17.5, 25, 50 μg/mL) of EGCG from insemination until the detecting time points. The embryos inseminated with H2O2-treated sperm were divided into two groups: the EGCG-treated group and the untreated group. The embryos inseminated with fresh sperm were used as the control group in the following experiments. The embryos in both EGCG-treated and untreated groups were collected and observed under a microscope once every hour from 16.5 hpi to 23.5 hpi. The rates of fertilization, one-cell cleavage, and two-cell cleavage were comparatively evaluated between the two groups.
Immunocytochemistry
Zygotes harvested at 6–8 hpi in Experiment 3–1 from EGCG-treated and untreated groups fertilized with 1 mM H2O2-treated sperm, and the control group inseminated with fresh sperm were washed with phosphate buffer saline (PBS, pH 7.2–7.4). The zona pellucida was removed by digestion with pancreatin (0.1%) for 30 s. Next, the zygotes were fixed with paraformaldehyde (Sangon Biotech. China, 4% in PBS) at 37°C for 1 h. The zygotes were washed thrice with PBS containing 0.05% Tween-20 (Solarbio, China) and permeabilized with 0.5% TritonX-100 (Solarbio, China) at room temperature (25°C) for 30 min. The zygotes were then incubated with primary pSer1981-ATM monoclonal antibody (1:500; Rockland, USA) at 4°C overnight, followed by incubation with the secondary Alexa Fluor® 488 goat anti-mouse IgG (H + L) antibody (1:200; Invitrogen, USA) at room temperature (25°C) for 1 h. The zygotes were counterstained with propidium iodide (PI) to visualize the DNA. The cells were mounted on glass slides with polyphosphate lysine (Boster, China) and imaged using a confocal laser scanning microscope (Nikon, Japan). The captured images were analyzed using the ImagePro Plus 6.0 software. At least 50 embryos pooled from five female mice were analyzed for each group. The experiments were repeated in duplicates to confirm the reproducibility of the results.
Statistical analysis
All statistical analyses were performed using the Statistics Package for the Social Sciences software, version 17.0 (SPSS Inc., USA). The difference was considered statistically significant when the P-value was less than 0.05. Percentage data comparisons between groups on embryonic development in Experiment 1 and 2 were analyzed by the Chi-squared (χ2) test. The results in Experiment 3–1 and 3–2 were expressed as means±standard error of the mean. Correlation of the one-cell cleavage rate between the EGCG-treated and untreated embryos in FM and EM were analyzed by Spearman’s correlation test. Lines in the dispersion plots were calculated by the linear regression method. ImagePro Plus 6.0 software was used for comparative analysis of the immunofluorescence intensity between different groups.
Author contributions
Designed the study, analyzed data, revised the paper, and approved the final version: ZL; performed the research, analyzed data, drafted the article, and approved the final version: MC, WL, WX.
Acknowledgments
We are grateful for the support from Center for Neuroscience, Shantou University Medical College for the utilization of the laser confocal microscopy.
Disclosure statement
No potential conflict of interest was reported by the authors.
Additional information
Funding
References
- Agarwal A, Tvrda E. 2017. Chapter 5 slow freezing of human sperm. Methods Mol Biol. 1568:67–78.
- Aitken RJ, Smith TB, Jobling MS, Baker MA, De Iuliis GN. 2014. Oxidative stress and male reproductive health. Asian J Androl. 16(1):31–38. doi:10.4103/1008-682X.122203.
- Aktas O, Prozorovski T, Smorodchenko A, Savaskan NE, Lauster R, Kloetzel PM, Infante-Duarte C, Brocke S, Zipp F. 2004. Green tea epigallocatechin-3-gallate mediates T cellular NF-kappa B inhibition and exerts neuroprotection in autoimmune encephalomyelitis. J Immunol. 173(9):5794–5800. doi:10.4049/jimmunol.173.9.5794.
- Alkmin DV, Martinez-Alborcia MJ, Parrilla I, Vazquez JM, Martinez EA, Roca J. 2013. The nuclear DNA longevity in cryopreserved boar spermatozoa assessed using the Sperm-Sus-Halomax. Represent spermatozoa with different degrees of nuclear DNA damage. Theriogenology. 79(9):1294–1300. doi:10.1016/j.theriogenology.2013.02.026.
- An Z, Qi Y, Huang D, Gu X, Tian Y, Li P, Li H, Zhang Y. 2014. EGCG inhibits Cd2+-induced apoptosis through scavenging ROS rather than chelating Cd2+ in HL-7702 cells. Toxicol Mech Methods. 24(4):259–267. doi:10.3109/15376516.2013.879975.
- Bakkenist CJ, Kastan MB. 2003. DNA damage activates ATM through intermolecular autophosphorylation and dimer dissociation. Nature. 421(6922):499–506. doi:10.1038/nature01368.
- Chen H, Landen CN, Li Y, Alvarez RD, Tollefsbol TO. 2013a. Epigallocatechin gallate and sulforaphane combination treatment induce apoptosis in paclitaxel-resistant ovarian cancer cells through hTERT and Bcl-2 down-regulation. Exp Cell Res. 319(5):697–706. doi:10.1016/j.yexcr.2012.12.026.
- Chen SJ, Allam JP, Duan YG, Haidl G. 2013b. Influence of reactive oxygen species on human sperm functions and fertilizing capacity including therapeutical approaches. Arch Gynecol Obstet. 288(1):191–199. doi:10.1007/s00404-013-2801-4.
- Deng C, Li T, Xie Y, Guo Y, Yang QY, Liang X, Deng CH, Liu GH. 2019. Sperm DNA fragmentation index influences assisted reproductive technology outcome: A systematic review and meta-analysis combined with a retrospective cohort study. Andrologia. 51(6):e13263. doi:10.1111/and.13263.
- Esteves SC. 2019. Interventions to prevent sperm DNA damage effects on reproduction. Adv Exp Med Biol. 1166:119–148.
- Fan YC, Chan WH. 2014. Epigallocatechin gallate induces embryonic toxicity in mouse blastocysts through apoptosis. Drug Chem Toxicol. 37(3):247–254. doi:10.3109/01480545.2013.838778.
- Farooqi AA, Wu SJ, Chang YT, Tang JY, Li KT, Ismail M, Liaw CC, Li RN, Chang HW. 2015. Activation and Inhibition of ATM by phytochemicals: awakening and sleeping the guardian angel naturally. Arch Immunol Ther Exp (Warsz). 63(5):357–366. doi:10.1007/s00005-015-0346-x.
- Govindaraj V, Keralapura Basavaraju R, Rao AJ. 2015. Changes in the expression of DNA double strand break repair genes in primordial follicles from immature and aged rats. Reprod Biomed Online. 30(3):303–310. doi:10.1016/j.rbmo.2014.11.010.
- Hamatani T, Carter MG, Sharov AA, Ko MS. 2004. Dynamics of global gene expression changes during mouse preimplantation development. Dev Cell. 6(1):117–131. doi:10.1016/S1534-5807(03)00373-3.
- Howlett SK, Bolton VN. 1985. Sequence and regulation of morphological and molecular events during the first cell cycle of mouse embryogenesis. J Embryol Exp Morphol. 87:175–206.
- Huang Z, Pang Y, Hao H, Du W, Zhao X, Zhu H. 2018. Effects of epigallocatechin-3-gallate on bovine oocytes matured in vitro. Asian-Australas J Anim Sci. 31(9):1420–1430. doi:10.5713/ajas.17.0880.
- Isbrucker RA, Bausch J, Edwards JA, Wolz E. 2006a. Safety studies on epigallocatechin gallate (EGCG) preparations. Part 1: genotoxicity. Food Chem Toxicol. 44(5):626–635. doi:10.1016/j.fct.2005.07.005.
- Isbrucker RA, Edwards JA, Wolz E, Davidovich A, Bausch J. 2006b. Safety studies on epigallocatechin gallate (EGCG) preparations. Part 3: teratogenicity and reproductive toxicity studies in rats. Food Chem Toxicol. 44(5):651–661. doi:10.1016/j.fct.2005.11.002.
- Krenning L, van den Berg J, Medema RH. 2019. Life or death after a break: what determines the choice? Mol Cell. 76(2):346–358. doi:10.1016/j.molcel.2019.08.023.
- Kuo LJ, Yang LX. 2008. Gamma-H2AX - a novel biomarker for DNA double-strand breaks. In Vivo. 22(3):305–309.
- Lanoue L, Green KK, Kwik-Uribe C, Keen CL. 2010. Dietary factors and the risk for acute infant leukemia: evaluating the effects of cocoa-derived flavanols on DNA topoisomerase activity. Exp Biol Med (Maywood). 235(1):77–89. doi:10.1258/ebm.2009.009184.
- Li Z, Lin Q, Liu R, Xiao W, Liu W. 2010. Protective effects of ascorbate and catalase on human spermatozoa during cryopreservation. J Androl. 31(5):437–444. doi:10.2164/jandrol.109.007849.
- Long L, Li Y, Wang YD, He QY, Li M, Cai XD, Peng K, Li XP, Xie D, Wen YL, et al. 2010. The preventive effect of oral EGCG in a fetal alcohol spectrum disorder mouse model. Alcohol Clin Exp Res. 34(11):1929–1936. doi:10.1111/j.1530-0277.2010.01282.x.
- Martins AD, Agarwal A, Henkel R. 2019. Sperm cryopreservation. In: Nagy Z, Varghese A, Agarwal A, editors. In vitro fertilization pp. 625-642. Cham: Springer. https://doi.org/10.1007/978-3-319-43011-9_51
- Ozmen B, Koutlaki N, Youssry M, Diedrich K, Al-Hasani S. 2007. DNA damage of human spermatozoa in assisted reproduction: origins, diagnosis, impacts and safety. Reprod Biomed Online. 14(3):384–395. doi:10.1016/S1472-6483(10)60883-8.
- Paoli D, Lombardo F, Lenzi A, Gandini L. 2014. Sperm cryopreservation: effects on chromatin structure. Adv Exp Med Biol. 791:137–150.
- Pouliliou S, Koukourakis MI. 2014. Gamma histone 2AX (γ-H2AX)as a predictive tool in radiation oncology. Biomarkers. 19(3):167–180. doi:10.3109/1354750X.2014.898099.
- Qi X. 2010. Reactive oxygen species scavenging activities and inhibition on DNA oxidative damage of dimeric compounds from the oxidation of (-)-epigallocatechin-3-O-gallate. Fitoterapia. 81(3):205–209. doi:10.1016/j.fitote.2009.09.004.
- Ribas-Maynou J, Fernández-Encinas A, García-Peiró A, Prada E, Abad C, Amengual MJ, Navarro J, Benet J. 2014. Human semen cryopreservation: a sperm DNA fragmentation study with alkaline and neutral Comet assay. Andrology. 2(1):83–87. doi:10.1111/j.2047-2927.2013.00158.x.
- Rijnders PM, Jansen CA. 1998. The predictive value of day 3 embryo morphology regarding blastocyst formation, pregnancy and implantation rate after day 5 transfer following in-vitro fertilization or intracytoplasmic sperm injection. Hum Reprod. 13(10):2869–2873. doi:10.1093/humrep/13.10.2869.
- Roth Z, Aroyo A, Yavin S, Arav A. 2008. The antioxidant epigallocatechin gallate (EGCG) moderates the deleterious effects of maternal hyperthermia on follicle-enclosed oocytes in mice. Theriogenology. 70:887–897. doi:10.1016/j.theriogenology.2008.05.053.
- Saito K, Mori S, Date F, Ono M. 2014. Epigallocatechin gallate inhibits oxidative stress-induced DNA damage and apoptosis in MRL-Fas(lpr) mice with autoimmune sialadenitis via upregulation of heme oxygenase-1 and Bcl-2. Autoimmunity. 47(1):13–22. doi:10.3109/08916934.2013.850079.
- Sancar A, Lindsey-Boltz LA, Unsal-Kacmaz K, Linn S. 2004. Molecular mechanisms of mammalian DNA repair and the DNA damage checkpoints. Annu Rev Biochem. 73(1):39–85. doi:10.1146/annurev.biochem.73.011303.073723.
- Shirakami Y, Shimizu M. 2018. Possible mechanisms of green tea and its constituents against cancer. Molecules. 23(9):E2284. doi:10.3390/molecules23092284.
- Spinaci M, Volpe S, De Ambrogi M, Tamanini C, Galeati G. 2008. Effects of epigallocatechin-3-gallate (EGCG) on in vitro maturation and fertilization of porcine oocytes. Theriogenology. 69(7):877–885. doi:10.1016/j.theriogenology.2008.01.005.
- Suzuki K, Yamauchi M, Yamashita S. 2011. ATM-dependent cellular response to DNA double strand breaks plays a pivotal role in the maintenance of the integrity of the genome. Radiat Prot Dosimetry. 143(2–4):279–283. doi:10.1093/rpd/ncq533.
- Tarozzi N, Nadalini M, Borini A. 2019. Effect on sperm DNA quality following sperm selection for ART: new insights. Adv Exp Med Biol. 1166:169–187.
- Tatemoto H, Ootaki K, Shigeta K, Muto N. 2001. Enhancement of developmental competence after in vitro fertilization of porcine oocytes by treatment with ascorbic acid 2-O-alpha-glucoside during in vitro maturation. Biol Reprod. 65(6):1800–1806. doi:10.1095/biolreprod65.6.1800.
- Thakur VS, Ruhul Amin AR, Paul RK, Gupta K, Hastak K, Agarwal MK, Jackson MW, Wald DN, Mukhtar H, Agarwal ML. 2010. p53-Dependent p21-mediated growth arrest pre-empts and protects HCT116 cells from PUMA-mediated apoptosis induced by EGCG. Cancer Lett. 296(2):225–232. doi:10.1016/j.canlet.2010.04.012.
- Wang B, Li Z, Wang C, Chen M, Xiao J, Wu X, Xiao W, Song Y, Wang X. 2013a. Zygotic G2/M cell cycle arrest induced by ATM/Chk1 activation and DNA repair in mouse embryos fertilized with hydrogen peroxide-treated epididymal mouse sperm. PLoS One. 8(9):e73987. doi:10.1371/journal.pone.0073987.
- Wang Z, Fu C, Yu S. 2013b. Green tea polyphenols added to IVM and IVC media affect transcript abundance, apoptosis, and pregnancy rates in bovine embryos. Theriogenology. 79(1):186–192. doi:10.1016/j.theriogenology.2012.10.002.
- Xiao J, Liu Y, Li Z, Zhou Y, Lin H, Wu X, Chen M, Xiao W. 2012. Effects of the insemination of hydrogen peroxide-treated epididymal mouse spermatozoa on gammaH2AX repair and embryo development. PLoS One. 7(6):e38742. doi:10.1371/journal.pone.0038742.