ABSTRACT
Our study established an effective next-generation sequencing (NGS) protocol for four-factor preimplantation genetic testing (PGT) using α- and β-thalassemia, human leukocyte antigen (HLA) typing, and aneuploidy screening. Three couples, in whom both partners were α- and β-double thalassemia carriers, underwent PGT between 2016 and 2018. These individuals sought an opportunity for hematopoietic stem cell transplantation to save their children from β-thalassemia major. A total of 35 biopsied trophectoderm samples underwent multiple displacement amplification (MDA). PGT for α- and β-thalassemia and HLA typing were performed on MDA products using NGS-based single-nucleotide polymorphism (SNP) haplotyping. Although two samples failed MDA, 94.3% (33/35) of samples were successfully amplified, achieving conclusive PGT results. Furthermore, 51.5% (17/33) of the embryos were diagnosed as unaffected non-carriers or carriers. Of the 17 unaffected embryos, nine (52.9%) were tested further and identified as euploid via NGS-based aneuploid screening, in which five had HLA types matching affected children. One family did not achieve any unaffected euploid embryos. The two other families transferred HLA-matched and unaffected euploid embryos, resulting in two healthy ‘savior babies.’ NGS-PGT results were confirmed in prenatal diagnosis. Therefore, NGS-SNP was effective in performing PGT for multipurpose detection within a single PGT cycle.
Introduction
Thalassemia is an autosomal recessive, heritable hemolytic disease with a global prevals (Weatherall and Clegg Citation2001). Provinces in southern China, especially Guangdong, Guangxi, Yunnan, and Hainan, have a high incidence of thalassemia (Lai et al. Citation2017). Among them, Guangxi accounted for the highest prevalence of α- and β-thalassemia, reaching 14.13% and 4.91%, respectively (Lai et al. Citation2017).
Globins’ molecular structure is determined by α-globin (16p13.3) and β-globin genes (11p15.5) (Mettananda and Higgs Citation2018; Thein Citation2018), in which both deletions and point mutations cause imbalances in globin chains, resulting in nonspecific a range of symptoms from to severe anemia (Mettananda and Higgs Citation2018). Deletion of four α-globin genes can cause Hb 7hydrops fetalis, a lethal syndrome characterized by stillbirth or death shortly after birth (Liang et al. Citation1985; Farashi and Harteveld Citation2018). Patients with β-thalassemia intermedia are asymptomatic at birth, with symptoms such as moderate anemia, bone disease, and splenomegaly not presenting until 2 years of age. Patients with β-thalassemia major, however, commonly develop severe anemia before 2 years of age, generally dying before 5 years of age, if timely treatment with regular red blood cell transfusions combined with iron chelation is not received (Origa Citation2017).
Since 1998, an increasing number of couples have delivered thalassemia-free babies through preimplantation genetic testing (PGT) for monogenic diseases (PGT-M), a less invasive option compared to other prenatal diagnostic tests (Kuliev et al. Citation1998; Vrettou et al. Citation2018). At present, applying PGT to α- or β-thalassemia alone is clinically mature; although in some cases, both the husband and wife are carriers of α- and β-double thalassemia, with a prevalence is 0.48% in the Chinese population (Lai et al. Citation2017). Simultaneous PGT-M for both thalassemia types has seldom been reported. Previously, we successfully conducted simultaneous PGT for the two types of thalassemia using polymerase chain reaction (PCR)-based detection (Shen et al. Citation2011) and short tandem repeat (STR) haplotyping (Shen et al. Citation2013). However, these approaches were characterized by adverse influences from allele dropouts (ADOs), limited STR loci, and laborious operations.
Currently, β-thalassemia major survival depends on receiving a hematopoietic stem cell transplantation (HSCT) treatment. Unfortunately, finding a bone marrow donor with compatible human leukocyte antigen (HLA) typing is difficult and time-consuming. PGT not only screens for thalassemia-unaffected embryos but also helps to select those with an HLA profile compatible with an affected child, making it possible for these families to deliver ‘savior babies’ (Verlinsky et al. Citation2001; Kakourou et al. Citation2017). According to the statistics between 1997 and 2007, 75.5% of PGT-M + HLA cycles were conducted for patients with β-thalassemia (Harper et al. Citation2012).
High throughput, large scale, and high-speed next-generation sequencing (NGS) has favorable applications in the field of PGT (Sullivan-Pyke and Dokras Citation2018), with its ability to perform linkage analysis via single-nucleotide polymorphisms (SNPs), which are ideal genetic markers widely distributed throughout the genome. The NGS platform combined with SNP-based haplotyping effectively eliminates diagnostic errors generated by ADOs, thus enabling concurrent genetic diagnosis and aneuploidy screening (Chen et al. Citation2016, Citation2017; Hao et al. Citation2018; Backenroth et al. Citation2019; Ji et al. Citation2019). Herein, we successfully used NGS to achieve simultaneous PGT for α- and β-thalassemia and HLA typing. Moreover, PGT for aneuploidy screening (PGT-A) using NGS was performed in the same PGT cycle to ensure euploid embryo transfer.
Results and discussion
Three families underwent PGT treatment between 2016 and 2018. In each family, both the husband and wife were carriers of α- and β-double thalassemia mutations or deletions. Moreover, each family had a surviving child with β-thalassemia major waiting for HSCT treatment. summarizes the basic information, reproductive history, and thalassemia genotypes of these families. Karyotype examination revealed no chromosomal abnormalities in these couples. A pedigree analysis was performed for each of the three enrolled families using NGS-based SNP haplotyping, in which paternal and maternal pathogenic haplotypes were distinguished. displays the number of informative SNPs and the informative rate for each family. We take Family 1 as an example to illustrate the results of SNP haplotyping. For α-thalassemia, both daughter (II-1) and father (I-1) were heterozygous for SEA deletion, while the mother (I-2) was heterozygous for −α3.7 deletion (). Therefore, the same haplotype between I-1 and II-1 was the pathogenic haplotype of paternal origin, and the haplotype carried by I-2, but different from II-1, was the pathogenic haplotype of maternal origin. For β-thalassemia, the pathogenic haplotypes were the two haplotypes carried by II-1, as the genotype of II-1 was CD41-42/CD17 (). The haplotypes for HLA were also detected by NGS-SNP (), and only embryos carrying the same two haplotypes as II-1 considered HLA matched, thus qualifying for HSCT.
Table 1. Basic information, genotypes of thalassemia, and reproductive history of the three families
Figure 1. Results of SNP-based pedigree analysis and PGT for α-thalassemia (Family 1). I-1: male patient. I-2: female patient. II-1: proband. SNP: single-nucleotide polymorphism. PGT: preimplantation genetic testing. ‘?’ indicates that no detection result was obtained for this SNP site. Double horizontal lines represent pathogenic site boundaries. In pedigree analysis, I-1 and II-1 were both heterozygous for the SEA deletion, while I-2 was heterozygous for the −α3.7 deletion. Therefore, the same haplotype between I-1 and II-1 was the pathogenic haplotype of paternal origin (marked with a dark blue background). The haplotype carried by I-2, but different from II-1, was the pathogenic haplotype of maternal origin (marked with a brown background). Based on SNP-based PGT, Embryo 7 was diagnosed as affected because it carried two pathogenic haplotypes; the other six embryos were unaffected carriers or noncarriers as they carried only one, or no, pathogenic haplotype
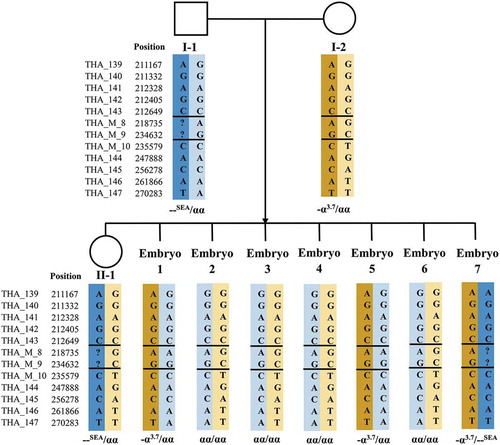
Figure 2. Results of SNP-based pedigree analysis and PGT for β-thalassemia (Family 1). I-1: male patient. I-2: female patient. II-1: proband. SNP: single-nucleotide polymorphism. PGT: preimplantation genetic testing. ‘?’ indicates that no detection result was obtained for this SNP site. Double horizontal lines represent pathogenic site boundaries. In pedigree analysis, as the genotype of II-1 was CD41-42/CD17, pathogenic haplotypes were the two carried by II-1 (marked with dark red and dark green backgrounds). Based on SNP-based PGT, all seven embryos were identified as unaffected carriers or noncarriers because they carried only one, or no, pathogenic haplotype
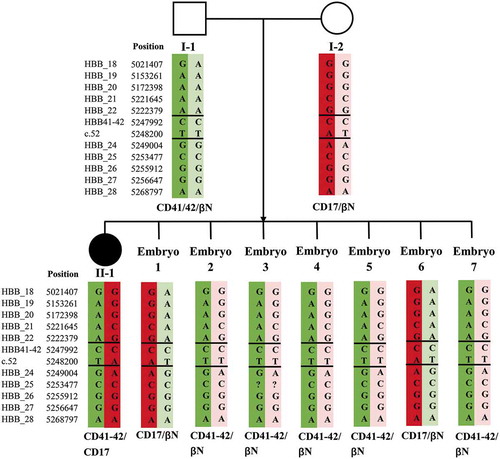
Figure 3. Results of SNP-based PGT for HLA typing (Family 1). I-1: male patient. I-2: female patient. II-1: proband. SNP: single-nucleotide polymorphism. PGT: preimplantation genetic testing. HLA: human leukocyte antigen. ‘Matched’: HLA type of the tested embryo matched with that of II-1. ‘Unmatched’: HLA type of the tested embryo did not match with that of II-1. Based on SNP-based PGT, Embryos 1, 2, 3, and 4 were identified as HLA types matching with II-1; the other three did not match
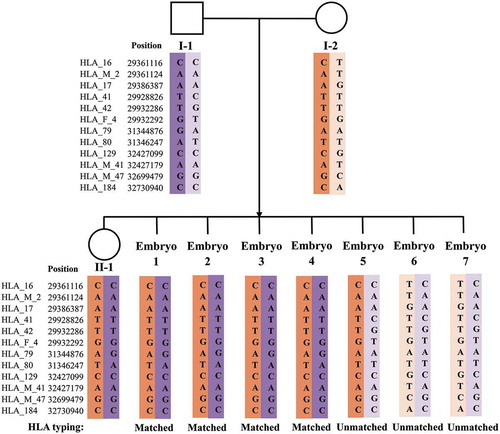
Table 2. Informative rate of selected SNPs for each family
The three couples underwent five oocyte pickup (OPU) and three PGT cycles. Following this, we retrieved 82 oocytes, 53 of which were normally fertilized through standard intracytoplasmic sperm injection (ICSI), resulting in 44 day-3 embryos. Finally, 35 embryos were cultured into blastocysts and subjected to trophectoderm biopsy. Although two trophectoderm samples failed multiple displacement amplification (MDA), 94.3% (33/35) of samples successfully amplified, obtaining conclusive PGT results. In total, 51.5% (17/33) of the embryos were diagnosed as unaffected non-carriers or carriers of thalassemia mutations. Furthermore, PGT-A results on these 17 embryos showed that nine (52.9%) were euploid, with five HLA matched and four HLA unmatched. summarizes the results of the three PGT cycles.
Table 3. Summary of the PGT cycles performed for the three families
A total of 27.3% (9/33) of embryos, both unaffected and euploid, were transferable. The overall rate of obtaining an embryo qualified for HSCT purposes (both transferable and HLA matched) was 15.2% (5/33). In particular, for Family 1, six of the seven embryos were unaffected by both α- and β-thalassemia; five of these were further identified as euploid, suggesting that Family 1 achieved five transferable embryos. Moreover, four transferable embryos were of the same HLA type as II-1, therefore qualifying for HSCT. For Family 2, none of the eight embryos were transferable. For Family 3, four transferable embryos were obtained, but only one was HLA matched. Supplemental shows detailed PGT outcomes for each embryo.
For Families 1 and 3, each couple transferred one HLA matched and unaffected euploid embryo in a frozen–thawed embryo transfer (FET) cycle. Both families achieved clinical pregnancy and were diagnosed with thalassemia mutations, HLA typing, and aneuploidy during the mid-trimester of pregnancy. Prenatal diagnosis results were consistent with those of NGS-based PGT. Families 1 and 3 both achieved healthy live births with HLA profiles compatible with respective probands.
Our study reported the successful application of NGS-based four-factor PGT, comprising two types of thalassemia, HLA typing, and aneuploidy screening. Our results support NGS for multipurpose detection in one PGT cycle when combined with whole-genome amplification (WGA) techniques.
Multipurpose PGT-M is rarely reported. Two studies from our center demonstrated the feasibility of PCR- and STR-based PGT for α- and β-double thalassemia (Shen et al. Citation2011, Citation2013). Additionally, one study reported simultaneous PGT for β-thalassemia, sideroblastic anemia, and HLA typing using ST7-based methods (Kakourou et al. Citation2016). Although clinical results in these studies were satisfactory, diagnostic methods required further improvement. ADO is the most common factor affecting PCR-based diagnosis accuracy. Given that informative STR loci are often limited, can only partially alleviate ADO-associated risk of misdiagnosis. Moreover, the accuracy of the results was also compromised via recombination between STRs, disease genes, and HLA loci (Gueye et al. Citation2014).
PGT-M for multiple loci is challenging both clinically (considerable number of embryos required) and experimentally (due to the small amount of starting material requiring preamplification). Compared with previous PGT-M methods, NGS is a powerful platform with improved throughput, speed, accuracy, and cost-effectiveness (Lee et al. Citation2017; Sullivan-Pyke and Dokras Citation2018). When combined with WGA, NGS performs well for different PGT indications, including monogenic disease, HLA typing, and aneuploidy (Fiorentino et al. Citation2014; Rafati et al. Citation2018; Ji et al. Citation2019). Hence, it is inevitable that NGS-based PGT will be used for multiple purposes. Our study supports that the MDA-NGS method can simultaneously detect two monogenic disorders and HLA typing. Although MDA-based WGA may cause significant amplification bias (de Bourcy et al. Citation2014), this defect may be minimized through linkage analysis incorporating numerous SNPs. In our study, 243 and 60 to 190 closely linked SNPs were chosen for α- and β-thalassemia haplotyping, respectively; far more than in the STR-based method. Although ADO occurred in a few loci, the abundance of SNP loci sufficiently compensated for this effect, similar to a study by Chen et al. (Chen et al. Citation2017). In previous studies, NGS-SNP and Sanger sequencing obtained consistent PGT-M results (Chen et al. Citation2016; Ji et al. Citation2019), supporting the accuracy of NGS-SNP-based PGT-M.
To date, methods, such as single-cell PCR (Verlinsky et al. Citation2001) and minisequencing, have been established to identify HLA-matched embryos (Fiorentino et al. Citation2004). As the HLA complex has a high degree of polymorphism, both methods require unique PCR primers to be designed for each family, rendering them inefficient. Another method, microsatellite technology, depends on the use of STRs (Fiorentino et al. Citation2004, Citation2005). Although this general HLA typing method avoids designing specific protocols for each family, its limitation is the shortage of the STR method, as described above. Recently emerging methods, such as SNP- and NGS-related technologies, provide increased detection loci, read lengths, resolution, and throughput, thus avoiding the shortcomings of traditional methodologies (Handyside et al. Citation2010; Xu et al. Citation2015; Carapito et al. Citation2016; Rafati et al. Citation2018). In our study, we verified that using NGS with SNP linkage analysis is feasible for HLA typing in preimplantation embryos. NGS-SNP-based HLA typing will eventually play a greater role in screening ‘savior babies’ in PGT-M practice.
Although common indications for PGT-A for couples without chromosomal abnormalities are advanced maternal age and a history of recurrent spontaneous miscarriage, recent studies have supported the addition of aneuploidy screening in the PGT-M cycle to improve pregnancy outcomes (Rechitsky et al. Citation2015), even for younger women (Hou et al. Citation2019). In our study, we performed PGT-A via NGS-based low-coverage genomic sequencing on the same samples to ensure euploid embryo transfer. Notably, Haploseek, a more sophisticated NGS method, was introduced by Backenroth et al. for concurrent PGT, but not for stepwise PGT-M and PGT-A, as outlined in our study (Backenroth et al. Citation2019). Combining copy-number variant detection with whole-genome haplotype prediction could achieve simultaneous PGT-M and PGT-A within 24 h (Backenroth et al. Citation2019).
Although NGS-based PGT is a highly accurate and reliable clinical tool, it may sometimes yield an inconclusive diagnosis (Neal et al. Citation2019). Whether poor embryo quality is associated with inconclusive diagnoses remains controversial (Cimadomo et al. Citation2018; Neal et al. Citation2019). However, technical issues occurring in biopsy, shipping, storage, amplification, and loading may lead to inconclusive PGT results (Neal et al. Citation2019). Therefore, NGS-PGT should be performed by specially trained technical personnel, and for every step of NGS, quality control metrics should be established for optimizing sample quality (Carvalho et al. Citation2020). In this study, two embryos of Family 3 experienced WGA failure; therefore, no PGT results were obtained. We did not perform a rebiopsy on these two embryos as Family 3 obtained transferable embryos, one of which qualified for HSCT. However, if the remaining embryos had not been transferable, a rebiopsy may have been considered. Recent studies have indicated that rebiopsy does not negatively impact pregnancy outcomes (Neal et al. Citation2019; Priner et al. Citation2019).
Patients with dual β-thalassemia mutations have β-thalassemia major and often cannot survive to child-bearing age. Patients with two types of α-thalassemia mutations, however, can experience mild-to-moderate anemia or hemoglobin H (HbH) disease, thereby having a longer life expectancy and reproductive capability than those with dual β-thalassemia mutations. When couples are carriers of α- and β-double thalassemia with a single α-thalassemia mutation, such as these three families, the probability of achieving a transferable embryo is 0.75 × 0.75 = 56.25%. In our study, the probability was close to the theoretical value at 51.5% (17/33). However, if one partner of each couple was HbH or carried two types of α-thalassemia mutations, the probability would decrease to 0.5 × 0.75 = 37.5%. Certainly, the probability would reduce by a quarter when combined with HLA typing, further declining when combined with PGT-A. For example, when combining PGT for two types of thalassemia and aneuploidy screening, Family 2 achieved no transferable embryo. To provide a large number of embryos for biopsy, poor responders generally need to undergo several ovarian stimulation cycles. If no blastocyst with matched HLA is obtained, patients may choose to transfer unaffected euploid embryos or reenter new PGT cycles.
In summary, our study supports the view that even in the case of limited DNA samples, NGS-SNP combined with appropriate WGA technology can achieve at least quadruple purpose testing in one PGT cycle. Moreover, this method presents more efficient and reliable outcomes than previous PCR and STR methods. However, considering the small sample size of our study, the clinical efficacy of our protocol must be investigated in larger-scale research.
Materials and methods
OPU cycle
We performed standard ovarian stimulation, OPU, ICSI, and embryo culture for each of the three families, as previously reported (Xu et al. Citation2009).
Embryo biopsy and blastocyst vitrification
The zona pellucida of unhatched day-5 blastocysts was laser-drilled to create an 18–20 mm hole. Blastocysts qualified for biopsy once several trophectoderm cells hatched from the zona pellucida on day 5 or 6. With the assistance of the OctaxShot™ laser system, we retrieved 5–10 trophectoderm cells using a biopsy pipette with a 30-μm internal diameter. Using the Kitazato vitrification kit (KitazatoBiopharma Co. Ltd., Shizuoka, Japan), we administered vitrification to biopsied blastocysts.
WGA and NGS
After three washes, biopsied trophectoderm cells were treated with MDA using the REPLI-g single-cell kit (Qiagen, Germany), followed by PGT of α- and β-thalassemia and HLA typing using NGS (Ion Torrent™).
The location of α- and β-thalassemia mutations was identified using the Database of Human Hemoglobin Variants and Thalassemia Mutations (http://globin.cse.psu.edu/cgi-bin/hbvar/counter). To establish α-globin gene haplotypes, we selected 143 SNPs mapped upstream, and 100 SNPs mapped 2 M downstream, of the SEA region. This region comprises the HBA1 (NM_000558.4 chr16:215400–234700) and HBA2 genes (NM_000517.4 chr16: 223,300 to 227,103). Meanwhile, 60 to 190 SNPs flanking the HBB gene (NM_000518.4 chr11:5246696–5248301) were used to establish β-globin gene haplotypes.
For HLA typing, the following five target areas were selected: the upstream region of HLA-A (NM_002116.7 chr6:29910247–29913661), the region between HLA-A and HLA-B (NM_005514.6 chr6: 31321649–31324989), the region between HLA-B and HLA-DRA (NM_019111.4 chr6: 32407619–32412823), the region between HLA-DRA and HLA-DQB1 (NM_002123.4 chr6: 32627241–32634466), and the upstream region of HLA-DQB1. A total of 197 to 294 high-density SNPs distributed in these five areas were selected as genetic markers.
All primers were designed using the Ion AmpliSeq™ Designer. Amplified DNA was subjected to DNA purification using Agencourt AMPure XP (Beckman Coulter, Inc.). Following this, DNA libraries were prepared using the Ion AmpliSeq™ library kit (Thermo Fisher Scientific, Inc.). Thereafter, sequencing was performed using the Ion Torrent Personal Genome Machine (Thermo Fisher Scientific, Inc.), according to the manufacturer’s instructions. Additionally, using the same NGS-based SNP haplotyping protocol, a pedigree analysis was conducted on the couples’ gDNA and probands.
To ensure euploid embryo transfer, we performed NGS-based PGT-A in the same PGT cycle, with a sequencing depth ranging from 0.03× to 0.1 ×. Furthermore, data analyses were performed by Jabrehoo Med Tech Co., Ltd.
Embryo transfer
Transferable embryos were defined as both unaffected (unaffected non-carriers or carriers) and euploid; only embryos with matched HLA types to affected probands (HLA-matched) qualified for HSCT purposes. For transferable embryos, we performed a standard FET. On day 14 after FET, the serum β-human chorionic gonadotropin level was measured to determine pregnancy. When a fetal heartbeat was detected via ultrasound on day 42 after FET, clinical pregnancy was confirmed. At 18 weeks of gestation, we performed amniocentesis for prenatal diagnosis of the thalassemia gene and HLA.
Ethics approval
The Research Ethics Committee of the First Affiliated Hospital of Sun Yat-sen University approved our study. Written consent from three families was obtained before the study commenced.
Author contributions
Protocol design: DC, XS, YX; Patient counseling: YX; Execution: XS, YX, CD, QY; Manuscript preparation: DC, XS; Manuscript revision: Y-WX, YZ, CZ; Supervision: CZ.
Supplemental_Table_1.docx
Download MS Word (40.1 KB)Acknowledgments
The authors thank Taylor & Francis Editing Services for providing the English polish.
Disclosure statement
The authors report no conflicts of interest.
Supplementary material
Supplemental data for this article can be accessed here.
Additional information
Funding
References
- Backenroth D, Zahdeh F, Kling Y, Peretz A, Rosen T, Kort D, Zeligson S, Dror T, Kirshberg S, Burak E, et al. 2019. Haploseek: a 24-hour all-in-one method for preimplantation genetic diagnosis (PGD) of monogenic disease and aneuploidy. Genet Med. 21(6):1390–1399. doi:10.1038/s41436-018-0351-7
- Carapito R, Radosavljevic M, Bahram S. 2016. Next-generation sequencing of the HLA locus: methods and impacts on HLA typing, population genetics and disease association studies. Hum Immunol. 77(11):1016–1023. doi:10.1016/j.humimm.2016.04.002.
- Carvalho F, Moutou C, Dimitriadou E, Dreesen J, Gimenez C, Goossens V, Kakourou G, Vermeulen N, Zuccarello D, De Rycke M. 2020. ESHRE PGT Consortium good practice recommendations for the detection of monogenic disorders. Hum Reprod Open. 2020(3):hoaa018. doi:10.1093/hropen/hoaa018.
- Chen L, Diao Z, Xu Z, Zhou J, Yan G, Sun H. 2017. The clinical application of NGS-based SNP haplotyping for PGD of Hb H disease. Syst Biol Reprod Med. 63(3):212–217. doi:10.1080/19396368.2017.1296501.
- Chen SC, Xu XL, Zhang JY, Ding GL, Jin L, Liu B, Sun DM, Mei CL, Yang XN, Huang HF, et al. 2016. Identification of PKD2 mutations in human preimplantation embryos in vitro using a combination of targeted next-generation sequencing and targeted haplotyping. Sci Rep. 6:25488. doi:10.1038/srep25488.
- Cimadomo D, Rienzi L, Romanelli V, Alviggi E, Levi-Setti PE, Albani E, Dusi L, Papini L, Livi C, Benini F, et al. 2018. Inconclusive chromosomal assessment after blastocyst biopsy: prevalence, causative factors and outcomes after re-biopsy and re-vitrification. A multicenter experience. Hum Reprod. 33(10):1839–1846. doi:10.1093/humrep/dey282
- de Bourcy CF, De Vlaminck I, Kanbar JN, Wang J, Gawad C, Quake SR. 2014. A quantitative comparison of single-cell whole genome amplification methods. PLoS One. 9(8):e105585. doi:10.1371/journal.pone.0105585.
- Farashi S, Harteveld CL. 2018. Molecular basis of α-thalassemia. Blood Cells Mol Dis. 70:43–53. doi:10.1016/j.bcmd.2017.09.004.
- Fiorentino F, Biricik A, Bono S, Spizzichino L, Cotroneo E, Cottone G, Kokocinski F, Michel CE. 2014. Development and validation of a next-generation sequencing-based protocol for 24-chromosome aneuploidy screening of embryos. Fertil Steril. 101(5):1375–1382. doi:10.1016/j.fertnstert.2014.01.051.
- Fiorentino F, Biricik A, Karadayi H, Berkil H, Karlikaya G, Sertyel S, Podini D, Baldi M, Magli MC, Gianaroli L, et al. 2004. Development and clinical application of a strategy for preimplantation genetic diagnosis of single gene disorders combined with HLA matching. Mol Hum Reprod. 10(6):445–460. doi:10.1093/molehr/gah055
- Fiorentino F, Kahraman S, Karadayi H, Biricik A, Sertyel S, Karlikaya G, Saglam Y, Podini D, Nuccitelli A, Baldi M. 2005. Short tandem repeats haplotyping of the HLA region in preimplantation HLA matching. Eur J Hum Genet. 13(8):953–958. doi:10.1038/sj.ejhg.5201435.
- Gueye NA, Jalas C, Tao X, Taylor D, Scott RT, Treff NR. 2014. Improved sensitivity to detect recombination using qPCR for Dyskeratosis congenita PGD. J Assist Reprod Genet. 31(9):1227–1230. doi:10.1007/s10815-014-0298-9.
- Handyside AH, Harton GL, Mariani B, Thornhill AR, Affara N, Shaw MA, Griffin DK. 2010. Karyomapping: a universal method for genome wide analysis of genetic disease based on mapping crossovers between parental haplotypes. J Med Genet. 47(10):651–658. doi:10.1136/jmg.2009.069971.
- Hao Y, Chen D, Zhang Z, Zhou P, Cao Y, Wei Z, Xu X, Chen B, Zou W, Lv M, et al. 2018. Successful preimplantation genetic diagnosis by targeted next-generation sequencing on an ion torrent personal genome machine platform. Oncol Lett. 15(4):4296–4302.
- Harper JC, Wilton L, Traeger-Synodinos J, Goossens V, Moutou C, SenGupta SB, Pehlivan Budak T, Renwick P, De Rycke M, Geraedts JP, et al. 2012. The ESHRE PGD Consortium: 10 years of data collection. Hum Reprod Update. 18(3):234–247. doi:10.1093/humupd/dmr052
- Hou W, Xu Y, Li R, Song J, Wang J, Zeng Y, Pan J, Zhou C, Xu Y. 2019. Role of aneuploidy screening in preimplantation genetic testing for monogenic diseases in young women. Fertil Steril. 111(5):928–935. doi:10.1016/j.fertnstert.2019.01.017.
- Ji X, Zhang Z, Shi J, He B. 2019. Clinical application of NGS-based SNP haplotyping for the preimplantation genetic diagnosis of primary open angle glaucoma. Syst Biol Reprod Med. 65(3):258–263. doi:10.1080/19396368.2019.1590479.
- Kakourou G, Vrettou C, Kattamis A, Destouni A, Poulou M, Moutafi M, Kokkali G, Pantos K, Davies S, Kitsiou-Tzeli S, et al. 2016. Complex preimplantation genetic diagnosis for beta-thalassaemia, sideroblastic anaemia, and human leukocyte antigen (HLA)-typing. Syst Biol Reprod Med. 62(1):69–76. doi:10.3109/19396368.2015.1100692
- Kakourou G, Vrettou C, Moutafi M, Traeger-Synodinos J. 2017. Pre-implantation HLA matching: the production of a Saviour Child. Best Pract Res Clin Obstet Gynaecol. 44:76–89. doi:10.1016/j.bpobgyn.2017.05.008.
- Kuliev A, Rechitsky S, Verlinsky O, Ivakhnenko V, Evsikov S, Wolf G, Angastiniotis M, Georghiou D, Kukharenko V, Strom C, et al. 1998. Preimplantation diagnosis of thalassemias. J Assist Reprod Genet. 15(5):219–225. doi:10.1023/A:1022571822585
- Lai K, Huang G, Su L, He Y. 2017. The prevalence of thalassemia in mainland China: evidence from epidemiological surveys. Sci Rep. 7(1):920. doi:10.1038/s41598-017-00967-2.
- Lee VCY, Chow JFC, Yeung WSB, Ho PC. 2017. Preimplantation genetic diagnosis for monogenic diseases. Best Pract Res Clin Obstet Gynaecol. 44:68–75. doi:10.1016/j.bpobgyn.2017.04.001.
- Liang ST, Wong VC, So WW, Ma HK, Chan V, Todd D. 1985. Homozygous alpha-thalassaemia: clinical presentation, diagnosis and management. A review of 46 cases. Br J Obstet Gynaecol. 92(7):680–684. doi:10.1111/j.1471-0528.1985.tb01447.x.
- Mettananda S, Higgs DR. 2018. Molecular basis and genetic modifiers of thalassemia. Hematol Oncol Clin North Am. 32(2):177–191. doi:10.1016/j.hoc.2017.11.003.
- Neal SA, Sun L, Jalas C, Morin SJ, Molinaro TA, Scott RT Jr. 2019. When next-generation sequencing-based preimplantation genetic testing for aneuploidy (PGT-A) yields an inconclusive report: diagnostic results and clinical outcomes after re biopsy. J Assist Reprod Genet. 36(10):2103–2109. doi:10.1007/s10815-019-01550-6.
- Origa R. 2017. β-thalassemia. Genet Med. 19(6):609–619. doi:10.1038/gim.2016.173.
- Priner S, Altarescu G, Schonberger O, Holzer H, Rubinstein E, Dekel N, Peretz A, Eldar-Geva T. 2019. The effect of repeated biopsy on pre-implantation genetic testing for monogenic diseases (PGT-M) treatment outcome. J Assist Reprod Genet. 36(1):159–164. doi:10.1007/s10815-018-1359-2.
- Rafati M, Akhondi MM, Sadeghi MR, Tara SZ, Ghaffari SR. 2018. Preimplantation high-resolution HLA sequencing using next generation sequencing. Biol Blood Marrow Transplant. 24(8):1575–1580. doi:10.1016/j.bbmt.2018.03.024.
- Rechitsky S, Pakhalchuk T, San Ramos G, Goodman A, Zlatopolsky Z, Kuliev A. 2015. First systematic experience of preimplantation genetic diagnosis for single-gene disorders, and/or preimplantation human leukocyte antigen typing, combined with 24-chromosome aneuploidy testing. Fertil Steril. 103(2):503–512. doi:10.1016/j.fertnstert.2014.11.007.
- Shen X, Xu Y, Zhong Y, Zhou C, Zeng Y, Zhuang G, Ding C, Li T. 2011. Preimplantation genetic diagnosis for α-and β-double thalassemia. J Assist Reprod Genet. 28(10):957–964. doi:10.1007/s10815-011-9598-5.
- Shen XT, Xu YW, Zhong YP, Zeng YH, Wang J, Ding CH, Xing WJ, Zhou CQ. 2013. Combination of multiple displacement amplification with short tandem repeat polymorphism in preimplantation genetic diagnosis. Beijing Da Xue Xue Bao Yi Xue Ban. 45(6):852–858.
- Sullivan-Pyke C, Dokras A. 2018. Preimplantation genetic screening and preimplantation genetic diagnosis. Obstet Gynecol Clin North Am. 45(1):113–125. doi:10.1016/j.ogc.2017.10.009.
- Thein SL. 2018. Molecular basis of β thalassemia and potential therapeutic targets. Blood Cells Mol Dis. 70:54–65. doi:10.1016/j.bcmd.2017.06.001.
- Verlinsky Y, Rechitsky S, Schoolcraft W, Strom C, Kuliev A. 2001. Preimplantation diagnosis for Fanconi anemia combined with HLA matching. JAMA. 285(24):3130–3133. doi:10.1001/jama.285.24.3130.
- Vrettou C, Kakourou G, Mamas T, Traeger-Synodinos J. 2018. Prenatal and preimplantation diagnosis of hemoglobinopathies. Int J Lab Hematol. 40(Suppl. 1):74–82. doi:10.1111/ijlh.12823.
- Weatherall DJ, Clegg JB. 2001. Inherited haemoglobin disorders: an increasing global health problem. Bull World Health Organ. 79(8):704–712.
- Xu Y, Chen S, Yin X, Shen X, Pan X, Chen F, Jiang H, Liang Y, Wang W, Xu X, et al. 2015. Embryo genome profiling by single-cell sequencing for preimplantation genetic diagnosis in a β -thalassemia family. Cli Chem. 61(4):617–626. doi:10.1373/clinchem.2014.228569
- Xu YW, Zeng YH, Deng J, Liu Y, Gao L, Zhou CQ, Zhuang GL. 2009. Preimplantation genetic diagnosis for alpha-thalassaemia in China. J Assist Reprod Gen. 26(7):399–403. doi:10.1007/s10815-009-9336-4.