ABSTRACT
Low birth weight (LBW) babies are associated with neonatal morbidity and mortality and are at increased risk for noncommunicable diseases (NCDs) in later life. However, the molecular determinants of LBW are not well understood. Placental insufficiency/dysfunction is the most frequent etiology for fetal growth restriction resulting in LBW and placental epigenetic processes are suggested to be important regulators of pregnancy outcome. Early life exposures like altered maternal nutrition may have long-lasting effects on the health of the offspring via epigenetic mechanisms like DNA methylation and microRNA (miRNA) regulation. miRNAs have been recognized as major regulators of gene expression and are known to play an important role in placental development. Angiogenesis in the placenta is known to be regulated by transcription factor peroxisome proliferator-activated receptor (PPAR) which is activated by ligands such as long-chain-polyunsaturated fatty acids (LCPUFA). In vitro studies in different cell types indicate that fatty acids can influence epigenetic mechanisms like miRNA regulation. We hypothesize that maternal fatty acid status may influence the miRNA regulation of PPAR genes in the placenta in women delivering LBW babies. This review provides an overview of miRNAs and their regulation of PPAR gene in the placenta of women delivering LBW babies.Abbreviations: AA - Arachidonic Acid; Ago2 - Argonaute2; ALA - Alpha-Linolenic Acid; ANGPTL4 - Angiopoietin-Like Protein 4; C14MC - Chromosome 14 miRNA Cluster; C19MC - Chromosome 19 miRNA Cluster; CLA - Conjugated Linoleic Acid; CSE - Cystathionine γ-Lyase; DHA - Docosahexaenoic Acid; EFA – Essential Fatty Acids; E2F3 - E2F transcription factor 3; EPA - Eicosapentaenoic Acid; FGFR1 - Fibroblast Growth Factor Receptor 1; GDM - Gestational Diabetes Mellitus; hADMSCs - Human Adipose Tissue-Derived Mesenchymal Stem Cells; hBMSCs - Human Bone Marrow Mesenchymal Stem Cells; HBV - Hepatitis B Virus; HCC - Hepatocellular Carcinoma; HCPT - Hydroxycamptothecin; HFD – High-Fat Diet; Hmads - Human Multipotent Adipose-Derived Stem; HSCS - Human Hepatic Stellate Cells; IUGR - Intrauterine Growth Restriction; LA - Linoleic Acid; LBW - Low Birth Weight; LCPUFA – Long-Chain Polyunsaturated Fatty Acids; MEK1 - Mitogen-Activated Protein Kinase 1; MiRNA - MicroRNA; mTOR - Mammalian Target of Rapamycin; NCDs - NonCommunicable Diseases; OA - Oleic Acid; PASMC - Pulmonary Artery Smooth Muscle Cell; PLAG1 - Pleiomorphic Adenoma Gene 1; PPAR - Peroxisome Proliferator-Activated Receptor; PPARα - PPAR alpha; PPARγ - PPAR gamma; PPARδ - PPAR delta; pre-miRNA - precursor miRNA; RISC - RNA-Induced Silencing Complex; ROS - Reactive Oxygen Species; SAT - Subcutaneous Adipose Tissue; WHO - World Health Organization
Introduction
Low birth weight (LBW), defined as the weight of babies less than 2500 g at birth, affects 15% to 20% of all births worldwide. As per the World Health Organization (WHO), globally in a year, more than 20 million babies are born LBW (WHO Citation2014). Increased perinatal mortality and morbidity, as well as risk of noncommunicable diseases in later life, are often reported to be associated with LBW (Alexander et al. Citation2014). LBW can be due to preterm birth or intrauterine growth restriction (IUGR) (Valero De Bernabé et al. Citation2004). Fetal growth is largely dependent on the placenta which is exposed to a number of changes in the maternal compartment like altered nutrition and insufficient uteroplacental blood flow (Brett et al. Citation2014). It has been reported that IUGR and preterm births are associated with abnormal placental vascularization (Junaid et al. Citation2014; Kim et al. Citation2003; Sundrani et al. Citation2014). Increasing evidence suggests that alterations in placental epigenetic patterns are associated with improper placental development and maternal-fetal exchange resulting in aberrant fetal growth (Koukoura et al. Citation2012).
Epigenetic regulation controls gene transcription either by methylation of DNA and histone modifications or by noncoding RNAs like microRNAs (miRNA). miRNAs are small noncoding single-stranded RNAs that inhibit the target expression either by mRNA degradation or translational repression (Morales Prieto and Markert Citation2011). They are known to participate in all essential cell processes and regulate almost 30–80% of the genes in the genome. miRNAs are differentially expressed in many tissues and are influenced by a number of external factors and disease conditions (Maccani et al. Citation2010; Nana-Sinkam Citation2014; Boon Citation2015; Issler and Chen Citation2015). They are reported to be expressed in the placenta (Östling et al. Citation2019) and are suggested to be crucial regulators of placental development since, they are involved in trophoblast cell invasion, migration and angiogenesis (Hayder et al. Citation2018).
Various growth factors and transcription factors are known to regulate the process of placental development. Peroxisome proliferator-activated receptors (PPAR) belonging to the ligand-activated nuclear hormone receptor family are transcription factors, critical for placental angiogenesis and development (Fournier et al. Citation2007; Biscetti et al. Citation2008; Parast et al. Citation2009; Nadra et al. Citation2010). These transcription factors are activated by natural ligands like polyunsaturated fatty acids (PUFA) and are involved in a number of cellular and molecular processes (Jump Citation2002, Citation2008). PPAR has the capability to accommodate and bind a variety of natural and synthetic lipophilic acids, such as essential fatty acids (EFA). Along with EFA, various eicosanoids are natural ligands of PPAR (Grygiel-Górniak Citation2014).
Reviews and studies have independently examined the influence of nutrients like fatty acids, vitamins (folate, vitamin D and vitamin E), minerals (selenium and zinc), proteins, as well as dietary flavonols on the miRNA expression in different in vivo and in vitro models (animal models, cell culture and humans) (Ross and Davis Citation2011; Burdge and Lillycrop Citation2014; Yang et al. Citation2014; Xu et al. Citation2014; Köpke et al. Citation2015; van Dijk et al. Citation2016; Boddicker et al. Citation2016; Quintanilha et al. Citation2017; Cui et al. Citation2017; Park et al. Citation2019). We have earlier demonstrated lower maternal and placental LCPUFA levels particularly omega-3 fatty acids (DHA) in women delivering LBW babies (Meher et al. Citation2016a). It is likely that these alterations in maternal fatty acids may influence the expression of miRNAs targeting key genes involved in placental development. In our earlier study on women delivering LBW babies, placental PPAR gamma (PPARγ) mRNA levels were lower and associated with placental DHA and baby weight. (Meher et al. Citation2016b). However, the underlying molecular mechanism associated with these associations is not clear. We propose the hypothesis that altered maternal fatty acid status is associated with disturbed placental development possibly via alterations in the miRNA regulation of PPAR genes in women delivering LBW babies. The current review provides an overview of the role of miRNAs in placental development and implications of the proposed hypothesis.
MicroRNAs (miRNA) and their biogenesis
MicroRNAs (miRNAs) are single-strand small noncoding RNA molecules of 20 to 24 nucleotides that work as epigenetic gene expression regulators. miRNAs are known to be important regulators of post-transcriptional events by binding to the mRNA thereby leading to its degradation or inhibiting the process of protein synthesis.
The miRNA genes are mostly intragenic, i.e., present within the introns and few miRNA genes are present in the exons of protein-coding genes (de Rie et al. Citation2017). These miRNA genes undergo a multistep regulatory process to form a mature functional miRNA. The majority of the miRNAs follow the common biogenesis pathway (Kim et al. Citation2016) that involves the following steps. In the nucleus, the miRNA gene is transcribed to form the primary miRNA (pri-miRNA) with the help of the RNA polymerase II (Lee et al. Citation2004). This pri-miRNA has self-complementary sequences and therefore it folds back to form a hairpin like structure. The pri-miRNA then undergoes endonucleolytic cleavage by the enzyme Drosha (RNA endonuclease III) and RNA-binding protein DGCR8 to form a stem-loop structure, i.e., precursor miRNA (pre-miRNA) (Lee et al. Citation2002; Denli et al. Citation2004). The pre-miRNA is then transported to the cytoplasm by the protein exportin 5 (Perron and Provost Citation2009) and undergoes endonucleolytic cleavage by the enzyme Dicer (RNA endonuclease III) and RNA-binding protein TPBP to form the mature form of miRNA duplex (Grishok et al. Citation2001; Hutvagner et al. Citation2001). This miRNA duplex then binds to the RNA-induced silencing complex (RISC) containing the protein Argonaute2 (Ago2) and other regulatory proteins (Sontheimer Citation2005). The miRNA duplex then unwinds and the strand with the lowest internal stability at the 5ʹ end, known as the guide strand is retained to form the mature RISC complex. The other strand called the passenger strand is discarded (Khvorova et al. Citation2003). The mature miRNA RISC complex then binds to the target mRNA resulting in translational repression or mRNA degradation depending on the degree of complementary sequence similarity.
miRNA and placenta
The placenta is a transient organ that forms a connection between the mother and the fetus and helps in the exchange of nutrients, gases and waste products between the two compartments. The human placenta is said to express more than 600 miRNAs (Chen and Wang Citation2013) and exhibit a specific miRNA expression profile (Mouillet et al. Citation2011a). miRNA regulation is suggested to be one of the regulatory mechanisms by which in utero exposures alter containing placental development and function (Carreras-Badosa et al. Citation2017). Normal placental development involves trophoblast proliferation, invasion and migration. Lately, a number of studies discussed the emerging role of miRNAs in pathways associated with trophoblast function (Ali et al. Citation2020).
A study in mice (Cheloufi et al. Citation2010) and an in vitro study in placental trophoblast cells (Forbes et al. Citation2012) indicate that inactivation of miRNA machinery is associated with abnormal placental development and altered cytotrophoblast proliferation thereby indicating the important role of miRNAs in placental growth and development (Frazier et al. Citation2020). It has been demonstrated that several miRNAs are upregulated or downregulated in the placenta thereby influencing placental development and function (Chen and Wang Citation2013).
Human trophoblasts express unique miRNAs, termed as ‘trophomiRs’, that have specialized functions during pregnancy (Sadovsky et al. Citation2015). Evidence from a number of in vitro studies using immortalized trophoblast and choriocarcinoma cell lines, primary cultures of trophoblasts, animal models and human studies indicates that miRNAs are involved in regulating trophoblast differentiation, migration, invasion, proliferation, apoptosis, vasculogenesis/angiogenesis and cellular metabolism, thereby playing an important role in placental growth and development (Hayder et al. Citation2018; Hu and Zhang Citation2019). It is suggested that there is a need to develop methods to identify placental miRNAs particularly specific to placental cells that may help to use miRNAs for treatment of placental disorders (Frazier et al. Citation2020). Thus, understanding the functions of miRNAs in the placenta can significantly improve our understanding of the molecular mechanisms associated with placental pathologies.
miRNA and low birth weight
Birth weight is known to be influenced by placental health and function (Roland et al. Citation2012), thereby affecting the health of the newborn and increasing the risk of diseases in later life. It is suggested that placental dysfunction is a major cause of fetal growth restriction (Krishna and Bhalerao Citation2011). Therefore, factors determining fetal growth and the underlying molecular mechanisms are important and worthy of attention.
The human placental villous trophoblasts express a large number of miRNAs and their genes are present in two large clusters, the chromosome 14 miRNA cluster (C14MC) and the chromosome 19 miRNA cluster (C19MC) (Morales-Prieto et al. Citation2012). The C19MC cluster is primate-specific and is expressed exclusively in the placenta with most of the miRNAs found in the exosomes released from human trophoblast cells thereby playing an important role in placental-maternal signalling (Bentwich et al. Citation2005; Zhang et al. Citation2008; Donker et al. Citation2012). This cluster is of about 100 kb in size on the human chromosome 19 and contains 46 intragenic miRNA genes that give rise to 58 miRNAs (Bortolin-Cavaillé et al. Citation2009). C19MC miRNAs are reported to be expressed in higher levels in the villous trophoblasts as compared to extravillous trophoblasts and are involved in the migration and invasion of trophoblast cells (Xie et al. Citation2014).
Studies report that different miRNAs are altered in placenta from fetal growth-restricted pregnancies and are discussed below. A genome-wide expression profile study in placental tissue indicates that a number of miRNAs involved in collagen and growth factor signalling are associated with the birth weight of the infant (Payton et al. Citation2020). Another genome-wide analysis study in IUGR placenta reports 37 differentially expressed miRNAs of which 4 belong to the C19MC (520a-3p, 520 f-5p, 515–5p, 519–5p) and 6 belong to the C14MC (299–3p, 494–3p, 376a-5p, 382–3p, 154–3p, 369–3p) that are involved in the regulation of cell migration and proliferation (Awamleh et al. Citation2019). Lower expression of several miRNAs like miR-515-5p, miR-516b, miR-518b, miR-519d, miR-520 h, miR-526b, miR-1323 from the chromosome 19 miRNA cluster and miR-194 has been shown to be associated with fetal growth restriction (Higashijima et al. Citation2013; Guo et al. Citation2013; Hromadnikova et al. Citation2015b). Similarly, a study by Wang et al. reports that the expression of miR-518b is decreased in IUGR cases and expression of another miRNA involved in placental functions, miR-519a, is increased in IUGR cases (Wang et al. Citation2014). Taken together, these studies suggest that the altered expression of these miRNAs is associated with disturbed placental trophoblast function leading to fetal growth restriction.
Human studies have demonstrated low placental expression of miR-16 and miR-21 (involved in the regulation of cell growth and developmental pathways) in women delivering low birth weight babies at term (Maccani et al. Citation2011). In contrast, a study in growth-restricted pregnancies at 32 weeks of gestation reported increased placental expression of miR-21 which negatively regulates the cystathionine γ-lyase (CSE) gene involved in the endogenous production of hydrogen sulfide, a placental vasodilator, thereby resulting in increased vascular resistance (Cindrova-Davies et al. Citation2013). It is likely that this difference could be associated with physiological effects seen at different gestational ages. Further, it is suggested that supplementation of vitamin B12 to pregnant Wistar rats up-regulates the placental expression of miR-16 and miR-21 thereby improving birthweight (Shah et al. Citation2017).
It has been reported that increased placental expression of miRNAs, miR-424 and miR-141 contributes to the pathogenesis of fetal growth restriction by targeting genes mitogen-activated protein kinase 1 (MEK1) and fibroblast growth factor receptor 1 (FGFR1) by miR-424 (Huang et al. Citation2013) and E2F transcription factor 3 (E2F3) and pleiomorphic adenoma gene 1 (PLAG1) by miR-141 (Tang et al. Citation2013). Increased maternal serum and placental miR-517a expression has been observed in pregnancies with LBW newborns. It is suggested that this overexpression of miR-517a is involved in inhibition of trophoblast invasion thereby leading to LBW in the newborn (Song et al. Citation2013). MiR-424, a hypoxia-regulated miRNA has been shown to be upregulated in fetal growth-restricted placenta and is suggested to be associated with the pathogenesis of fetal growth restriction (Huang et al. Citation2013) by regulating the trophoblast-derived cell line proliferation and invasion (Zou et al. Citation2019).
Further, increased expression of miR-10b and miR-363 targeting genes associated with angiogenesis and amino acid transport has been reported in IUGR placenta as compared to controls (Thamotharan et al. Citation2017). It is suggested that upregulation of miR-210-3p (involved in trophoblast proliferation and invasion) in IUGR placenta may contribute to impaired placentation (Li et al. Citation2019a). Altered expression of miRNAs associated with cardiovascular and cerebrovascular disease has also been reported in the placenta of IUGR pregnancies (Hromadnikova et al. Citation2015a).
Thus, identifying the miRNAs associated with the progression of fetal growth restriction is critical and this will help in early diagnosis and management of the disease (Chiofalo et al. Citation2017).
Extracellular miRNAs
Recently, studies have shown that miRNAs are released in the extracellular space like plasma and extracellular fluids. These are called as extracellular miRNAs and placental extracellular miRNAs have been detected in the plasma of pregnant women across gestation, that rapidly decline after delivery (Chim et al. Citation2008). These placental extracellular miRNAs are derived primarily from the trophoblast layer, which lines the human placental villi (Mouillet et al. Citation2015). During pregnancy, these extracellular miRNAs mediate a cross-talk between the mother and the feto-placental unit. They are released from the trophoblast layer in two forms: nonvesicular miRNAs that are bound to proteins like Ago2, nucleophosmin1, or high-density lipoproteins or vesicular miRNAs that are packaged within the extracellular vesicles, such as exosomes, microvesicles, and apoptotic bodies (Ouyang et al. Citation2014).
Altered expression of circulating placental miRNAs in maternal blood has been reported in IUGR pregnancies (Tsochandaridis et al. Citation2015) and are suggested to be associated with fetal growth (Rodosthenous et al. Citation2017). Thus, circulating miRNAs that can be easily found in the maternal blood samples are suggested to provide a potential for noninvasive prenatal diagnostic tests.
The expression of placental miRNAs in trophoblasts is reported to be influenced by various environmental factors like hypoxia (Kulshreshtha et al. Citation2007), exposure to bisphenol A (Avissar-Whiting et al. Citation2010) or cigarette smoking (Maccani et al. Citation2010) thereby regulating the expression of genes involved in trophoblast function. Further, miRNA expression is also suggested to be altered in response to maternal nutrient availability (Cui et al. Citation2017). Therefore, the next section discusses nutrients particularly fatty acids influencing miRNA regulation.
Influence of fatty acids on MiRNA regulation in pregnancy
Maternal nutrition during pregnancy and postnatal periods are known to influence developmental processes via changes in the epigenetic mechanisms. Establishment of these epigenetic patterns in the placenta and the fetus have a profound influence on the development of disease and disorders in the offspring in their later life (Li Citation2018).
Evidence suggests that various nutrients both macro – and micro-nutrients can modulate miRNA expression. Animal and in vitro studies indicate that nutrients like folate (Li et al. Citation2019a), vitamin B12 (Adaikalakoteswari et al. Citation2017) and flavonols like quercetin (Park et al. Citation2019) influence the expression of miRNAs. A study in female C57BL/6 mice indicates that maternal omega-3 fatty acid supplementation helps in fetal brown adipogenesis in synergy with miRNA and histone modifications thereby providing long-term health benefits to the offspring (Fan et al. Citation2018). Other studies on mice and calf also indicate that supplementation of one-carbon metabolites like choline and methionine can affect the expression profile of miRNAs in the placenta (Kwan et al. Citation2018) and polymorphonuclear leukocytes (Jacometo et al. Citation2018) respectively. Maternal low-protein diet in mice is also shown to alter liver expression of miRNAs targeting the inflammatory pathway genes thereby influencing the metabolic health in the offspring (Zheng et al. Citation2017).
A study carried out in rats demonstrates that fish oil (containing omega-3 fatty acids) supplementation protects the rat colon from carcinogen-induced miRNA dysregulation (LA et al. Citation2009). A study in pregnant rats reported that maternal intake of different fatty acids from soybean, olive, fish, linseed or palm oil resulted in the genome wide differential expression of miRNAs in the maternal and offspring liver and adipose tissue suggesting that these effectsfor its functions in placental development particularly may help in understanding the long-term changes in the offspring (Casas-Agustench et al. Citation2015). Similarly, another animal study suggests that maternal high-fat diet influences offspring lipid metabolism by altering the expression of hepatic β-oxidation-related genes and miRNA targeting these genes (Benatti et al. Citation2014).
Thus, it is likely that maternal fatty acid status can influence the expression of miRNAs that targets key genes involved in the process of placental development.
Peroxisome proliferator-activated receptors (PPAR) and miRNA in placental development
Peroxisome proliferator-activated receptors (PPAR)
PPARs are nuclear transcription factors that regulate anti-inflammatory, metabolic and tissue developmental processes. Three PPAR isotypes have been identified in mammals: PPAR alpha (PPARα), PPAR delta (PPARδ) and PPAR gamma (PPARγ) (Lee et al. Citation2009). All these isotypes of PPAR (PPARα, PPARδ and PPARγ) are reported to be expressed in the placenta (Fournier et al. Citation2007) and they are suggested to play an important role in placental angiogenesis and development (Nadra et al. Citation2010; Meher et al. Citation2015).
PPARα
Animal studies indicate that PPARα plays a regulatory role in pathways associated with lipid peroxidation, lipid metabolism and production of nitric oxide associated with angiogenesis (Martínez et al. Citation2008, Citation2011). An animal study indicates that maternal obesity decreases placental PPARα expression thereby modulating fatty acid oxidation pathway and maternal supplementation of adiponectin increased the expression of PPARα in the placenta and reversed the negative effects of maternal obesity on placental function (Aye et al. Citation2015). Similarly, maternal adiponectin has been reported to activate placental PPARα in human primary trophoblast cells (Aye et al. Citation2014). In addition, a study in rodent reports that paternal obesity can also affect the expression of PPARα in the placenta particularly in a sex-specific manner. The authors suggest that there is a need to explore more on the effect of paternal obesity on placental function (Binder et al. Citation2015).
PPARδ
PPARδ is also known to play an important role during implantation and decidualization (Ding et al. Citation2003a; Ding et al. Citation2003b). A PPARδ null mice study indicates that maternal and embryonic PPARδ is crucial for successful pregnancy since it connects different processes like implantation, decidualization and placentation (Wang et al. Citation2007). This is supported by another study in the mouse which demonstrates that activation of PPARδ causes changes in the placental metabolic activities thereby influencing placental development during early pregnancy (Ding et al. Citation2014). In contrast, another study in a rat model indicates that a different dosage of the PPARδ agonist induces placental malformation (Nishimura et al. Citation2013). These differences in the studies could be attributed to the different dosages of the PPARδ agonists and different animal models used. Further, a study on pregnant rats injected with inhibitors of PPARγ and PPARδ indicates that PPARγ, PPARδ and mammalian target of rapamycin (mTOR) signalling pathways interact synergistically to regulate early placental development (Roberti et al. Citation2018).
PPARγ
In the human placenta, PPARγ is known to be expressed in the villous and extravillous cytotrophoblast cells, thereby regulating the process of placental development. Considerable evidence indicates that PPARγ plays a role in trophoblast invasion (Fournier et al. Citation2008; Parast et al. Citation2009), differentiation of cytotrophoblast into syncytiotrophoblast (Schaiff et al. Citation2000; Tarrade et al. Citation2001; Capparuccia et al. Citation2002) and develops a vascular network between the maternal and fetal compartments (Parast et al. Citation2009). In addition, PPARγ also plays an important role in trophoblast maturation which is required to establish the maternal-fetal transport (Asami-Miyagishi et al. Citation2004). PPARγ is known for its functions in placental development particularly spatiotemporal regulation of trophoblast cell genes that are involved in trophoblast differentiation (Shalom-Barak et al. Citation2012).
This is supported by a number of animal studies indicating that deficiency of PPARγ is associated with placental abnormalities and fetal demise (Barak et al. Citation1999; Kubota et al. Citation1999; NI et al. Citation2013). The PPARγ gene knockout study by Barak et al. provided the first evidence that PPARγ deficiency affects trophoblast differentiation and placental vascularization thereby resulting in embryonic lethality (Barak et al. Citation1999). In vitro and animal studies indicate that PPARγ regulates angiogenic factors – vascular endothelial growth factor (VEGF) and angiopoietin and hypoxia-inducible factor thereby influencing trophoblast invasion and placental angiogenesis (Garnier et al. Citation2015; Zhang et al. Citation2017). A mouse model of hypoxia during pregnancy resulted in the development of IUGR and reduced placental PPARγ expression. Pharmacological activationof PPAR by a PPARγ agonist, pioglitazone, prevented the hypoxia-induced fetal growth restriction (Lane et al. Citation2019). The authors suggest that this protection of fetal growth restriction could be the due role of PPARγ in regulating placental vascularization. Further, PPARγ is known to have anti-inflammatory effects by inhibiting the production of reactive oxygen species (ROS) and lowering inflammatory markers through down-regulation of NF-kappaB pathway (Zhang et al. Citation2018), thereby mediating placental vascularization. This is supported by studies during pregnancy which demonstrates that PPARγ activation by rosiglitazone reduces the macrophage-mediated pro-inflammatory response, thereby preventing preterm birth (Xu et al. Citation2016) and prevents trophoblast-associated inflammation by inhibition of NF-kappaB (Kadam et al. Citation2019).In vitro studies on extravillous trophoblast cells and placental explants indicate that the heterodimer of PPARγ/RXRα binds to the promoter region of angiopoietin-like protein 4 (ANGPTL4). ANGPTL4 is an angiogenic marker involved in the process of angiogenesis in various tissues and thus is suggested to be a potential target gene of PPARγ (Liu et al. Citation2017). As in vitro study on human placental choriocarcinoma cells suggests that the PPARγ signalling pathway is associated with progesterone regulation thereby influencing fetal growth and development (Hu et al. Citation2017). A study in cultured human term villous cytotrophoblast cells suggests that phthalates affect that transcriptional activity of PPARγ thereby influencing lipid metabolism and differentiation of cytotrophoblast cells (Shoaito et al. Citation2019). Thus, considerable evidence indicates that alterations in the placental expression of PPARγ is associated with placental abnormalities.
Fatty acids and PPARs in pregnancy
Fatty acids are important for the developing fetus and play a critical role in determining the duration of gestation and pregnancy outcome (Cetin et al. Citation2010; Coletta et al. Citation2010). Polyunsaturated fatty acids (PUFA) are classified into two main families based on the presence of the first double bond at the n-6 or n-3 position: omega-6 and omega-3 fatty acids. Linoleic acid (LA) (omega-6) and alpha-linolenic acid (ALA) (omega-3) are the dietary essential fatty acids and precursors of highly unsaturated members of their family, i.e., long-chain polyunsaturated fatty acids (LCPUFA) – arachidonic acid (AA) and docosahexaenoic acid (DHA) respectively (Benatti et al. Citation2004).
Maternal omega-3 fatty acid supplementation during pregnancy has been reported to be associated with length of gestation and birth weight (Larqué et al. Citation2012; Middleton et al. Citation2018). Fatty acids are also known to affect gene expression by regulating transcription factors (Deckelbaum et al. Citation2006). One of the widely studied transcription factor during pregnancy is PPAR.
Evidence from various animal studies suggests that dietary treatments supplemented with monounsaturated and polyunsaturated fatty acids influence the expression of PPARγ, thereby regulating the PPAR signalling (Capobianco et al. Citation2008, Citation2018; Jawerbaum and Capobianco Citation2011; Martinez et al. Citation2012). Different types of maternal dietary fat have been shown to differentially influence the expression of PPAR isoforms. Cows fed LA diets report increased expression of placental PPARα as compared to those fed oleic acid (OA) whereas, PPARγ and PPARδ expression were decreased in the placenta of cows fed with LA compared to cows fed with OA (Salehi and DJ Citation2017).
In vitro studies on human placental trophoblasts indicate that PPARs are involved in the regulation of fatty acid storage and transport (Schaiff et al. Citation2005). Incubation of fatty acids like OA, EPA, DHA, AA, conjugated linoleic acid (CLA) has been shown to increase the expression of PPARγ in placental choriocarcinoma (BeWo) cells (Duttaroy et al. Citation2003).
A study in obese pregnant women reports higher placental PPARγ mRNA and protein expression whereas lower placental PPARα mRNA expression as compared to lean women. It is suggested that this higher PPARγ stimulates lipid esterification and storage in the obese placenta and the lower PPARα expression may influence the fatty acid oxidation in obese placenta (Calabuig-Navarro et al. Citation2017). Another study by the same group reported that supplementation of omega-3 fatty acids during pregnancy lowered the total lipid content and expression of PPARγ and other related genes involved in lipid esterification and storage. In contrast, omega-3 fatty acid supplementation had no significant effect on the expression of PPARα and other genes associated with fatty acid oxidation pathway (Calabuig-Navarro et al. Citation2016).
These observations indicate an important role of fatty acids in regulating key transcription factors like PPAR in the placenta thereby influencing placental lipid metabolism.
placenta
microRNA regulation of PPAR
PPAR genes are suggested to be regulated by epigenetic changes (Lendvai et al. Citation2016; Kitsiou-Tzeli and Tzetis Citation2017; Capobianco et al. Citation2018). Various review articles have summarized that several miRNAs target different forms of PPAR, thereby suggesting their role in the transcriptional regulation of different pathological conditions like metabolic disorders (inflammation and cancer) (Portius et al. Citation2017), mesenchymal stem cell differentiation (Huang et al. Citation2016a) and hepatocellular carcinoma (Hsu and Chi Citation2014).
Evidence from independent review articles and studies in different health conditions has suggested that the different isoforms of PPAR are regulated by different miRNAs summarized in . These studies indicate their regulatory role in various processes like adipogenic differentiation, pulmonary hypertension, vascular dysfunction associated with disease conditions like cardiovascular diseases, heart diseases and obesity-related diseases.
Table 1. MicroRNAs Targeting PPAR
microRNA regulation of PPAR during pregnancy
Studies have reported the role of different miRNAs as epigenetic regulators of PPARs in various tissues and cell lines during pregnancy and are summarized below. An animal study by Sarr et al. indicates that adverse in utero environmental conditions alter the expression of lipid-synthesis genes including PPARγ and miRNAs like miR-24 and miR-103-2 factors in the adipose tissue of IUGR offspring (Sarr et al. Citation2014). Another study using a rat model of gestational diabetes reported increased expression of PPARγ and reduced expression of miR-130 that targets PPARγ in the liver of only male fetuses. In contrast, increased expression of PPARδ and reduced expression of miR-9 that targets PPARδ was observed only in the liver of female fetuses. The authors conclude that there are sex-dependent changes in the miRNAs that targets different isoforms of PPAR in the liver of rats with gestational diabetes (Fornes et al. Citation2018).
It is well known that alterations in the maternal nutrients during pregnancy epigenetically programs the fetus. A rat model of maternal food restriction resulting in intrauterine growth-restricted offspring reported that miRNAs promoting adipogenesis like miR-30d, let-7a and miR-103 were increased in the bone marrow-derived mesenchymal stem cells as compared to control offspring. However, the miRNAs, miR-27 and miR-130 targeting PPARγ were not significantly different. Therefore, it is likely that in these growth-restricted offspring as a result of maternal food restriction, selective adipogenic pathways are activated (Gong et al. Citation2016). Another study in Meishan pigs reported that maternal low-protein diet throughout gestation and lactation increased the expression of miR-130b in the subcutaneous fat of the offspring. Thus, miR-130b targeting PPARγ gene regulates offspring lipid metabolism in response to maternal dietary protein status (Pan et al. Citation2013).
A study in IUGR rat offspring reported that increased expression of miR-29a in the skeletal muscle cell line inhibited the expression of its target gene PPARδ thereby inducing insulin resistance (Zhou et al. Citation2016). Another study by Adaikalakoteswari et al. observed that the expression of miRNAs (miR-27b, miR-23a, miR-130b) targeting PPARγ gene was downregulated in the human adipocyte cell line deficient in vitamin B12. This was also validated in the subcutaneous adipose tissue and maternal serum collected at the delivery of pregnant women deficient in vitamin B12. The authors suggest that miRNAs can regulate the expression of genes involved in the process of adipogenesis (Adaikalakoteswari et al. Citation2017).
The above suggests a miRNA mediated mechanism of PPAR regulation. To the best of our knowledge, there is limited literature on the placenta-specific miRNAs targeting the different PPAR isoforms in pregnancy outcomes. Only one study on women with gestational diabetes mellitus (GDM) has reported that increased placental expression of miRNA-518d is associated with reduced placental PPARα protein expression suggesting that upregulation of miR-518d may be associated with the pathogenesis of GDM (Zhao et al. Citation2014). Therefore, there is a need to explore miRNAs in the human placenta that target different isoforms of PPAR thereby regulating placental development and function.
Implications for women delivering LBW Babies
LBW is a public health problem and India is reported to have the largest number of LBW babies. Alterations in the maternal nutritional status is known to alter placental and fetal growth and development, resulting in LBW babies. These LBW babies are at increased risk of developing NCDs in later life. However, the underlying molecular mechanisms are not clear. Over a decade, growing evidence suggests that epigenetic factors during the early intrauterine, perinatal and postnatal periods can influence the health of the offspring during infancy and later in adult life. These effects may possibly be mediated by epigenetic modifications like miRNA regulation.
Emerging evidence indicates a potential role for nutrients like fatty acids in influencing epigenetic mechanisms. Fatty acids, particularly LCPUFA play an important role during placental as well as fetal growth since they are involved in number physiological processes associated with cellular growth and function. These LCPUFA are ligands for a transcription factor, PPAR and reports indicate that reduced placental PPAR expression is associated with disturbed placental development. Based on this we hypothesize that altered maternal fatty acid status is associated with disturbed placental development possibly via alterations in the miRNA regulation of PPAR genes in women delivering LBW babies (). The literature\described in this review indicates the importance of PPARs in placental development; however, the role of these transcription factors in women delivering LBW essentially remains to be explored. Increased mRNA and protein expression of PPARγ has been reported in the placental tissue of fetal growth-restricted pregnancies as compared to controls (Chui et al. Citation2013). Similarly, another study by Holdsworth–Carson et al. reported increased protein levels of PPARα and PPARγ in placentas from intrauterine growth restriction (IUGR) pregnancies (Holdsworth-Carson et al. Citation2010). However, using immunocytochemistry, a study by Rodie et al. demonstrated no difference in the PPARδ and PPARγ expression in IUGR and control placenta (Rodie et al. Citation2005). Thus, these reports are variable and inconclusive with some showing lower, higher or no change in the levels of PPAR. The discrepancy in these studies could be due to a difference in the methodology used and the difference in patient selection particularly with respect to the mode of delivery or the status of labor. Further, these studies have limited sample size.
Figure 1. Maternal fatty acids epigenetically regulate PPAR expression through miRNA regulation in the placenta thereby influencing fetal outcome. Fatty acids are ligands for transcription factor PPAR, which are expressed in the placenta and are involved in placental angiogenesis and development. Alterations in the maternal fatty acids status can epigenetically regulate PPAR in the placenta by influencing the miRNAs targeting the PPAR gene thereby resulting in defective placentation. This may result into adverse pregnancy outcome like LBW and increased risk for noncommunicable diseases in the offspring.PPAR: Peroxisome Proliferator-Activated Receptor; RISC: RNA-induced Silencing Complex; LBW: Low Birth Weight; NCDs: NonCommunicable Diseases
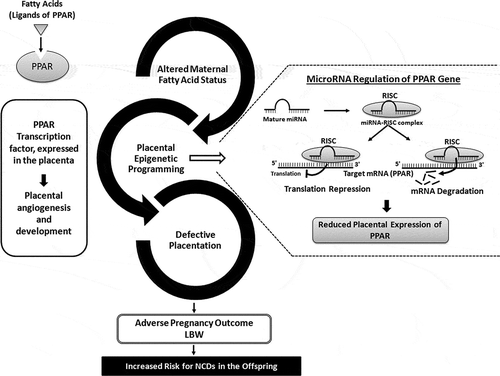
Considering the important role of PPARs in the process of implantation, placental development as well as the initiation of labor, PPAR could be considered as a potential therapeutic target for placental disorders. However, the underlying molecular mechanisms associated with PPAR regulation need to be explored. There are no studies examining the expression of miRNAs targeting PPARs in placenta from women delivering LBW babies and their association with maternal fatty acid status. Elucidation of these regulatory mechanisms will provide a better understanding of the molecular pathways leading to altered fetal growth. This will also identify placental epigenetic markers (differentially regulated miRNAs) associated with LBW. miRNAs are considered as valuable circulating biomarkers and therapeutic targets. The differentially regulated miRNAs identified can be validated in the maternal plasma. This may lead to the identification of epigenetic biomarkers associated with fetal growth during pregnancy. In addition, studies examining the influence of maternal nutrition on miRNA expression and function may provide clues for supplementation to prevent adverse pregnancy outcomes.
To summarize, based on the above literature, investigating the miRNAs regulating fetal growth by targeting the miRNAs regulating transcription factors like PPAR is warranted. Animal studies can help to understand the effect of fatty acid supplementation on the placental expression of transcription factors (PPAR) and miRNAs targeting PPAR. In human studies, the expression of predicted miRNAs regulating PPAR genes can be examined in the placenta of women delivering LBW babies and compared with women delivering normal birth weight (NBW) babies. Further, the association of these miRNAs with maternal and placenta fatty acid status can be examined. In vitro studies on placental trophoblast cells from LBW and NBW samples can also be used. The above-mentioned examinations will provide insights into the molecular mechanisms related to epigenetic programming of diseases in LBW babies.
Disclosure of interest
The authors report no conflict of interest.
Author Contributions
Contributed to the conception and design of the review: DPS, SRJ; wrote the review: DPS, ARK, SRJ. All authors reviewed the final manuscript.
Acknowledgments
The authors acknowledge the Department of Biotechnology (DBT), Government of India for funding the project “Epigenetic regulation of placental peroxisome proliferator activated receptor (PPAR) in women delivering low birth weight babies” (Grant number: BT/PR30592/BIC/101/1081/2018).
Additional information
Funding
References
- Adaikalakoteswari A, Vatish M, Alam MT, Ott S, Kumar S, Saravanan P. 2017. Low Vitamin B12 in Pregnancy Is Associated With Adipose-Derived Circulating miRs Targeting PPARγ and Insulin Resistance. J Clin Endocrinol Metab. 102(11):4200–4209.
- Alexander BT, Henry Dasinger J, Intapad S. 2014. Effect of low birth weight on women's health. Clinical Therapeutics. 36(12):1913–1923 doi:10.1016/j.clinthera.2014.06.026
- Ali A, GJ B, RV A, QA W. 2020. The Role of LIN28-let-7-ARID3B Pathway in Placental Development. Int J Mol Sci. 21(10):3637. doi:10.3390/ijms21103637.
- Asami-Miyagishi R, Iseki S, Usui M, Uchida K, Kubo H, Morita I. 2004. Expression and function of PPARgamma in rat placental development. Biochem Biophys Res Commun. 315(2):497–501. doi:10.1016/j.bbrc.2004.01.074.
- Avissar-Whiting M, Veiga KR, Uhl KM, Maccani MA, Gagne LA, Moen EL, Marsit CJ. 2010. Bisphenol A exposure leads to specific microRNA alterations in placental cells. Reprod Toxicol. 29(4):401–406.
- Awamleh Z, GB G, VKM H. 2019. Placental microRNAs in pregnancies with early onset intrauterine growth restriction and preeclampsia: potential impact on gene expression and pathophysiology. BMC Med Genomics. 12(1):91. doi:10.1186/s12920-019-0548-x.
- Aye IL, Gao X, Weintraub ST, Jansson T, Powell TL. 2014. Adiponectin inhibits insulin function in primary trophoblasts by PPARα-mediated ceramide synthesis. Mol Endocrinol. 28(4):512–524
- Aye Irving L. M. H., Rosario FJ, Powell TL, Jansson T. 2015. Adiponectin supplementation in pregnant mice prevents the adverse effects of maternal obesity on placental function and fetal growth. Proc Natl Acad Sci U S A. 112(41):12858–12863 doi:10.1073/pnas.1515484112
- Barak Y, Nelson MC, ES O, YZ J, Ruiz-Lozano P, KR C, Koder A, RM E. 1999. PPAR gamma is required for placental, cardiac, and adipose tissue development. Mol Cell. 4(4):585–595. doi:10.1016/S1097-2765(00)80209-9.
- Benatti P, Peluso G, Nicolai R, Calvani M. 2004. Polyunsaturated fatty acids: biochemical, nutritional and epigenetic properties. J Am Coll Nutr. 23:281–302.
- Benatti RO, Melo AM, Borges FO, Ignacio-Souza LM, Simino LAP, Milanski M, Velloso LA, Torsoni MA, Torsoni AS. 2014. Maternal high-fat diet consumption modulates hepatic lipid metabolism and microRNA-122 (miR-122) and microRNA-370 (miR-370) expression in offspring. Br J Nutr. 111(12):2112–2122.
- Bentwich I, Avniel A, Karov Y, Aharonov R, Gilad S, Barad O, Barzilai A, Einat P, Einav U, Meiri E, et al.. 2005. Identification of hundreds of conserved and nonconserved human microRNAs. Nat Genet. 37(7):766–770. doi:10.1038/ng1590.
- Bi R, Bao C, Jiang L, Liu H, Yang Y, Mei J, Ding F. 2015. MicroRNA-27b plays a role in pulmonary arterial hypertension by modulating peroxisome proliferator-activated receptor γ dependent Hsp90-eNOS signaling and nitric oxide production. Biochem Biophys Res Commun. 460(2):469–475.
- Binder NK, Beard SA, Kaitu'u-Lino TJ, Tong S, Hannan NJ, Gardner DK. 2015. Paternal obesity in a rodent model affects placental gene expression in a sex-specific manner. Reproduction. 149(5):435–444
- Biscetti F, Gaetani E, Flex A, Aprahamian T, Hopkins T, Straface G, Pecorini G, Stigliano E, RC S, Angelini F, et al.. 2008. Selective activation of peroxisome proliferator-activated receptor (PPAR) alpha and PPAR gamma induces neoangiogenesis through a vascular endothelial growth factor-dependent mechanism. Diabetes. 57(5):1394–1404. doi:10.2337/db07-0765.
- Boddicker RL, Koltes JE, Fritz-Waters ER, Koesterke L, Weeks N, Yin T, Mani V, Nettleton D, Reecy JM, Baumgard LH, et al. 2016. Genome-wide methylation profile following prenatal and postnatal dietary omega-3 fatty acid supplementation in pigs. Anim Genet. 47(6):658–671
- Boon RA, Dimmeler S. 2015. MicroRNAs in myocardial infarction. Nat Rev Cardiol. 12(3):135–142. doi:10.1038/nrcardio.2014.207.
- Bortolin-Cavaillé ML, Dance M, Weber M, Cavaillé J. 2009. C19MC microRNAs are processed from introns of large Pol-II, non-protein-coding transcripts. Nucleic Acids Res. 37(10):3464–3473. doi:10.1093/nar/gkp205.
- Brett KE, Ferraro ZM, Yockell-Lelievre J, Gruslin A, Adamo KB. 2014. Maternal-fetal nutrient transport in pregnancy pathologies: the role of the placenta. Int J Mol Sci. 15: 16153–16185
- Burdge GC, Lillycrop KA. 2014. Fatty acids and epigenetics. Curr Opin Clin Nutr Metab Care. 17(2):156–161. doi:10.1097/MCO.0000000000000023.
- Cai K, Li T, Guo L, Guo H, Zhu W, Yan L, Li F. 2019. Long non-coding RNA LINC00467 regulates hepatocellular carcinoma progression by modulating miR-9-5p/PPARA expression. Open Biol. 9(9):190074. doi:10.1098/rsob.190074.
- Calabuig-Navarro V, Haghiac M, Minium J, Glazebrook P, GC R, Hoppel C, Hauguel De-mouzon S, Catalano P, O’Tierney-Ginn P. 2017. Effect of Maternal Obesity on Placental Lipid Metabolism. Endocrinology. 158(8):2543–2555. doi:10.1210/en.2017-00152.
- Calabuig-Navarro V, Puchowicz M, Glazebrook P, Haghiac M, Minium J, Catalano P, Hauguelde MS, O’Tierney-Ginn P. 2016. Effect of ω-3 supplementation on placental lipid metabolism in overweight and obese women. Am J Clin Nutr. 103(4):1064–1072. doi:10.3945/ajcn.115.124651.
- Capobianco E, Fornes D, SL R, TL P, Jansson T, Jawerbaum A. 2018. Supplementation with polyunsaturated fatty acids in pregnant rats with mild diabetes normalizes placental PPARγ and mTOR signaling in female offspring developing gestational diabetes. J Nutr Biochem. 53:39–47. doi:10.1016/j.jnutbio.2017.10.006.
- Capobianco E, White V, Higa R, Martínez N, Jawerbaum A. 2008. Effects of natural ligands of PPARgamma on lipid metabolism in placental tissues from healthy and diabetic rats. Mol Hum Reprod. 14(8):491–499. doi:10.1093/molehr/gan039.
- Capparuccia L, Marzioni D, Giordano A, Fazioli F, De NM, Busso N, Todros T, Castellucci M. 2002. PPARgamma expression in normal human placenta, hydatidiform mole and choriocarcinoma. Mol Hum Reprod. 8(6):574–579. doi:10.1093/molehr/8.6.574.
- Carreras-Badosa G, Bonmatí A, FJ O, JM M, Guindo-Martinez M, Torrents D, Prats-Puig A, JM M-C, de Zegher F, Ibanez L, et al.. 2017. Dysregulation of Placental miRNA in Maternal Obesity Is Associated With Pre- and Postnatal Growth. J Clin Endocrinol Metab. 102(7):2584–2594. doi:10.1210/jc.2017-00089.
- Casas-Agustench P, Iglesias-Gutiérrez E, Dávalos A. 2015. Mother’s nutritional miRNA legacy: nutrition during pregnancy and its possible implications to develop cardiometabolic disease in later life. Pharmacol Res. 100:322–334. doi:10.1016/j.phrs.2015.08.017.
- Cetin I, Berti C, Calabrese S. 2010. Role of micronutrients in the periconceptional period. Hum Reprod Update. 16(1):80–95. doi:10.1093/humupd/dmp025.
- Chau BN, Xin C, Hartner J, Ren S, Castano AP, Linn G, Li J, Tran PT, Kaimal V, Huang X, et al.. 2012. MicroRNA-21 promotes fibrosis of the kidney by silencing metabolic pathways. Sci Transl Med. 4(121):121ra18.
- Cheloufi S, Dos SCO, MM C, GJ H. 2010. A dicer-independent miRNA biogenesis pathway that requires Ago catalysis. Nature. 465(7298):584–589. doi:10.1038/nature09092.
- Chen C, Xiang H, YL P, Peng J, SW J. 2014a. Mature miR-183, negatively regulated by transcription factor GATA3, promotes 3T3-L1 adipogenesis through inhibition of the canonical Wnt/β-catenin signaling pathway by targeting LRP6. Cell Signal. 26(6):1155–1165. doi:10.1016/j.cellsig.2014.02.003.
- Chen DB, Wang W. 2013. Human placental microRNAs and preeclampsia. Biol Reprod. 88(5):130.
- Chen L, YM D, CB J, Yang L, CM S, GF X, LX P, FY H, CM Z, XR G. 2014b. MiR-146b is a regulator of human visceral preadipocyte proliferation and differentiation and its expression is altered in human obesity. Mol Cell Endocrinol. 393(1–2):65–74. doi:10.1016/j.mce.2014.05.022.
- Chen Q, Qiu F, Zhou K, HG M, Takahashi Y, RVS R, Yang Y, Moran E, JX M. 2017. Pathogenic Role of microRNA-21 in Diabetic Retinopathy Through Downregulation of PPARα. Diabetes. 66(6):1671–1682. doi:10.2337/db16-1246.
- Chen T, Zhang Y, Liu Y, Zhu D, Yu J, Li G, Sun Z, Wang W, Jiang H, Hong Z. 2019. MiR-27a promotes insulin resistance and mediates glucose metabolism by targeting PPAR-γ-mediated PI3K/AKT signaling. Aging (Albany NY). 11(18):7510–7524. doi:10.18632/aging.102263.
- Chi C-W., Hsu H-T.. 2014. Emerging role of the peroxisome proliferator-activated receptor-gamma in hepatocellular carcinoma. Journal of Hepatocellular Carcinoma. 1:127–35 doi:10.2147/JHC
- Chim SS, Shing TK, Hung EC, Leung TK, Lau TK, Chiu RWK, Dennis LYM. 2008. Detection and characterization of placental microRNAs in maternal plasma. Clin Chem. 54(3):482‐490.
- Chiofalo B, Laganà AS, Vaiarelli A, La RVL, Rossetti D, Palmara V, Valenti G, Rapisarda AMC, Granese R, Sapia F, et al.. 2017. Do miRNAs Play a Role in Fetal Growth Restriction? A Fresh Look to a Busy Corner. Biomed Res Int. 2017:6073167. doi:10.1155/2017/6073167.
- Chui A, Kalionis B, Abumaree M, Cocquebert M, Fournier T, Evain-Brion D, SP B, Murthi P. 2013. Downstream targets of the homeobox gene DLX3 are differentially expressed in the placenta of pregnancies affected by human idiopathic fetal growth restriction. Mol Cell Endocrinol. 377(1–2):75–83. doi:10.1016/j.mce.2013.06.032.
- Chuppa S, Liang M, Liu P, Liu Y, Casati MC, AW C, Patullo L, AJ K. 2018. MicroRNA-21 regulates peroxisome proliferator-activated receptor alpha, a molecular mechanism of cardiac pathology in Cardiorenal Syndrome Type 4. Kidney Int. 93(2):375–389. doi:10.1016/j.kint.2017.05.014.
- Cindrova-Davies T, Herrera EA, Niu Y, Kingdom J, Giussani DA, Burton GJ. 2013. Reduced cystathionine γ-lyase and increased miR-21 expression are associated with increased vascular resistance in growth-restricted pregnancies: hydrogen sulfide as a placental vasodilator. Am J Pathol. 182(4):1448–1458.
- Coletta JM, Bell SJ, Roman AS. 2010. Omega-3 Fatty acids and pregnancy. Rev Obstet Gynecol. 3(4):163–171.
- Cui J, Zhou B, SA R, Zempleni J. 2017. Nutrition, microRNAs, and Human Health. Adv Nutr. 8(1):105–112. doi:10.3945/an.116.013839.
- de Rie D, Abugessaisa I, Alam T, Arner E, Arner P, Ashoor H, Åström G, Babina M, Bertin N, AM B, et al.. 2017. An integrated expression atlas of miRNAs and their promoters in human and mouse. Nat Biotechnol. 35(9):872–878. doi:10.1038/nbt.3947.
- Deckelbaum RJ, Worgall TS, Seo T. 2006. n-3 fatty acids and gene expression. Am J ClinNutr. 83(6 Suppl):1520S–25S.
- Denli AM, Tops BB, Plasterk RH, Ketting RF, Hannon GJ. 2004. Processing of primary microRNAs by the Microprocessor complex. Nature. 432: 231–235
- vanDijk SJ, Zhou J, Peters TJ, Buckley M, Sutcliffe B, Oytam Y, Gibson RA, McPhee A, Yelland LN, Makrides M, et al. 2016. Effect of prenatal DHA supplementation on the infant epigenome: results from a randomized controlled trial. Clin Epigenetics. 8:114
- Ding H, Zhang Y, Liu L, Yuan H, Qu J, Shen R. 2014. Activation of peroxisome proliferator activator receptor delta in mouse impacts lipid composition and placental development at early stage of gestation. Biol Reprod. 91(3):57.
- Ding NZ, Ma XH, Diao HL, Xu LB, Yang ZM. 2003a. Differential expression of peroxisome proliferator-activated receptor delta at implantation sites and in decidual cells of rat uterus. Reproduction. 125(6):817–825.
- Ding NZ, Teng CB, Ma H, Ni H, Ma XH, Xu LB, Yang ZM. 2003b. Peroxisome proliferator-activated receptor delta expression and regulation in mouse uterus during embryo implantation and decidualization. Mol Reprod Dev. 66(3):218–224
- Donker RB, JF M, Chu T, CA H, DB S, AE M, Sadovsky Y. 2012. The expression profile of C19MC microRNAs in primary human trophoblast cells and exosomes. Mol Hum Reprod. 18(8):417–424. doi:10.1093/molehr/gas013.
- Duttaroy AK, Crozet D, Taylor J, MJ G. 2003. Acyl-CoA thioesterase activity in human placental choriocarcinoma (BeWo), cells: effects of fatty acids. Prostaglandins Leukot Essent Fatty Acids. 68(1):43–48. doi:10.1016/S0952-3278(02)00234-X.
- El Azzouzi H, Leptidis S, Dirkx E, Hoeks J, van Bree B, Brand K, EA M, Poels E, JC S, van den Hoogenhof MM, et al.. 2013. The hypoxia-inducible microRNA cluster miR-199a∼214 targets myocardial PPARδ and impairs mitochondrial fatty acid oxidation. Cell Metab. 18(3):341–354. doi:10.1016/j.cmet.2013.08.009.
- Fan R, AM T, Jang Y, SH R, Chung S. 2018. Maternal n-3 PUFA supplementation promotes fetal brown adipose tissue development through epigenetic modifications in C57BL/6 mice. Biochim Biophys Acta Mol Cell Biol Lipids. 1863(12):1488–1497. doi:10.1016/j.bbalip.2018.09.008.
- Forbes K, Farrokhnia F, JD A, Westwood M. 2012. Dicer-dependent miRNAs provide an endogenous restraint on cytotrophoblast proliferation. Placenta. 33(7):581–585. doi:10.1016/j.placenta.2012.03.006.
- Fornes D, White V, Higa R, Heinecke F, Capobianco E, Jawerbaum A. 2018. Sex-dependent changes in lipid metabolism, PPAR pathways and microRNAs that target PPARs in the fetal liver of rats with gestational diabetes. Mol Cell Endocrinol. 461:12–21. doi:10.1016/j.mce.2017.08.004.
- Fournier T, Thérond P, Handschuh K, Tsatsaris V, Evain-Brion D. 2008. PPARgamma and early human placental development. Curr Med Chem. 15(28):3011–3024. doi:10.2174/092986708786848677.
- Fournier T, Tsatsaris V, Handschuh K, Evain-Brion D. 2007. PPARs and the placenta. Placenta. 28(2–3):65–76. doi:10.1016/j.placenta.2006.04.009.
- Frazier S, MW M, Mulvana H, Graham D. 2020. From animal models to patients: the role of placental microRNAs, miR-210, miR-126, and miR-148a/152 in preeclampsia. Clin Sci (Lond). 134(8):1001–1025.
- Garnier V, Traboulsi W, Salomon A, Brouillet S, Fournier T, Winkler C, Desvergne B, Hoffmann P, QY Z, Congiu C, et al.. 2015. PPARγ controls pregnancy outcome through activation of EG-VEGF: new insights into the mechanism of placental development. Am J Physiol Endocrinol Metab. 309(4):E357–E369.
- Gatfield D, Le MG, CE V, Gerlach D, Schaad O, Fleury-Olela F, AL R, Oresic M, CC E, EM Z, et al.. 2009. Integration of microRNA miR-122 in hepatic circadian gene expression. Genes Dev. 23(11):1313–1326. doi:10.1101/gad.1781009.
- Gokina NI, Chan S-L., Chapman AC, Oppenheimer K, Jetton TL, Cipolla MJ. 2013. Inhibition of PPARγ during rat pregnancy causes intrauterine growth restriction and attenuation of uterine vasodilation. Front Physiol. 4:184 doi:10.3389/fphys.2013.00184
- Gong M, Antony S, Sakurai R, Liu J, Iacovino M, VK R. 2016. Bone marrow mesenchymal stem cells of the intrauterine growth-restricted rat offspring exhibit enhanced adipogenic phenotype. Int J Obes (Lond). 40(11):1768–1775. doi:10.1038/ijo.2016.157.
- Grishok A, AE P, Conte D, Li N, Parrish S, Ha I, DL B, Fire A, Ruvkun G, CC M. 2001. Genes and mechanisms related to RNA interference regulate expression of the small temporal RNAs that control C. Elegans Developmental Timing Cell. 106(1):23–34. doi:10.1016/S0092-8674(01)00431-7.
- Grygiel-Górniak B. 2014. Peroxisome proliferator-activated receptors and their ligands: nutritional and clinical implications–a review. Nutr J. 13(1):17. doi:10.1186/1475-2891-13-17.
- Gu J-J., Zhang J-H., Chen H-J., Wang S-S.. 2016. MicroRNA-130b promotes cell proliferation and invasion by inhibiting peroxisome proliferator-activated receptor-γ in human glioma cells. International Journal of Molecular Medicine. 37(6):1587–1593 doi:10.3892/ijmm.2016.2580
- Guo L, SQ T, NE H, AH J, AA M-R, Thames B, EA S, Deng C, JA P. 2013. Differentially expressed microRNAs and affected biological pathways revealed by modulated modularity clustering (MMC) analysis of human preeclamptic and IUGR placentas. Placenta. 34(7):599–605. doi:10.1016/j.placenta.2013.04.007.
- Guo Y, Chen Y, Zhang Y, Zhang Y, Chen L, Mo D. 2012. Up-regulated miR-145 expression inhibits porcine preadipocytes differentiation by targeting IRS1. Int J Biol Sci. 8(10):1408–1417. doi:10.7150/ijbs.4597.
- Hayder H, O’Brien J, Nadeem U, Peng C. 2018. MicroRNAs: crucial regulators of placental development. Reproduction. 155(6):R259–R271. doi:10.1530/REP-17-0603.
- Higashijima A, Miura K, Mishima H, Kinoshita A, Jo O, Abe S, Hasegawa Y, Miura S, Yamasaki K, Yoshida A, et al.. 2013. Characterization of placenta-specific microRNAs in fetal growth restriction pregnancy. Prenat Diagn. 33(3):214–222. doi:10.1002/pd.4045.
- Holdsworth-Carson SJ, Lim R, Mitton A, Whitehead C, GE R, Permezel M, Lappas M. 2010. Peroxisome proliferator-activated receptors are altered in pathologies of the human placenta: gestational diabetes mellitus, intrauterine growth restriction and preeclampsia. Placenta. 31(3):222–229. doi:10.1016/j.placenta.2009.12.009
- Hromadnikova I, Kotlabova K, Hympanova L, Krofta L. 2015a. Cardiovascular and Cerebrovascular Disease Associated microRNAs Are Dysregulated in Placental Tissues Affected with Gestational Hypertension, Preeclampsia and Intrauterine Growth Restriction. PLoS One. 10(9):e0138383.
- Hromadnikova I, Kotlabova K, Ondrackova M, Pirkova P, Kestlerova A, Novotna V, Hympanova L, Krofta L. 2015b. Expression profile of C19MC microRNAs in placental tissue in pregnancy-related complications. DNA Cell Biol. 34(6):437–457. doi:10.1089/dna.2014.2687.
- Hu W, Gao F, Zhang H, Hiromori Y, Arakawa S, Nagase H, Nakanishi T, Hu J. 2017. Activation of Peroxisome Proliferator-Activated Receptor Gamma and Disruption of Progesterone Synthesis of 2-Ethylhexyl Diphenyl Phosphate in Human Placental Choriocarcinoma Cells: comparison with Triphenyl Phosphate. Environ Sci Technol. 51(7):4061–4068. doi:10.1021/acs.est.7b00872.
- Hu W, Wang X, Ding X, Li Y, Zhang X, Xie P, Yang J, Wang S. 2012. MicroRNA-141 represses HBV replication by targeting PPARA. PLoS One. 7(3):e34165.
- Hu XQ, Zhang L. 2019. MicroRNAs in Uteroplacental Vascular Dysfunction. Cells. 8(11):1344. doi:10.3390/cells8111344.
- Huang C, Gou S, Wang L, Huang K, Liu L, Zhao W, Zheng L, Xiao J. 2016a. MicroRNAs and Peroxisome Proliferator-Activated Receptors Governing the Differentiation of Mesenchymal Stem Cells. Curr Stem Cell Res Ther. 11(3):197–207. doi:10.2174/1574888X10666150528144517.
- Huang C, XJ L, QunZhou XJ, TT M, XM M, Li J, Li J. 2016b. MiR-146a modulates macrophage polarization by inhibiting Notch1 pathway in RAW264.7 macrophages. Int Immuno Pharmacol. 32:46–54. doi:10.1016/j.intimp.2016.01.009.
- Huang L, Shen Z, Xu Q, Huang X, Chen Q, Li D. 2013. Increased levels of microRNA-424 are associated with the pathogenesis of fetal growth restriction. Placenta. 34(7):624–627. doi:10.1016/j.placenta.2013.04.009.
- Hutvagner G, Mclachlan J, AE P, Balint E, Tuschl T, PD Z. 2001. A cellular function for the RNA-interference enzyme Dicer in the maturation of the let-7 small temporal RNA. Science. 293:834–838.
- Iliopoulos D, KN M, Oikonomou P, Tsezou A, Koutsopoulos S. 2008. Integrative microRNA and proteomic approaches identify novel osteoarthritis genes and their collaborative metabolic and inflammatory networks. PLoS One. 3(11):e3740. doi:10.1371/journal.pone.0003740.
- Issler O, Chen A. 2015. Determining the role of microRNAs in psychiatric disorders. Nat Rev Neurosci. 16(4):201–212. doi:10.1038/nrn3879.
- Jacometo CB, Alharthi AS, Zhou Z, Luchini D, Loor JJ. 2018. Maternal supply of methionine during late pregnancy is associated with changes in immune function and abundance of microRNA and mRNA in Holstein calf polymorphonuclear leukocytes. J Dairy Sci. 101(9):8146–8158
- Jang SY, Chae MK, Lee JH, Lee EJ, Yoon JS. 2019. MicroRNA-27 inhibits adipogenic differentiation in orbital fibroblasts from patients with Graves' orbitopathy. PLoS One. 14(8):e0221077
- Jawerbaum A, Capobianco E. 2011. Review: effects of PPAR activation in the placenta and the fetus: implications in maternal diabetes. Placenta. 32(Suppl. 2):S212–S217. doi:10.1016/j.placenta.2010.12.002.
- Jennewein C, von Knethen A, Schmid T, Brüne B. 2010. MicroRNA-27b contributes to lipopolysaccharide-mediated peroxisome proliferator-activated receptor gamma (PPARgamma) mRNA destabilization. J Biol Chem. 285(16):11846–11853. doi:10.1074/jbc.M109.066399.
- Jeong BC, Kang IH, Koh JT. 2014. MicroRNA-302a inhibits adipogenesis by suppressing peroxisome proliferator-activated receptor γ expression. FEBS Lett. 588(18):3427–3434
- Jump DB. 2002. Dietary polyunsaturated fatty acids and regulation of gene transcription. Curr Opin Lipidol. 13(2):155–164. doi:10.1097/00041433-200204000-00007.
- Jump DB. 2008. N-3 polyunsaturated fatty acid regulation of hepatic gene transcription. Curr Opin Lipidol. 19(3):242–247. doi:10.1097/MOL.0b013e3282ffaf6a.
- Junaid TO, Brownbill P, Chalmers N, Johnstone ED, Aplin JD. 2014. Fetoplacental vascular alterations associated with fetal growth restriction. Placenta. 35(10):808–15
- Kadam L, Kilburn B, Baczyk D, HR K-G, Kingdom J, Drewlo S. 2019. Rosiglitazone blocks first trimester in-vitro placental injury caused by NF-κB-mediated inflammation. Sci Rep. 9(1):2018. doi:10.1038/s41598-018-38336-2.
- Karbiener M, Fischer C, Nowitsch S, Opriessnig P, Papak C, Ailhaud G, Dani C, EZ A, Scheideler M. 2009. microRNA miR-27b impairs human adipocyte differentiation and targets PPARgamma. Biochem Biophys Res Commun. 390(2):247–251. doi:10.1016/j.bbrc.2009.09.098.
- Khvorova A, Reynolds A, SD J. 2003. Functional siRNAs and miRNAs exhibit strand bias. Cell. 115(2):209–216. doi:10.1016/S0092-8674(03)00801-8.
- Kida K, Nakajima M, Mohri T, Oda Y, Takagi S, Fukami T, Yokoi T. 2011. PPARα is regulated by miR-21 and miR-27b in human liver. Pharm Res. 28(10):2467–2476. doi:10.1007/s11095-011-0473-y.
- Kim SY, Kim AY, Lee HW, Son YH, Lee GY, Lee J-W., Lee YS, Kim JB. 2010. miR-27a is a negative regulator of adipocyte differentiation via suppressing PPARγ expression. Biochemical and Biophysical Research Communications. 392(3):323–328 doi:10.1016/j.bbrc.2010.01.012
- Kim YK, Kim B, Kim VN. 2016. Re-evaluation of the roles of DROSHA, Exportin 5, and DICER in microRNA biogenesis. Proc Natl Acad Sci U S A. 113(13):E1881–E1889. doi:10.1073/pnas.1602532113.
- Kim YM, Bujold E, Chaiworapongsa T, Gomez R, Yoon BH, Thaler HT, Rotmensch S, Romero R. 2003. Failure of physiologic transformation of the spiral arteries in patients with preterm labor and intact membranes. American Journal of Obstetrics and Gynecology. 189(4):1063–1069 doi:10.1067/S0002-9378(03)00838-X
- Kitsiou-Tzeli S, Tzetis M. 2017. Maternal epigenetics and fetal and neonatal growth. Curr Opin Endocrinol Diabetes Obes. 24:43–46.
- Köpke S, Buhrke T, Lampen A. 2015. miRNA expression in human intestinal Caco-2 cells is comparably regulated by cis- and trans-fatty acids. Lipids. 50(3):227–239. doi:10.1007/s11745-015-3988-x.
- Koukoura O, Sifakis S, DA S. 2012. DNA methylation in the human placenta and fetal growth (review). Mol Med Rep. 5(4):883–889. doi:10.3892/mmr.2012.763.
- Krishna U, Bhalerao S. 2011. Placental insufficiency and fetal growth restriction. J Obstet Gynaecol India. 61(5):505–511. doi:10.1007/s13224-011-0092-x.
- Kubota N, Terauchi Y, Miki H, Tamemoto H, Yamauchi T, Komeda K, Satoh S, Nakano R, Ishii C, Sugiyama T, et al.. 1999. PPAR gamma mediates high-fat diet-induced adipocyte hypertrophy and insulin resistance. Mol Cell. 4(4):597–609. doi:10.1016/S1097-2765(00)80210-5.
- Kulshreshtha R, Ferracin M, SE W, Garzon R, Alder H, FJ A-P, Davuluri R, CG L, CM C, Negrini M, et al.. 2007. A microRNA signature of hypoxia. Mol Cell Biol. 27(5):1859–1867. doi:10.1128/MCB.01395-06.
- Kumar S, Rani R, Karns R, CR G. 2019. Augmenter of liver regeneration protein deficiency promotes hepatic steatosis by inducing oxidative stress and microRNA-540 expression. Faseb J. 33(3):3825–3840. doi:10.1096/fj.201802015R.
- Kwan STC, King JH, Grenier JK, Yan J, Jiang X, Roberson MS, Caudill MA. 2018. Maternal Choline Supplementation during Normal Murine Pregnancy Alters the Placental Epigenome: Results of an Exploratory Study. Nutrients. 10(4):E417
- LA D, Wang N, MS S, JR L, Ivanov I, RS C. 2009. n-3 Polyunsaturated fatty acids modulate carcinogen-directed non-coding microRNA signatures in rat colon. Carcinogenesis. 30(12):2077–2084. doi:10.1093/carcin/bgp245.
- Lane SL, Dodson RB, Doyle AS, Park H, Rathi H, Matarrazo CJ, Moore LG, Lorca RA, Wolfson GH, Julian CG. 2019. Pharmacological activation of peroxisome proliferator‐activated receptor γ (PPAR‐γ) protects against hypoxia‐associated fetal growth restriction. FASEB J. 33(8):8999–9007 doi:10.1096/fsb2.v33.8
- Larqué E, Gil-Sánchez A, MT P-S, Koletzko B. 2012. Omega 3 fatty acids, gestation and pregnancy outcomes. Br J Nutr. 107(S2):S77–S84. doi:10.1017/S0007114512001481.
- Lee EK, Lee MJ, Abdelmohsen K, Kim W, Kim MM, Srikantan S, Martindale JL, Hutchison ER, Kim HH, Marasa BS, et al. 2011. miR-130 suppresses adipogenesis by inhibiting peroxisome proliferator-activated receptor gamma expression. Mol Cell Biol. 31(4):626–638
- Lee J-J., Drakaki A, Iliopoulos D, Struhl K. 2012. MiR-27b targets PPARγ to inhibit growth, tumor progression and the inflammatory response in neuroblastoma cells. Oncogene. 31(33):3818–3825. doi:10.1038/onc.2011.543
- Lee MY, Lee YJ, Kim YH, Lee SH, Park JH, Kim MO, Suh HN, Ryu JM, Yun SP, Jang MW, et al. 2009. Role of Peroxisome Proliferator-Activated Receptor (PPAR)δ in Embryonic Stem Cell Proliferation. Int J Stem Cells. 2(1):28–34
- Lee Y, Jeon K, JT L, Kim S, VN K. 2002. MicroRNA maturation: stepwise processing and subcellular localization. Embo J. 21(17):4663–4670. doi:10.1093/emboj/cdf476.
- Lee Y, Kim M, Han J, KH Y, Lee S, HeeBaek S, Kim VN. 2004. MicroRNA genes are transcribed by RNA polymerase II. Embo J. 23(20):4051–4060. doi:10.1038/sj.emboj.7600385.
- Lendvai A, MJ D, Plosch T, Ensenauer R. 2016. The peroxisome proliferator-activated receptors under epigenetic control in placental metabolism and fetal development. Am J Physiol Endocrinol Metab. 310(10):E797–10. doi:10.1152/ajpendo.00372.2015.
- Li L, Huang X, He Z, Xiong Y, Fang Q. 2019a. miRNA-210-3p regulates trophoblast proliferation and invasiveness through fibroblast growth factor 1 in selective intrauterine growth restriction. J Cell Mol Med. 23(6):4422–4433. doi:10.1111/jcmm.14335.
- Li P, Wang X, Shan Q, Wu Y, Wang Z. 2017. MicroRNA-130b promotes cell migration and invasion by inhibiting peroxisome proliferator-activated receptor-γ in human glioma. Oncol Lett. 13(4):2615–2622. doi:10.3892/ol.2017.5760.
- Li W, Li Z, Zhou D, Zhang X, Yan J, Huang G. 2019b. Maternal folic acid deficiency stimulates neural cell apoptosis via miR-34a associated with Bcl-2 in the rat foetal brain. Int J Dev Neurosci. 72(1):6–12. doi:10.1016/j.ijdevneu.2018.11.002.
- Li X, Chen Y, Wu S, He J, Lou L, Ye W, Wang J. 2015. microRNA-34a and microRNA-34c promote the activation of human hepatic stellate cells by targeting peroxisome proliferator-activated receptor γ. Mol Med Rep. 11(2):1017–1024. doi:10.3892/mmr.2014.2846.
- Li Y. 2018. Epigenetic Mechanisms Link Maternal Diets and Gut Microbiome to Obesity in the Offspring. Front Genet. 9:342. doi:10.3389/fgene.2018.00342.
- Li Z-J., Ou-Yang P-H., Han X-P.. 2014. Profibrotic effect of miR-33a with Akt activation in hepatic stellate cells. Cellular Signalling. 26(1):141–148 doi:10.1016/j.cellsig.2013.09.018
- Lin L, Lin H, Wang L, Wang B, Hao X, Shi Y. 2015. miR-130a regulates macrophage polarization and is associated with non-small cell lung cancer. Oncol Rep. 34(6):3088–3096. doi:10.3892/or.2015.4301.
- Lin Q, Gao Z, RM A, Ye J, Yun Z. 2009. A role of miR-27 in the regulation of adipogenesis. Febs J. 276(8):2348–2358. doi:10.1111/j.1742-4658.2009.06967.x.
- Liu L, Zhuang X, Jiang M, Guan F, Fu Q, Lin J. 2017. ANGPTL4 mediates the protective role of PPARγ activators in the pathogenesis of preeclampsia. Cell Death Dis. 8(9):e3054. doi:10.1038/cddis.2017.419.
- Liu Y, Zhang Y, Liu P, Bai H, Li X, Xiao J, Yuan Q, Geng S, Yin H, Zhang H, et al.. 2019. MicroRNA-128 knockout inhibits the development of Alzheimer’s disease by targeting PPARγ in mouse models. Eur J Pharmacol. 843:134–144. doi:10.1016/j.ejphar.2018.11.004.
- Luo H, Zhu H, Zhou B, Xiao X, Zuo X. 2015. MicroRNA-130b regulates scleroderma fibrosis by targeting peroxisome proliferator-activated receptor γ. Mod Rheumatol. 25(4):595–602. doi:10.3109/14397595.2014.1001311.
- Maccani MA, Avissar-Whiting M, Banister CE, McGonnigal B, Padbury JF, Marsit CJ. 2010. Maternal cigarette smoking during pregnancy is associated with downregulation of miR-16 , miR-21 , and miR-146a in the placenta . Epigenetics. 5(7):583–589 doi:10.4161/epi.5.7.12762
- Maccani MA, JF P, CJ M. 2011. miR-16 and miR-21 expression in the placenta is associated with fetal growth. PLoS One. 6(6):e21210. doi:10.1371/journal.pone.0021210.
- Martinelli R, Nardelli C, Pilone V, Buonomo T, Liguori R, Castanò I, Buono P, Masone S, Persico G, Forestieri P, et al.. 2010. miR-519d overexpression is associated with human obesity. Obesity (Silver Spring). 18(11):2170–2176. doi:10.1038/oby.2009.474.
- Östling H, Kruse R, Helenius G, Lodefalk M. 2019. Placental expression of microRNAs in infants born small for gestational age. Placenta. 81:46–53. doi:10.1016/j.placenta.2019.05.001.
- Martínez N, Capobianco E, White V, Pustovrh MC, Higa R, Jawerbaum A. 2008. Peroxisome proliferator-activated receptor alpha activation regulates lipid metabolism in the feto-placental unit from diabetic rats. Reproduction. 136(1):95–103. doi:10.1530/REP-08-0028.
- Martínez N, Kurtz M, Capobianco E, Higa R, White V, Jawerbaum A. 2011. PPARα agonists regulate lipid metabolism and nitric oxide production and prevent placental overgrowth in term placentas from diabetic rats. J Mol Endocrinol. 47(1):1–12. doi:10.1530/JME-10-0173.
- Martinez N, Sosa M, Higa R, Fornes D, Capobianco E, Jawerbaum A. 2012. Dietary treatments enriched in olive and safflower oils regulate seric and placental matrix metalloproteinases in maternal diabetes. Placenta. 33(1):8–16. doi:10.1016/j.placenta.2011.10.015.
- McGregor RA, Choi MS. 2011. microRNAs in the regulation of adipogenesis and obesity. Curr Mol Med. 11(4):304–316
- Meher A, Randhir K, Mehendale S, Wagh G, Joshi S, Annunziato L. 2016a. Maternal Fatty Acids and Their Association with Birth Outcome: A Prospective Study. PLoS One. 11(1):e0147359. doi:10.1371/journal.pone.0147359.
- Meher A, Sundrani D, Joshi S. 2015. Maternal nutrition influences angiogenesis in the placenta through peroxisome proliferator activated receptors: A novel hypothesis. Mol Reprod Dev. 82(10):726–734. doi:10.1002/mrd.22518.
- Meher AP, Wadhwani N, Randhir K, Mehendale S, Wagh G, SR J. 2016b. Placental DHA and mRNA levels of PPARγ and LXRα and their relationship to birth weight. J Clin Lipidol. 10(4):767–774. doi:10.1016/j.jacl.2016.02.004.
- Middleton P, JC G, JF G, Shepherd E, SF O, Makrides M. 2018. Omega-3 fatty acid addition during pregnancy. Cochrane Database Syst Rev. 11:CD003402.
- Morales Prieto DM, Markert UR. 2011. MicroRNAs in pregnancy. Journal of Reproductive Immunology. 88(2):106–111 doi:10.1016/j.jri.2011.01.004
- Morales-Prieto P, Chaiwangyen W, Ospina-Prieto S, Schneider U, Herrmann J, Gruhn B, UR M. 2012. MicroRNA expression profiles of trophoblastic cells. Placenta. 33(9):725‐734.
- Motohashi N, MS A, JC C, LM K. 2012. Identification of a novel microRNA that regulates the proliferation and differentiation in muscle side population cells. Stem Cells Dev. 21(16):3031–3043. doi:10.1089/scd.2011.0721.
- Mouillet JF, Chu T, Sadovsky Y. 2011a. Expression patterns of placental microRNAs. Birth Defects Res A Clin Mol Teratol. 91(8):737–743. doi:10.1002/bdra.20782.
- Mouillet JF, Ouyang Y, CB C, Sadovsky Y. 2015. MicroRNAs in placental health and disease. Am J Obstet Gynecol. 213(4 Suppl):S163‐S172.
- Nadra K, Quignodon L, Sardella C, Joye E, Mucciolo A, Chrast R, Desvergne B. 2010. PPARgamma in placental angiogenesis. Endocrinology. 151(10):4969–4981. doi:10.1210/en.2010-0131.
- Nana-Sinkam SP, Croce CM. 2014. MicroRNA regulation of tumorigenesis, cancer progression and interpatient heterogeneity: towards clinical use. Genome Biol. 15(9):445 doi:10.1186/s13059-014-0445-8
- Nishimura K, Nakano N, VS C, Kaneto M, Torii M, MA H, Yamauchi N, Kawai M. 2013. Effect of PPARβ/δ agonist on the placentation and embryo-fetal development in rats. Birth Defects Res B Dev Reprod Toxicol. 98(2):164–169. doi:10.1002/bdrb.21052.
- Ouyang Y, JF M, CB C, Sadovsky Y. 2014. Review: placenta-specific microRNAs in exosomes - good things come in nano-packages. Placenta. 35(Suppl):S69–73. doi:10.1016/j.placenta.2013.11.002.
- Pan S, Zheng Y, Zhao R, Yang X. 2013. MicroRNA-130b and microRNA-374b mediate the effect of maternal dietary protein on offspring lipid metabolism in Meishan pigs. Br J Nutr. 109(10):1731–1738. doi:10.1017/S0007114512003728.
- Parast MM, Yu H, Ciric A, MW S, Davis V, DS M, Dey SK. 2009. PPARgamma regulates trophoblast proliferation and promotes labyrinthine trilineage differentiation. PLoS One. 4(11):e8055. doi:10.1371/journal.pone.0008055.
- Park S, Lim W, FW B, KY W, Song G. 2019. Quercetin inhibits proliferation of endometriosis regulating cyclin D1 and its target microRNAs in vitro and in vivo. J Nutr Biochem. 63:87–100. doi:10.1016/j.jnutbio.2018.09.024.
- Payton A, Clark J, Eaves L, Santos JHP, Smeester L, JT B, TM O, RC F, JE R. 2020. Placental genomic and epigenomic signatures associated with infant birth weight highlight mechanisms involved in collagen and growth factor signaling. Reprod Toxicol. 96:221–230. doi:10.1016/j.reprotox.2020.07.007.
- Perron MP, Provost P. 2009. Protein components of the microRNA pathway and human diseases. Methods Mol Biol. 487:369–385.
- Portius D, Sobolewski C, Foti M. 2017. MicroRNAs-Dependent Regulation of PPARs in Metabolic Diseases and Cancers. PPAR Res. 2017:7058424. doi:10.1155/2017/7058424.
- Quintanilha BJ, Reis BZ, Duarte GBS, Cozzolino SMF, Rogero MM. 2017. Nutrimiromics: Role of microRNAs and Nutrition in Modulating Inflammation and Chronic Diseases. Nutrients. 9(11):E1168
- Roberti SL, Higa R, White V, TL P, Jansson T, Jawerbaum A. 2018. Critical role of mTOR, PPARγ and PPARδ signaling in regulating early pregnancy decidual function, embryo viability and feto-placental growth. Mol Hum Reprod. 24(6):327–340. doi:10.1093/molehr/gay013.
- Rodie VA, Young A, Jordan F, Sattar N, Greer I A., Freeman D J.. 2005. Human placental peroxisome proliferator-activated receptor delta and gamma expression in healthy pregnancy and in preeclampsia and intrauterine growth restriction. J Soc Gynecol Investig. 12(5):320–329. doi:10.1016/j.jsgi.2005.03.004
- Rodosthenous RS, HH B, AP S, AC J, AE D, Svensson K, Solano M, Tellez-Rojo M, Wright R, Baccarelli A. 2017. Second trimester extracellular microRNAs in maternal blood and fetal growth: an exploratory study. Epigenetics. 12(9):804–810. doi:10.1080/15592294.2017.1358345.
- Roland MC, CM F, Voldner N, Godang K, Bollerslev J, Haugen G, Henriksen T, Harvey N. 2012. Fetal growth versus birthweight: the role of placenta versus other determinants. PLoS One. 7(6):e39324. doi:10.1371/journal.pone.0039324.
- Ross SA, Davis CD. 2011. MicroRNA, nutrition, and cancer prevention. Adv Nutr. 2(6):472–485. doi:10.3945/an.111.001206.
- Sadovsky Y, Mouillet J-F, Ouyang Y, Bayer A, Coyne CB. 2015. The Function of TrophomiRs and Other MicroRNAs in the Human Placenta. Cold Spring Harb Perspect Med. 5(8):a023036. doi:10.1101/cshperspect.a023036.
- Salehi R, DJ A. 2017. Prepartum maternal diets supplemented with oilseeds alter the fatty acid profile in bovine neonatal plasma possibly through reduced placental expression of fatty acid transporter protein 4 and fatty acid translocase. Reprod Fertil Dev. 29(9):1846–1855. doi:10.1071/RD15476.
- Sarkar J, Gou D, Turaka P, Viktorova E, Ramchandran R, JU R. 2010. MicroRNA-21 plays a role in hypoxia-mediated pulmonary artery smooth muscle cell proliferation and migration. Am J Physiol Lung Cell Mol Physiol. 299(6):L861–L871. doi:10.1152/ajplung.00201.2010.
- Sarr O, JA T, Zhao L, TY L, TR R, Randeva HS. 2014. Low birth weight male guinea pig offspring display increased visceral adiposity in early adulthood. PLoS One. 9(6):e98433. doi:10.1371/journal.pone.0098433.
- Schaiff WT, Bildirici I, Cheong M, Chern PL, Nelson DM, Sadovsky Y. 2005. Peroxisome Proliferator-Activated Receptor-γ and Retinoid X Receptor Signaling Regulate Fatty Acid Uptake by Primary Human Placental Trophoblasts. J Clin Endocrinol Metab. 90(7):4267–4275. doi:10.1210/jc.2004-2265.
- Schaiff WT, Carlson MG, Smith SD, Levy R, Nelson DM, Sadovsky Y. 2000. Peroxisome Proliferator-Activated Receptor- Modulates Differentiation of Human Trophoblast in a Ligand-Specific Manner. Journal of Clinical Endocrinology & Metabolism. 85(10):3874–3881 doi:10.1210/jc.85.10.3874
- Seenprachawong K, Tawornsawutruk T, Nantasenamat C, Nuchnoi P, Hongeng S, Supokawej A. 2018. miR-130a and miR-27b Enhance Osteogenesis in Human Bone Marrow Mesenchymal Stem Cells via Specific Down-Regulation of Peroxisome Proliferator-Activated Receptor γ. Front Genet. 9:543. doi:10.3389/fgene.2018.00543.
- Shah T, Mishra S, More A, Otiv S, Apte K, Joshi K. 2017. Combination of vitamin B12 active forms improved fetal growth in Wistar rats through up-regulation of placental miR-16 and miR-21 levels. Life Sci. 191:97–103. doi:10.1016/j.lfs.2017.10.017.
- Shalom-Barak T, Zhang X, Chu T, Timothy SW, Reddy JK, Xu J, Sadovsky Y, Barak Y. 2012. Placental PPARγ regulates spatiotemporally diverse genes and a unique metabolic network. Dev Biol. 372(1):143–155. doi:10.1016/j.ydbio.2012.08.021.
- Shoaito H, Petit J, Chissey A, Auzeil N, Guibourdenche J, Gil S, Laprévote O, Fournier T, Degrelle SA. 2019. The Role of Peroxisome Proliferator–Activated Receptor Gamma (PPARγ) in Mono(2-ethylhexyl) Phthalate (MEHP)-Mediated Cytotrophoblast Differentiation. Environ Health Perspect. 127(2):27003. doi:10.1289/EHP3730.
- Song GY, Song WW, Han Y, Wang D, Na Q. 2013. Characterization of the role of microRNA-517a expression in low birth weight infants. Journal of Developmental Origins of Health and Disease. 4(6):522–526. doi:10.1017/S204017441300024X.
- Sontheimer EJ. 2005. Assembly and function of RNA silencing complexes. Nat Rev Mol Cell Biol. 6(2):127–138. doi:10.1038/nrm1568.
- Sun J, Wang Y1, Li Y, Zhao G. 2014. Downregulation of PPARγ by miR-548d-5p suppresses the adipogenic differentiation of human bone marrow mesenchymal stem cells and enhances their osteogenic potential. J Transl Med. 12(1):168. doi:10.1186/1479-5876-12-168.
- Sun L, Trajkovski M. 2014. MiR-27 orchestrates the transcriptional regulation of brown adipogenesis. Metabolism. 63(2):272–282. doi:10.1016/j.metabol.2013.10.004.
- Sundrani DP, Reddy US, Chavan-Gautam PM, Mehendale SS, Chandak GR, Joshi SR. 2014. Altered methylation and expression patterns of genes regulating placental angiogenesis in preterm pregnancy. Reprod Sci. 21(12):1508–1517
- Tang Q, Wu W, Xu X, Huang L, Gao Q, Chen H, Sun H, Xia Y, Sha J, Wang X, et al.. 2013. miR-141 contributes to fetal growth restriction by regulating PLAG1 expression. PLoS One. 8(3):e58737. doi:10.1371/journal.pone.0058737.
- Taniguchi M1, Nakajima I, Chikuni K, Kojima M, Awata T, Mikawa S. 2014. MicroRNA-33b downregulates the differentiation and development of porcine preadipocytes. Mol Biol Rep. 5(8):1081–1090. doi:10.1007/s11033-013-2954-z.
- Tao L, Wu L, Zhang W, Ma W-T, Yang G-Y, Zhang J, Xue D-Y, Chen B, Liu C. 2020. Peroxisome proliferator-activated receptor γ inhibits hepatic stellate cell activation regulated by miR-942 in chronic hepatitis B liver fibrosis. Life Sci. 253:117572. doi:10.1016/j.lfs.2020.117572.
- Tarrade A, Schoonjans K, Guibourdenche J, Bidart JM, Vidaud M, Auwerx J, Rochette-Egly C, Evain-Brion D. 2001. PPARγ/RXRα Heterodimers Are Involved in Human CGβ Synthesis and Human Trophoblast Differentiation. Endocrinology. 142(10):4504–4514. doi:10.1210/endo.142.10.8448.
- Thamotharan S, Chu A, Kempf K, Janzen C, Grogan T, Elashoff DA, Devaskar SU, Baud O. 2017. Differential microRNA expression in human placentas of term intra-uterine growth restriction that regulates target genes mediating angiogenesis and amino acid transport. PLoS One. 12(5):e0176493. doi:10.1371/journal.pone.0176493.
- Thulin P, Wei T, Werngren O, Cheung L, RM F, Grandér D, Corcoran M, Ehrenborg E. 2013. MicroRNA-9 regulates the expression of peroxisome proliferator-activated receptor δ in human monocytes during the inflammatory response. Int J Mol Med. 31(5):1003–1010.
- Tong JL, Zhang CP, Nie F, Xu XT, Zhu MM, Xiao SD, Ran ZH. 2011. MicroRNA 506 regulates expression of PPAR alpha in hydroxycamptothecin-resistant human colon cancer cells. FEBS Lett. 585(22):3560–3568. doi:10.1016/j.febslet.2011.10.021.
- Tryggestad JB, Teague AM, Sparling DP, Jiang S, Chernausek SD. 2019. Macrophage-Derived microRNA-155 Increases in Obesity and Influences Adipocyte Metabolism by Targeting Peroxisome Proliferator-Activated Receptor Gamma. Obesity (Silver Spring). 27(11):1856–1864
- Tsochandaridis M, Nasca L, Toga C, Levy-Mozziconacci A. 2015. Circulating microRNAs as clinical biomarkers in the predictions of pregnancy complications. Biomed Res Int. 2015:294954. doi:10.1155/2015/294954.
- Tu K, Zheng X, Dou C, Li C, Yang W, Yao Y, Liu Q. 2014. MicroRNA-130b promotes cell aggressiveness by inhibiting peroxisome proliferator-activated receptor gamma in human hepatocellular carcinoma. Int J Mol Sci. 15(11):20486–20499. doi:10.3390/ijms151120486.
- Valero De Bernabé J, Soriano T, Albaladejo R, Juarranz M, Calle ME, Martínez D, Domínguez-Rojas V. 2004. Risk factors for low birth weight: a review. Eur J Obstet Gynecol Reprod Biol. 116(1):3–15. doi:10.1016/j.ejogrb.2004.03.007.
- Wang D, Na Q, Song -W-W, Song G-Y. 2014. Altered Expression of miR-518b and miR-519a in the placenta is associated with low fetal birth weight. Am J Perinatol. 31(9):729–734. doi:10.1055/s-0033-1361832.
- Wang H, Xie H, Sun X, Tranguch S, Zhang H, Jia X, Wang D, Das SK, Desvergne B, Wahli W, et al.. 2007. Stage-specific Integration of Maternal and Embryonic Peroxisome Proliferator-activated Receptor δ Signaling Is Critical to Pregnancy Success. J Biol Chem. 282(52):37770–37782. doi:10.1074/jbc.M706577200.
- Wang J, Song Y, Zhang Y, Xiao H, Sun Q, Hou N, Guo S, Wang Y, Fan K, Zhan D, et al.. 2012. Cardiomyocyte overexpression of miR-27b induces cardiac hypertrophy and dysfunction in mice. Cell Res. 22(3):516–527.
- Wang Wei-Lin Winnie., Welsh J, Tenniswood M. 2013. 1,25-Dihydroxyvitamin D3 modulates lipid metabolism in prostate cancer cells through miRNA mediated regulation of PPARA. The Journal of Steroid Biochemistry and Molecular Biology. 136:247–251 doi:10.1016/j.jsbmb.2012.09.033
- WHO. 2014. Global nutrition targets 2025: low birth weight policy brief Geneva. World Health Organization.
- Xie L, JF M, Chu T, WT P, Sadovsky E, Knöfler M, Sadovsky Y. 2014. C19MC microRNAs regulate the migration of human trophoblasts. Endocrinology. 155(12):4975‐4985.
- Xu B, Shen J, Li D, Ning B, Guo L, Bing H, Chen J, Li Y. 2020. Overexpression of microRNA-9 inhibits 3T3-L1 cell adipogenesis by targeting PNPLA3 via activation of AMPK. Gene. 730:144260.
- Xu G, Shi C, Ji C, Song G, Chen L, Yang L, Zhao Y, Guo X. 2014. Expression of microRNA-26b, an obesity-related microRNA, is regulated by free fatty acids, glucose, dexamethasone and growth hormone in human adipocytes. Mol Med Rep. 10(1):223–228.
- Xu Y, Romero R, Miller D, Kadam L, TN M, Plazyo O, Garcia-Flores V, SS H, Xu Z, AL T, et al.. 2016. An M1-like macrophage polarization in decidual tissue during spontaneous preterm labor that is attenuated by rosiglitazone treatment. J Immunol. 196(6):2476–2491.
- Xue M, Wang K, Wang A, Li R, Wang Y, Sun S, Yan D, Song G, Xu H, Sun G, et al.. 2019. MicroRNA Sequencing Reveals the Effect of Different Levels of Non-Fibrous Carbohydrate/Neutral Detergent Fiber on Rumen Development in Calves. Animals (Basel). 9(8):496. doi: 10.3390/ani9080496.
- Yang W-M., Jeong H-J., Park S-Y., Lee W. 2014. Induction of miR-29a by saturated fatty acids impairs insulin signaling and glucose uptake through translational repression of IRS-1 in myocytes. FEBS Letters. 588(13):2170–2176 doi:10.1016/j.febslet.2014.05.011
- Yang Z, Bian C, Zhou H, Huang S, Wang S, Liao L, RC Z. 2011. MicroRNA hsa-miR-138 inhibits adipogenic differentiation of human adipose tissue-derived mesenchymal stem cells through adenovirus EID-1. Stem Cells Dev. 20(2):259–267.
- Yao F, Yu Y, Feng L, Li J, Zhang M, Lan X, Yan X, Liu Y, Guan F, Zhang M, et al.. 2017. Adipogenic miR-27a in adipose tissue upregulates macrophage activation via inhibiting PPARγ of insulin resistance induced by high-fat diet-associated obesity. Exp Cell Res. 355(2):105–112.
- Zhang J, Peng X, Yuan A, Xie Y, Yang Q, Xue L. 2017. Peroxisome proliferator activated receptor γ mediates porcine placental angiogenesis through hypoxia inducible factor, vascular endothelial growth factor and angiopoietin mediated signaling. Mol Med Rep. 16(3):2636–2644.
- Zhang JF, Fu WM, He ML, Xie WD, Lv Q, Wan G, Li G, Wang H, Lu G, Hu X, et al. 2011. MiRNA-20a promotes osteogenic differentiation of human mesenchymal stem cells by co-regulating BMP signaling. RNA Biol. 8(5):829–838
- Zhang M, Zhou Z, Wang J, Li S. 2016. MiR-130b promotes obesity associated adipose tissue inflammation and insulin resistance in diabetes mice through alleviating M2 macrophage polarization via repression of PPAR-γ. Immunol Lett. 180:1–8.
- Zhang R, YQ W, Su B. 2008. Molecular evolution of a primate-specific microRNA family. Mol Biol Evol. 25(7):1493‐1502.
- Zhang Y, Huang X, Zhou J, Yin Y, Zhang T, Chen D. 2018. PPARγ provides anti-inflammatory and protective effects in intrahepatic cholestasis of pregnancy through NF-κB pathway. Biochem Biophys Res Commun. 504(4):834–842.
- Zhao C, Zhang T, Shi Z, Ding H, Ling X. 2014. MicroRNA-518d regulates PPARα protein expression in the placentas of females with gestational diabetes mellitus. Mol Med Rep. 9(6):2085–2090.
- Zheng J, Xiao X, Zhang Q, Wang T, Yu M, Xu J. 2017. Maternal Low-Protein Diet Modulates Glucose Metabolism and Hepatic MicroRNAs Expression in the Early Life of Offspring. Nutrients. 9(3):E205.
- Zheng L, GC L, Sheng J, YD Y. 2010. Effect of miRNA-10b in regulating cellular steatosis level by targeting PPAR-alpha expression, a novel mechanism for the pathogenesis of NAFLD.J. Gastroenterol Hepatol. 25(1):156–163.
- Zhou J, KC W, Wu W, Subramaniam S, Shyy JY, Chiu JJ, Li JY, Chien S. 2011. MicroRNA-21 targets peroxisome proliferators-activated receptor-alpha in an autoregulatory loop to modulate flow-induced endothelial inflammation. Proc Natl Acad Sci U S A. 108(25):10355–10360.
- Zhou Y, Gu P, Shi W, Li J, Hao Q, Cao X, Lu Q, Zeng Y. 2016. MicroRNA-29a induces insulin resistance by targeting PPARδ in skeletal muscle cells. Int J Mol Med. 37(4):931–938.
- Zou Z, He Z, Cai J, Huang L, Zhu H, Luo Y. 2019. Potential role of microRNA-424 in regulating ERRγ to suppress trophoblast proliferation and invasion in fetal growth restriction. Placenta. 83:57–62.