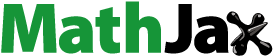
Abstract
We examined the effects of DNA methyltransferase inhibitor – RG108, and histone deacetylase inhibitor – SAHA, on the reprogramming parameters of cloned mouse embryos produced by somatic cell nuclear transfer into oocytes. The programming parameters studied included dynamics of histone reacetylation, developmental rate, DNA methylation, and transcript levels of genes, all of which are pivotal to lineage specification and blastocyst formation. At the pronuclear stage, somatic nucleus-transplanted oocytes treated with 5 µM SAHA presented higher histone acetylation at H3K9, H3K14, H4K16 and H4K12, compared to untreated clones (p < 0.05). At the morula stage, cloned embryos treated with 5 μM RG108 or 5 μM SAHA presented lower DNA methylation intensity compared to untreated clones (p < 0.05), resembling the intensity levels of fertilized embryos. However, these effects were not observed when RG108 and SAHA were used in combination. The rate of morula formation was significantly higher in cloned embryos treated with 5 µM SAHA than in untreated clones, whereas treatment with RG108 resulted in no obvious effects on morula formation rates. On the other hand, the combined treatment with RG108 and SAHA resulted in inferior rates of cloned morula formation, compared to untreated clones. At the blastocyst stage, the aberrant expression levels of key developmental genes Oct4 and Cdx2, but not Nanog, were corrected in cloned embryos by the treatment with RG108. This is similar to the intensity levels seen in fertilized embryos. The expression of Rpl7l1 gene was significantly higher in embryos treated with both RG108 and SAHA than in untreated and in control groups. In summary, the present study showed that SAHA and RG108, when applied separately, improve the rate and quality of cloned mouse embryos.
Introduction
A popular method for producing cloned animals is Somatic Cell Nuclear Transfer (SCNT) into oocytes. Despite numerous studies conducted on various mammalian species, SCNT continues to be considered inefficient. Problems include cloned embryo death at pre- and post-implantation stages (Ogonuki Citation2002; Sayaka et al. Citation2008), high rate of abortion during early gestation and after birth, abnormal placentas (Hill Citation2014), liver necrosis, and tumors and pneumonia (Santos Pereira et al. Citation2020). Poor cloning efficiency is often attributed to incomplete reprogramming of the nucleus transplant (Whitworth and Prather Citation2010), which is mediated by epigenetic factors operating on the chromatin (Totonchi et al. Citation2007). These factors are crucial for the proper pre- and post-implantation development of the cloned embryos. Among the epigenetic mechanisms, histone acetylation and DNA methylation are considered critical factors in the transcriptional regulation of gene expression during early mammalian development (Whitworth and Prather Citation2010). Increasing evidence suggests that histone acetylation and DNA methylation are defective in cloned embryos (Ogura et al. Citation2013; Long et al. Citation2014; Niemann Citation2016), and resultant gene expression is abnormal, and abnormal gene expression profiles have been observed in the placenta and live cloned animals (Sim et al. Citation2017). These abnormal epigenetic traits and gene expression patterns are associated with the overall low success rates of cloning (Kishigami et al. Citation2006). To improve the developmental competence of cloned embryos, studies have targeted epigenetic remodeling using small molecules such as DNA methylation inhibitor 5-aza20-deoxycytidine (5-aza-dC) (Ding et al. Citation2008; Tsuji et al. Citation2009), Histone DeACetylase inhibitor (HDACi), TrichoStatin A (TSA) (Himaki et al. Citation2010; Inoue et al. Citation2015), valproic acid (Lv et al. Citation2020), scriptaid (Wen et al. Citation2014), and sodium butyrate (Xiong et al. Citation2015). SAHA (Suberoylanilide Hydroxamic Acid) is a novel HDACi that has been reported to significantly increase the production of healthy cloned offspring (Sun et al. Citation2017). RG108 or N-Phthalyl-L-tryptophan has been reported to be a strong, specific, and non-nucleoside DNA methyltransferase inhibitor (Zhai et al. Citation2018). Interestingly, this inhibitor need not be incorporated into the DNA strands and merely blocks the active site of the enzyme. Unlike RG108, nucleoside inhibitors such as 5-aza2'-deoxycytidine and zebularine must be incorporated into the DNA strands to trap DNMTs and are therefore considered toxic (Brueckner et al. Citation2005).
To the best of our knowledge, there are no published studies in which cloned embryos were treated with both SAHA and RG108 to mimic the DNA methylation and acetylation patterns of fertilized embryos. In this context, we have examined the effects of SAHA and RG108 on SCNT efficiency of mouse embryos. The objective was to understand how these two small molecules ameliorate the level of DNA methylation and histone acetylation modifications of cloned mouse embryos at the morula stage. This, in turn, would help understand the importance of these parameters in improving cloning efficiency. We hypothesized that combining these two small molecules as epigenetic modifiers would allow for better development of the cloned embryo.
Results
Effects of SAHA on the dynamic histone modifications of pronuclear stage cloned embryos
Since SAHA is a histone deacetylase inhibitor, we examined the dynamics of reacetylation of H3K9, H3K14, H4K12 and H4K16 in nucleus-transplanted oocytes at the 3rd, 6th and 10th h after activation (). No acetylation of H3K9 was observed 3 h after SCNT (). Following oocyte activation (6th and 10th h), acetylation of H3K9 was more intense in the pseudo-pronuclei of clones treated with SAHA than in the untreated clones (p < 0.05, ). Similar to H3K9, the acetylation of H3K14 showed a different pattern in SAHA-treated vs. untreated cloned embryos. Re-acetylation occurred faster in the former, being detected at 3 h post-activation in SAHA-treated embryos than 6 h post-activation in untreated clones. In terms of signal intensity, H3K14 acetylation was stronger in SAHA-treated clones at 3, 6, and 10 h post-activation than untreated clones (p < 0.05, ).
Figure 1. Dynamic modification of H3K9 acetylation in pseudo-pronuclei of cloned embryos. A. H3K9 acetylation of clone (a–c) and clone + SAHA (d–f) 3 h, 6 h and 10 h after activation. (a´–f´) DNA staining of the same oocytes is shown. The artifacts in these figures were shown with the letter A. Scale Bar: 10 µm. B. H3K9 acetylation level of clone and clone + SAHA embryos after image J analysis. H3K9 acetylation 6 and 10 h after activation was significantly higher in the clone + SAHA group than in the clone group (p < 0.05). Error bar; standard error of mean. Data were analyzed using t-test. (a,b) Significant differences between clone and clone + SAHA groups (p < 0.05).
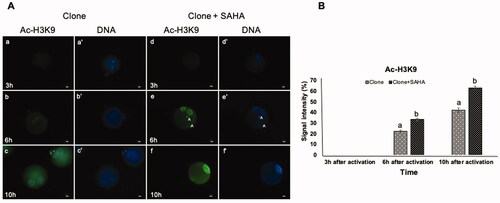
Figure 2. Dynamic modification of H3K14 acetylation in pseudo-pronuclei of cloned embryos. A. H3K14 acetylation of clone (a–c) and clone + SAHA (d–f) 3 h, 6 h and 10 h after activation. (a´–f´) DNA staining of the same oocytes is shown. The artifacts in these figures were shown with the letter A. Scale Bar: 10 µm. B. Quantitative data analysis using Image J also showed a significant increase in H3K14 acetylation in the clone + SAHA group 3, 6, and 10 h after activation (p < 0.05). Data were analyzed using T-test. Error bar; standard error of mean. (a,b) Significant differences between clone and clone + SAHA groups (p < 0.05).
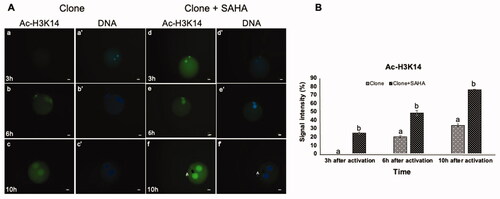
Figure 3. Dynamic modification of H4K12 acetylation in pseudo-pronuclei of cloned embryos. A. H4K12 acetylation of clone (a–c) and clone + SAHA (d–f) 3 h, 6 h and 10 h after activation. (a´–f´) DNA staining of the same oocytes is shown. The artifacts in these figures were shown with the letter A. Scale Bar: 10 µm. B. H3K12 acetylation severity was significantly increased in clone + SAHA embryos at 3, 6 and 10 h after activation (p < 0.05). Error bar; standard error of mean. Data were analyzed using t-test. (a,b) Significant differences between clone and clone + SAHA groups (p < 0.05).
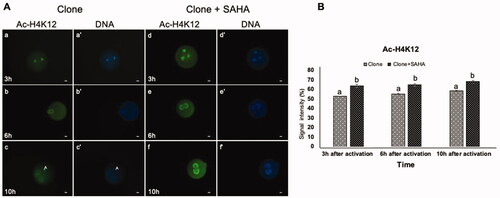
Figure 4. Dynamic modification of H4K16 acetylation in pseudo-pronuclei of cloned embryos. A. H4K16 acetylation of clone (a–c) and clone + SAHA (d–f) 3 h, 6 h and 10 h after activation. (a´–f´) DNA staining of the same oocytes is shown. The artifacts in these figures were shown with the letter A. Scale Bar: 10 µm. B. H416 acetylation intensity was significantly increased in clone + SAHA embryos 6 and 10 hours after activation compared to the untreated group (P < 0.05). Error bar; standard error of mean. Data were analyzed using t-test. (a,b) Significant differences between clone and clone + SAHA groups (p < 0.05).
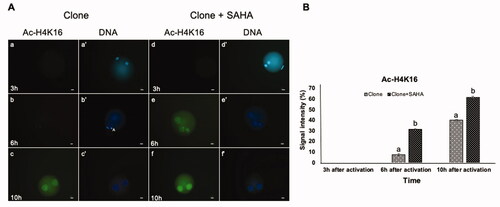
We also examined the acetylation of H4K12 and H4K16. In contrast to the re-acetylation observed for H3K14, the pattern for H4K12 was similar in the pseudo-pronuclei of SAHA-treated clones than the untreated controls (). H4K16 reacetylation was stronger and occurred earlier in the pronuclei of SAHA-treated cloned embryos, at 6 hours post-activation, compared to 10 hours post-activation in untreated clones (p < 0.05, ).
DNA methylation levels in cloned embryos
Since RG108 is a DNA methyltransferase inhibitor, we examined the level of DNA methylation in morula-stage cloned embryos (72 h) that were treated with different concentrations – 5 and 10 µM – of RG108 during development from two cell stage. Cloned embryos treated with 5 µM of RG108 showed a higher rate of development (43.33 ± 2.64). Based on these results, we selected 5 µM as the optimum concentration of RG108 and used it in the following experiments. The intensity of methylation in morula stage embryos of fertilized, untreated and treated cloned groups was evaluated by ELISA at 450 nm (). The results of signal quantification showed that there were significant differences between the methylation intensity of the morula stage embryos from the fertilized control group (19.1 ± 1.7) and those of the untreated cloned embryos (30.84 ± 3.01). As expected, this rate was higher in the cloned embryo group than in the fertilized control group. Methylation intensity in the cloned groups treated with 5 μM SAHA (19.69 ± 2.11) and 5 μM RG108 (18.76 ± 1.33) was significantly lower than in the untreated cloned group (p < 0.05) and was similar to that of the fertilized control group. The DNA methylation levels in cloned morula that were treated with SAHA and RG108 (32.51 ± 5.19) were not significantly different from those of the untreated cloned group ().
Figure 5. Differences in DNA methylation intensity of morula-stage embryos in untreated clones, clones treated with RG108 and SAHA (either separately or together), vs. fertilized embryos. As the diagram shows, methylation intensity in the cloned group treated with RG108 and cloned group treated with SAHA was significantly lower than that of the untreated cloned group (p < 0.05) and similar to the fertilized control group. Data were analyzed using Dunnett. values with different superscripts (a with b) are significantly different (p < 0.05).
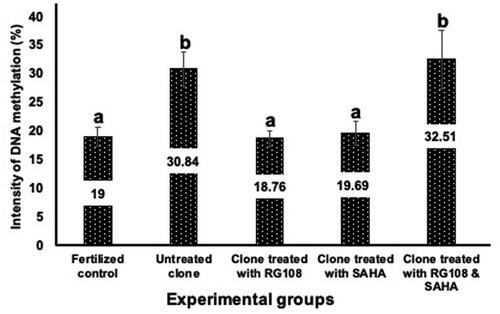
Evaluation of embryo development
The rates of development of the cloned embryos up to the morula stage in the treated and untreated groups are shown in . There was no difference between the experimental groups in the reconstructed oocytes that survived and reached the 2PN and 2-cell stages. Likewise, the proportions of the 2-cell stage that progressed to the morula stage in the group treated with RG108 and the untreated group were similar (43.33 ± 2.64 vs 50.35 ± 1.52). In contrast, the proportion of morulae was significantly higher in the cloned group treated with 5 µM SAHA than in the untreated cloned group (64.22 ± 3.21). On the other hand, the extent of development in the cloned group under the two treatments, RG108 and SAHA, was significantly reduced (27.53 ± 2.43) compared to the group treated with RG108 and SAHA and the untreated clone ().
Table 1. Developmental potential of cloned mouse embryos treated with 5 µm RG108 and 5 µM SAHA.
Assessment of development and blastocyst formation related- gene expression
In this experiment, the control group was designed to fertilized embryos. Gene expression analysis revealed that the embryo group treated with RG108 had Oct4 mRNA expression similar to the fertilized control group. On the other hand, the cloned embryos (3.25 ± 0.57) treated with SAHA (3.51 ± 0.11), and both molecules (3.13 ± 0.26) had significantly increased Oct4 mRNA expression compared to the fertilized control group ().
Figure 6. Transcript expression of key developmental genes in morula-stage embryos of untreated clones, clones treated with RG108 and SAHA, and fertilized controls. RG108 treatment of cloned embryos decreased the expression of the Oct4 gene to the level of the fertilized control group. 18 s rRNA served as the internal control. The data were analyzed using one‐way analysis of variance, followed by Tukey’s test. values with different superscripts (a with b) and (c with d) are significantly different (p < 0.05).
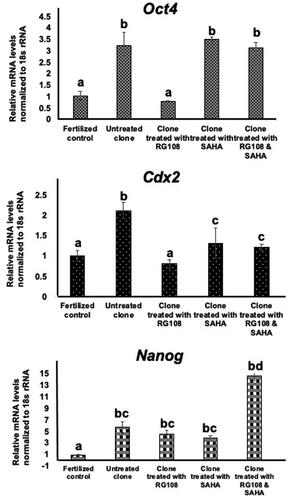
Cdx2 mRNA expression was significantly increased in the untreated clone group (2.12 ± 0.2) compared to the fertilized control group (p < 0.05). Cdx2 mRNA expression was decreased in the cloned embryos treated with SAHA (1.32 ± 0.37), RG108 (0.81 ± 0.1), and both together (1.22 ± 0.07) compared to the untreated cloned group. This decrease was significant in the RG108-treated cloned group (p < 0.05, ).
Nanog mRNA expression was significantly higher in untreated (5.72 ± 0.32) and cloned groups that were treated with SAHA (3.82 ± 0.51), RG108 (4.56 ± 0.61) and both drugs together (14.62 ± 0.85) compared to the fertilized control group (p < 0.05). Expression of Nanog was also significantly increased in the cloned group treated with both small molecules compared to the other treated cloned groups (p < 0.05, ).
The relative gene expression of Rpl7a in the fertilized control group showed significant differences from those of the cloned groups treated with RG108 (1.76 ± 0.1) and those treated with both drugs together (3.85 ± 0.42, p < 0.05). There were no significant differences between the untreated groups (1.01 ± 0.21), the groups treated with RG108 (1.76 ± 0.1) and the groups treated with SAHA (1.76 ± 0.62) ().
Figure 7. Transcript expression of the markers Rrp7a and Rpl7l1 in morula-stage embryos of untreated clones, clones treated with RG108 and SAHA, and fertilized controls. 18 s rRNA served as the internal control. The data were analyzed using one‐way analysis of variance, followed by Tukey’s test. values with different superscripts (a with b) and (c with d) are significantly different (p < 0.05).
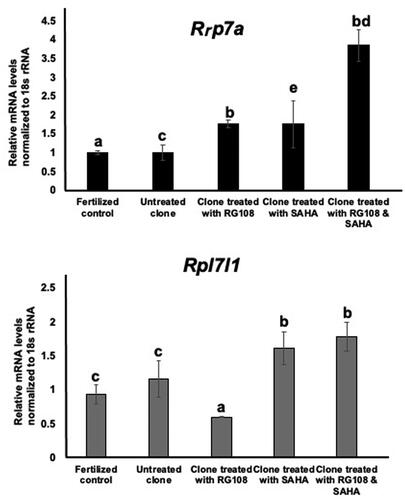
There were no significant differences in gene expression of Rpl7l1 between the fertilized control group and the cloned untreated group (1.16 ± 0.27), the group treated with RG108 (0.6 ± 0.01), the group treated with SAHA (1.62 ± 0.24) and the group treated with both small molecules (1.78 ± 0.21). The gene expression of Rpl7l1 in the clone group treated with RG108 showed a significant decrease compared to the clone treated with SAHA and the clone treated with both small molecules (p < 0.05, ).
Discussion
There are numerous differences between the cloned and fertilized embryos, which become visible as early as the pseudo-pronuclei phase, a few hours after SCNT and are related to acetylation dynamics (Yamanaka et al. Citation2009). If the cloned embryos resembled the fertilized embryos more closely, then there is better chance of implantation and subsequent post-implantation development. This study examined the dynamic histone acetylation and modification of lysine residues on histones 3 and 4 in the first cell cycle of conventional SCNT embryos compared to SCNT embryos treated with SAHA. We tested if the reprogramming of histone acetylation in cloned mouse embryos could be improved by treating them with histone deacetylase inhibitors and SAHA, which may help to improve the development of the cloned embryos. Most of the lysine histone residues are deacetylated in the condensed chromosomes with the resumption of meiosis and maintained until MII (Gu et al. Citation2010). When the cumulus cell nucleus was injected into the enucleated metaphase II oocytes, H3K9 and H4K16 were deacetylated within 3 hours of SCNT. Re-acetylation of these histone lysine residues occurs during the activation of the reconstructed oocytes with a higher signal intensity in cloned embryos treated with SAHA compared to untreated cloned embryos. Re-acetylation of H3K14 detected at 3 h post-activation in SAHA-treated embryos, compared to 6 h post-activation in untreated clones. After activation, re-establishing histone acetylation in the cloned embryos is important for gene activation in cloned embryos (Wang et al. Citation2007). In contrast, H4K12 undergoes partial deacetylation upon somatic cell nuclear transfer to the oocyte, as observed in the study of Wang et al. Citation2007. Yoshida et al. (Citation2007) showed that H4K12 was acetylated when the sperm was injected into an oocyte. It appears that SAHA treatment of cloned embryos can improve acetylation levels in the pseudo-pronuclei of cloned embryos to mimic normal embryo development.
Cloned embryos present higher levels of DNA methylation than fertilized embryos produced in vivo (Teperek and Miyamoto Citation2013). In the mouse zygote, the male pronucleus is rapidly demethylated after fertilization by an active mechanism, while the female pronucleus is passively demethylated in the early cleavage stage embryo (Iqbal et al. Citation2011). Global DNA methylation is at its lowest level at the morula stage and de novo methylation occurs at the blastocyst stage (Carlson et al. Citation1992). Cloned embryos receiving somatic nuclei, also undergo global DNA demethylation, although this demethylation has not been completed at the late 1 cell stage and there is aberrant hypermethylation in morula stage (Zhang et al. Citation2021). Incomplete DNA methylation reprogramming disrupted the developmental potential of cloned embryos (Gao et al. Citation2018). Several studies have examined the efficacy of methyl transferase inhibitors on somatic cells before the transplantation of the nucleus. Zhai et al (Citation2018) showed that cleavage and blastocyst rates in the cloned embryos constructed from RG108-treated donor fibroblasts were significantly higher than those in the control embryos.
Contrary to these reports, in the present study, it was found that the rate of development up to the morula stage was not significantly different in the cloned group treated with 5 μM RG108 compared to the untreated cloned group. Thus, it appears that the treatment of cloned embryos with 5 μM RG108 does not increase the number of embryos that develop to the morula stage. However, unlike this small molecule, the highest rate of embryo development to the morula stage was seen in the group treated with SAHA. SAHA increased the developmental rate by about 20% compared to RG108 and this increase is significant compared to the other groups. In order to further improve the efficiency of SCNT, the present study used DNMTi (RG108) and HDACi (SAHA) to treat the cloned embryos. When the cloned embryos were treated with both molecules simultaneously, the results differed from those seen when the two molecules were added separately. In the former case (two molecules together), we observed the lowest success rate in embryo development. It has been earlier reported that the use of TSA and 5-aza- deoxycytidine to treat the donor cell prior to SCNT improved the developmental potential of the derivative cloned embryos in bovine (Wang et al. Citation2011). In order to further improve the efficiency of SCNT, Fang et al used DNMTi (Zebularine) and HDACi (Scriptaid) to treat the cloned embryos after activation (Fang et al. Citation2019). Results from this earlier study indicated that treatment with both zebularine and scriptaid improved the developmental potential in sheep, achieving a similar blastocyst rate as their IVF counterparts. Similar results have also been reported in bovine models upon using TSA and 5-aza-deoxycytidine (Wang et al. Citation2011). For the first time, this study used RG108 to treat cloned mouse embryos from two-cell to morula stage. RG108 and SAHA appear to prevent the useful activity of each on embryo development. Tsuji et al. (Citation2009) examined the effect of TSA and 5-aza-2′-deoxycytidine on cloned mouse embryos. These researchers treated embryos with 5-aza- deoxycytidine for 48 h starting from the 2-cell stage, when the bulk of embryonic genome activation has already occurred, until the morula stage (Tsuji et al. Citation2009). The ability of cloned embryos treated with 100 nM 5-aza-deoxycytidine to develop into morula was significantly lower than for control embryos; and no fetuses were obtained after transfer of 5-aza-deoxycytidine treated embryos to recipients. These data showed that 5-aza-2′-deoxycytidine was toxic to preimplantation of mouse embryos, which is a similar effect seen in somatic cells. We evaluated the toxicity of RG108 on the in vivo embryo viability. This small molecule did not have negative effects on the morula formation rate of fertilized 2-cell embryos. Therefore, it appears that this reduction only occurs in the reprogramming system, possibly via interactions of RG108 and SAHA with unspecified reprogramming players.
Our results also showed that the level of DNA methylation in the cloned embryos was significantly higher than that of the control embryo produced by fertilization. The DNA methylation level of cloned morula after treatment with RG108 decreased compared to the untreated embryos. Our results show that RG108 as a DNA methyltransferase1 inhibitor acts successfully in reduction of methylation a normal rate in the treated cloned groups. Although RG108 did not increase the rate of cloned embryo development to morula, it affected the quality of these embryos by decreasing the DNA methylation intensity. Therefore, the correction of hypermethylation may itself not be responsible for efficient development. Other epigenetic modifications such as a high level of histone acetylation and DNA methylation correction may be required. The results of methylation intensity in the SAHA-treated cloned group were similar to those in the RG108-treated group, which probably indicates the effect that this histone deacetylase inhibitor could have on the Dnmt1 enzyme. The results of a study by Arzenani et al. (Citation2011) have shown the reduction of DNA methylation levels using another histone deacetylase inhibitor (TSA). The mechanism of this decrease was reportedly the decrease of the protein level of the Dnmt1 enzyme due to ubiquitin-dependent degradation, as well as a decrease in its nuclear expression and the association of Dnmt1 with chromatin. Another study by Januchowski et al. (Citation2007) reported that TSA in the jurkat T cell line reduced the level of expression of Dnmt1. Changes in DNA methylation at the overall level and specific genes as well as the decrease in Dnmt1 at both mRNA and protein levels, have also been demonstrated by TSA in human cancer cell lines (Kar et al. Citation2014). The methylation intensity of the cloned group treated with both small molecules did not decrease and remained similar to the untreated cloned group. The high methylation level of embryos in this group also confirms the low rate of embryo development up to the morula stage.
Blastomeres begin to differentiate molecularly at the 8-cell/morula stage into cells of the future inner cell mass (ICM) and trophectoderm (TE). In a somatic nucleus transferred to the ooplasm, the reactivation of Oct4 mRNA from the silent somatic state is a key clue for assessing the reprogramming rate. Oct4 is not expressed in somatic cells (Lengner et al. Citation2007); thus, it could be a good handle for evaluating the reprograming for pluripotency of differentiated cells. We wondered whether the silent somatic allele of Oct4 gene undergoes transformation into the active state following transfer to the ooplasm. The abnormal expression of this gene in cloned embryos could be directly and indirectly related to the developmental potential and the abnormality of other essential genes. Apart from other genes, variable expression and instability in the Oct4 level are the causes of the defects observed in the cloned embryos (Boiani et al. Citation2005). Our results are in line with the observations of Sebastiano et al. (Citation2005), who showed that the level of Oct4 expression in cloned mouse embryos was significantly higher than in vitro fertilized embryos. We showed that RG108 treatment of mouse cloned embryos decreased the expression of the Oct4 gene to the level of the control group. It seems that the treatment exerts a ‘correcting’ effect on Oct4 expression in cloned mouse embryos. In 2018, Wong et al. showed that 5-aza-dC and TSA treated cloned blastocysts shared the same Oct4 expression profile as the IVF embryos. Oct4 expression was not reduced in cloned embryos after both SAHA treatment and treatment with the two small molecules compared to untreated cloned embryos.
Furthermore, the expression of Cdx2 and Nanog mRNAs in the cloned embryos at the morula stage was higher than that of the control group. Our results are consistent with those obtained by Mallol et al. (Citation2015), who showed that levels of Cdx2 expression in cloned embryos are higher than those of the ICSI group. Similar to Oct4, Cdx2 expression was decreased only in the group of cloned embryos treated with RG108. A report by Balbach et al. (Citation2012) on the expression level of this gene in the morula stage compared with embryos obtained from ICSI is also in line with the data obtained in our study. The team of Balbach et al. reported that the expression level of Cdx2 had a much wider distribution in the morula stage cloned mouse embryos (Balbach et al. Citation2012). Furthermore, the expression of Nanog is regulated by the expressions of Cdx2 and Oct4. Therefore, as expected, due to the high expression and efficient performance of these two genes in regulating Nanog expression, the expression of Nanog in cloned embryos was higher than that of the control group. The treatment of cloned embryos with RG108, SAHA, and both small molecules together did not decrease the expression of Nanog. Interestingly, the expression pattern of pluripotent genes in cloned mouse embryos was not like that of cloned ovine embryos. The expression of the Oct4 and Cdx2 genes in ovine cloned embryos was lower than that of the control group, which increased and reached the expression level of these genes in the control embryos after treatment of cumulus cells with zebularine (5 nanomolar) and Scriptaid (0.2 millimolar). We found that treatment of early cloned embryos with RG108 affected the expression of Oct4 and Cdx2 (development-related genes) at the morula stage.
The genes involved in the early lineage specification were investigated in the morula stage in this study. Further assessment is needed to determine the function and potential of the cloned embryos in their transition from the morula to the blastocyst stage. Researchers who have studied the mentioned properties in the blastocyst stage have ignored the fact that many cloned embryos are not able to transit from the morula to the blastocyst stage. Compared to the control group, the expression of the key genes (Rrp7a and Rpl7l1) that are important for blastocyst formation was normal in the cloned embryos. These genes may have the same regulatory mechanisms as somatic cells, mature oocyte, and pre-implantation embryos. In fact, they are not reprogrammed, so there is no need to change active expression statuses to inactive positions or vice versa.
Further experiments are needed to test the effects of SAHA and RG108 on the developmental rate and development-related genes at the blastocyst stage. The present study showed that treatment with SAHA and RG108 alone – but not in combination – improved cloned mouse embryos’ developmental rate and gene expression. We postulate that this is due to the negative reciprocal interactions of RG108 and SAHA a and interactions with yet unidentified reprogrammers.
Materials and methods
Chemicals and animals
All chemicals used in this study were obtained from the Sigma Chemical Company (USA) unless otherwise indicated.
Eight to ten-week-old B6D2 F1 female mice (C57BL/6 × DBA/2 hybrids) were used as oocyte and somatic cell donors. As a control group, in vitro fertilized embryos were obtained from the mating of F1 females with DBA2 males (backcross). The mice were obtained from Razi Institute (Tehran, Iran) and maintained according to the guidelines of the animal welfare research ethics committee of Royan Institute.
Preparation of matured oocytes, donor cells and fertilized embryo
B6D2F1 female mice were superovulated by intraperitoneal injection of 7.5 IU of pregnant mare serum gonadotropin (PMSG; Intervet, Belgium) followed 48 h later by 7.5 IU human chorionic gonadotropin (hCG; Organon, Holland). Cumulus-oocyte complexes (COCs) were collected from the oviducts 15 h after hCG injection. Then COCs were treated with 50 IU hyaluronidase in a 100 L drop of HEPES-buffered CZB medium (H-CZB) at 37 °C until the cumulus cells were dispersed. The oocytes that showed good morphology were selected and cultured in αMEM medium containing 2 µg/mL bovine serum albumin (BSA) (MP Biomedical LLC, France) at 37 °C in an atmosphere of 5% CO2 in air until they were used. The dispersed cumulus cells were left in the hyaluronidase drop, diluted with an equal volume of αMEM with 2 mg/ml BSA, and stored at 4 °C until the injection of their nuclei into the enucleated oocytes.
To obtain in vivo fertilized morula stage embryos, B6D2 superovulated females were mated with DBA2 males after hCG administration. The embryos were collected from their oviducts after 66 h post hCG and were cultured in αMEM medium at 37 °C under an atmosphere of 5% CO2 in air.
Enucleation and nuclear transfer
Nuclear transfer experiments were performed after preparation of oocytes and donor cells, with an invert microscope (Nikon Ti, Japan) equipped with micromanipulation system (Eppendorf, Japan) and piezo (Prime Tech Model PMM-150U). Metaphase II oocytes were stripped of the chromosomal spindle (‘enucleation’) to create recipients for SCNT, as follows. Oocytes were treated with 5 µg/mL cytochalasin B (CB) dissolved in H-CZB medium containing 0.1% polyvinylpyrrolidone (PVP) (Calbiochem, Germany) for 7 min. Metaphase II spindles were aspirated using a piezo-driven pipette with an inner diameter of 6–8 µm. Enucleated oocytes were rinsed extensively in medium not containing CB, and then returned to the αMEM medium in the CO2 incubator.
After a 30 min recovery, enucleated oocytes were transferred to H-CZB medium containing 1% PVP. Nucleus-donor cells were disrupted, and their nuclei were injected individually into the enucleated oocytes using a piezo-driven pipette with an inner diameter of 5–7 µm. Reconstructed oocytes were then washed and incubated for 1 h at room temperature (at least 25 °C) in a 1:1 mix of αMEM medium with 2 mg/ml BSA and H-CZB medium with 1% (w/v) PVP.
Activation of reconstructed oocytes and embryo culture
Reconstructed oocytes were activated for 6 hours in Ca2+-free-αMEM supplemented with 10 mM strontium, 2 mg/mL BSA, and 4 µg/mL CB. The reconstructed oocytes were then carefully rinsed and cultured in αMEM supplemented with 2 mg/mL BSA and 10 µg/mL gentamicin. The experiments were carried out at a constant temperature of 37 °C in a 5% CO2 in air medium.
Experimental design
To determine the effects of SAHA (Cayman, Europe) treatment on histone modifications in pseudo-pronuclei and understand the developmental rate and gene expression, the reconstructed oocytes were allocated randomly to ‘treated’ and ‘non-treated’ groups. The treated group was first exposed to 5 µM SAHA in the activation medium from the beginning of activation (3 and 6 h) and then exposed for additional 4 h in αMEM medium (10 h, clone + SAHA group) (the optimal treatment concentration of SAHA was determined in our previous study (Shamaghdari et al. Citation2013)). At the end of the 10-h treatment, embryos were divided into two groups: one fixed for the analysis of histone acetylation, the other washed and cultured in regular αMEM medium until the morula stage. Untreated controls were cultured as described above except for the absence of SAHA. In vivo fertilized morula stage embryos were used as a control for evaluation of developmental gene expression.
To determine the effects of RG108 on the developmental rate up until the morula stage, DNA methylation intensity, and the expression of genes involved in linage specification and blastocyst formation, 2-cell embryos were allocated randomly to treated and non-treated groups, each of which was subjected to the following treatment. The treated embryos were transferred to αMEM supplemented with 2 mg/mL BSA, 0.01 mg/mL gentamicin, and 5 µm RG108 and kept for 48 h until the morula stage. Untreated controls were kept in regular αMEM until the morula stage, without renewing the medium. In vivo fertilized morula stage embryos were used as controls.
To determine the combined effect of SAHA and RG108 on cloned embryos, 2-cell embryos developed from SCNT oocytes were treated with 5 µM SAHA for 10 h, then transferred to αMEM medium supplemented with 5 µM RG108 further culture (48 h) to the morula stage.
Analysis of histone acetylation
The method used for indirect immunofluorescence was adapted from Wang et al. (Citation2007) with some modifications. All steps were performed at room temperature, unless otherwise mentioned. The embryos were fixed with 4% paraformaldehyde for at least 20 min, and then permeabilized for 30 min with 0.2% Triton X-100 in PBS. After three washings, all samples were incubated in a blocking solution (1% BSA and 0.05% Tween-20 in PBS) overnight at 4 °C. Oocytes were incubated with the first antibodies to H3K9 acetylation (Upstate, 06-942 1:250), H3K14 acetylation (Millipore, 06-911, 1:250), H4K12 acetylation (Millipore, 06-1352, 1:400) and H4K16 acetylation (Millipore, 07-329, 1:250) for 1 h. After three washings, the oocytes were incubated with fluorescein isothiocyanate (FITC, Nordic Immunology, 6643) conjugated goat anti-rabbit second antibody (1:1000) for 1 h. Finally, the DNA was stained with 4’,6-diamidino2-phenylindole (DAPI, 1 µg/ml) and all the samples were mounted in anti-fade solution. At least ten oocytes were processed for each separate sample and the experiments were replicated at least four times.
Quantitative evaluation of DNA methylation by ELISA method
In this method, the detection was based on the adhesion of the extracted DNA to the bottom of the plate coated with a primary antibody against 5-methyl cytosine, followed by a secondary antibody conjugated to the enzyme, against the primary antibody. By providing the conjugated enzyme substrate with a secondary antibody, the reaction between the enzyme and the substrate begins. As a result of the reaction, a yellow color is produced and the color intensity at 450 nm – which represents the intensity of methylated spots on the genome – is detected by an ELISA reader. The procedure for measuring total genomic methylation according to the protocol of Methylamp Global DNA Methylation Quantification Kit (EPIGENTEK, USA) was performed for morula stage embryos. The following formula was used to calculate the extent of methylation as percentage:
OD: Optical Density
RNA isolation, cDNA synthesis and qRT-PCR
For each group, about 15 morula was randomly chosen, pooled and stored at −80 °C. The evaluation of the gene expression level was replicated using three independent biological reactions. Total RNA was extracted using the RNasey Micro Kit (QIAGEN, Germany) according to the manufacturer’s protocol. The extracted RNA was treated with QIAGEN RNA-free DNase I to digest the genomic DNA in the samples. The cDNA was synthesized and amplified using the RevertAidTM H Minus First Strand cDNA Synthesis Kit (Fermentas, Germany) under the following conditions. RT reaction was set up with 11 µL embryo lysate and 1 µL Random primer, which was incubated at 70 °C for 5 min. The following components were added to the reaction to final volume of 20 μl: 5X Reaction Buffer, dNTPs Mix (10 mM), RNase Inhibitor (20 U/µL), Reverse Transcriptase (20 U/µl). The 20 μL volume was reverse transcribed as follows: (i) 25 °C for 10 min (ii) 42 °C for 1 h (iii) 70 °C for 10 min. The reactions were assembled on ice and before each incubation, the mixtures were mixed gently and centrifuged to collect the contents at the bottom of the vessel. The cDNA was stored at −20 °C.
Quantitative real-time reverse transcription-polymerase chain reaction (qRT-PCR) was run to determine the expression levels of the candidate genes. All gene transcripts were quantified through absolute quantitative RT-PCR using SYBR Green PCR Master Mix (Ampliqon, Germany). All PCR reactions were performed in duplicate in a 20 µL reaction volume in an ABI prism 7500 Sequence Detection System (Applied Biosystem, USA). Each reaction contained 1 µL cDNA template, 10 µL SYBR Green Premix, 1 µL both PCR forward and reverse (5 pmol/mL) and 7 µL dH2O. Thermal cycling conditions were initiated for 15 min at 95 °C to activate hot-start Taq polymerase followed by 40 PCR cycles of denaturation at 94 °C for 15 s, then 60 °C for 30 sec and extension at 72 °C for 30 s. The oligonucleotide primers used are listed in . Each gene intensity value was normalized to 18 s as endogenous housekeeping gene. The 2−ΔΔCT method was used to quantify the relative mRNA levels using the following formula.
Table 2. Primer sequences used in qRT-PCR and amplification product sizes.
The mean of all samples was determined, and for each gene, average expression levels were reported. Melting curve analysis and gel electrophoresis on a 1.7% agarose gel were used to confirm the PCR reaction's specificity (single melting peak, single electrophoretic band). dH2O replaced cDNA for the negative controls in the real time reaction tubes.
Statistical analysis
All statistical analyses were performed using SPSS16+ software (SPSS Inc; Chicago. IL, USA). The histone acetylation intensity was quantified using the Image J software and analyzed by chi square. The DNA methylation level and cell number average were analyzed using Dunnett and One-Sample t-test. The Mann–Whitney U-test analyzed the data on the developmental rate. The levels of gene expression were tested using ANOVA, followed by Tukey’s test. Data were presented as mean ± SD. Differences were considered significant at p < 0.05.
Ethics approval for animal study
All procedures were performed in accordance with the institutional guidelines on animal experimentation and care and were approved by the Ethical Committee at Royan Institute (IR.ACECR.ROYAN.REC.1394.130).
Authors’ contributions
Preparation of samples, experimental design, data collection and analysis and drafting the manuscript: MZ and BSH; Sample preparation for molecular test: ZV; Contributed to the conception and design of study and editing and final approval of manuscript: AD and PEY.
Abbreviations | ||
SAHA | = | Suberoylanilide hydroxamic acid |
SCNT | = | somatic cell nuclear transfer |
5-aza-dC | = | 5-aza20-deoxycytidine |
HDACi | = | histone deacetylase inhibitor |
TSA | = | Trichostatin A |
qRT-PCR | = | Quantitative real-time reverse transcription-polymerase chain reaction |
ICM | = | Inner cell mass |
TE | = | Trophectoderm |
hCG | = | Human chorionic gonadotropin |
COCs | = | Cumulus-oocyte complexes |
H-CZB | = | HEPES-buffered CZB medium |
BSA | = | Bovine serum albumin |
CB | = | cytochalasin B |
PVP | = | polyvinylpyrrolidone |
DAPI | = | 4’,6-diamidino2-phenylindole |
Acknowledgment
The authors are thankful to statistic epidemiology and laboratory Animal departments. We thank Dr. Michele Boiani for providing critical review.
Disclosure statement
No potential conflict of interest was reported by the authors.
Additional information
Funding
References
- Arzenani MK, Zade AE, Ming Y, Vijverberg SJ, Zhang Z, Khan Z, Sadique S, Kallenbach L, Hu L, Vukojević V, et al. 2011. Genomic DNA hypomethylation by histone deacetylase inhibition implicates DNMT1 nuclear dynamics. Mol Cell Biol. 31(19):4119–4128.
- Balbach ST, Esteves TC, Houghton FD, Siatkowski M, Pfeiffer MJ, Tsurumi C, Kanzler B, Fuellen G, Boiani M. 2012. Nuclear reprogramming: kinetics of cell cycle and metabolic progression as determinants of success. PLoS One. 7(4):e35322.
- Boiani M, Gentile L, Gambles VV, Cavaleri F, Redi CA, Schöler HR. 2005. Variable reprogramming of the pluripotent stem cell marker Oct4 in mouse clones: distinct developmental potentials in different culture environments. Stem Cells. 23(8):1089–1104.
- Brueckner B, Garcia Boy R, Siedlecki P, Musch T, Kliem HC, Zielenkiewicz P, Suhai S, Wiessler M, Lyko F. 2005. Epigenetic reactivation of tumor suppressor genes by a novel small-molecule inhibitor of human DNA methyltransferases. Cancer Res. 65(14):6305–6311.
- Carlson LL, Page AW, Bestor TH. 1992. Properties and localization of DNA methyltransferase in preimplantation mouse embryos: implications for genomic imprinting. Genes Dev. 6(12B):2536–2541.
- Ding X, Wang Y, Zhang D, Wang Y, Guo Z, Zhang Y. 2008. Increased pre-implantation development of cloned bovine embryos treated with 5-aza-2'-deoxycytidine and trichostatin A. Theriogenology. 70(4):622–630.
- Fang X, Xia W, Cao H, Guo Y, Wang H, Zhang X, Wan P, Liu C, Wei Q, Sun S, et al. 2019. Effect of supplemetation of Zebularine and Scriptaid on efficiency of in vitro developmental competence of ovine somatic cell nuclear transferred embryos. Anim Biotechnol. 8:1–9.
- Gao R, Wang C, Gao Y, Xiu W, Chen J, Kou X, Zhao Y, Liao Y, Bai D, Qiao Z, et al. 2018. Inhibition of aberrant DNA re-methylation improves post-implantation development of somatic cell nuclear transfer embryos. Cell Stem Cell. 23(3):426–435.
- Gu L, Wang Q, Sun QY. 2010. Histone modifications during mammalian oocyte maturation. Cell Cycle. 9(10):1942–1950.
- Hill JR. 2014. Incidence of abnormal offspring from cloning and other assisted reproductive technologies. Annu Rev Anim Biosci. 2:307–321.
- Himaki T, Yokomine TA, Sato M, Takao S, Miyoshi K, Yoshida M. 2010. Effects of trichostatin A on in vitro development and transgene function in somatic cell nuclear transfer embryos derived from transgenic Clawn miniature pig cells. Anim Sci J. 81(5):558–563.
- Inoue K, Oikawa M, Kamimura S, Ogonuki N, Nakamura T, Nakano T, Abe K, Ogura A. 2015. Trichostatin A specifically improves the aberrant expression of transcription factor genes in embryos produced by somatic cell nuclear transfer. Sci Rep. 5:10127.
- Iqbal K, Jin S-GP, Pfeifer GE, Szabó P. 2011. Reprogramming of the paternal genome upon fertilization involves genome-wide oxidation of 5-methylcytosine. Proc Natl Acad Sci U S A. 108(9):3642–3647.
- Januchowski R, Dabrowski M, Ofori H, Jagodzinski PP. 2007. Trichostatin A down-regulate DNA methyltransferase 1 in Jurkat T cells. Cancer Lett. 246(1-2):313–317.
- Kar S, Sengupta D, Deb M, Shilpi A, Parbin S, Rath SK, Pradhan N, Rakshit M, Patra SK. 2014. Expression profiling of DNA methylation-mediated epigenetic gene-silencing factors in breast cancer. Clin Epigenetics. 6(1):20.
- Kishigami S, Mizutani E, Ohta H, Hikichi T, Van Thuan N, Wakayama S, Bui HT, Wakayama T. 2006. Significant improvement of mouse cloning technique by treatment with trichostatin A after somatic nuclear transfer. Biochemic Biophys Res Commun. 340(1):183–189.
- Lengner CJ, Camargo FD, Hochedlinger K, Welstead GG, Zaidi S, Gokhale S, Scholer HR, Tomilin A, Jaenisch R. 2007. Oct4 expression is not required for mouse somatic stem cell self-renewal. Cell Stem Cell. 1(4):403–415.
- Long CR, Westhusin ME, Golding MC. 2014. Reshaping the transcriptional frontier: epigenetics and somatic cell nuclear transfer. Mol Reprod Dev. 81(2):183–193.
- Lv L, Lu X, Feng T, Rehman S, Sun J, Wu Z, Shi D, Liu Q, Cui K. 2020. Valproic acid enhances in vitro developmental competence of porcine handmade cloned embryos. Livest Sci. 233:103957.
- Mallol A, Santaló J, Ibáñez E. 2015. Improved development of somatic cell cloned mouse embryos by vitamin C and latrunculin A. PLoS One. 10(3):e0120033.
- Niemann H. 2016. Epigenetic reprogramming in mammalian species after SCNT-based cloning. Theriogenology. 86(1):80–90.
- Ogonuki N, Inoue K, Yamamoto Y, Noguchi Y, Tanemura K, Suzuki O, Nakayama H, Doi K, Ohtomo Y, Satoh M, et al. 2002. Early death of mice cloned from somatic cells. Nat Genet. 30(3):253–254.
- Ogura A, Inoue K, Wakayama T. 2013. Recent advancements in cloning by somatic cell nuclear transfer. Philos Trans R Soc Lond B Biol Sci. 368(1609):20110329.
- Santos Pereira LDR, Porto M, Reis Júnior JL, Rumpf R, Silva Júnior ER, Soto-Blanco BL, Câmara ACJ, Borges JR. 2020. Pathological features of cloned calves that died in the neonatal period. Pesq Vet Bras. 40(11):852–862.
- Sayaka W, Satoshi K, Van Thuan N, Hiroshi O, Takafusa H, Eiji M, Thuy BH, Masashi M, Teruhiko W. 2008. Effect of volume of oocyte cytoplasm on embryo development after parthenogenetic activation, intracytoplasmic sperm injection, or somatic cell nuclear transfer. Zygote. 16(3):211–222.
- Sebastiano V, Gentile L, Garagna S, Redi CA, Zuccotti M. 2005. Cloned pre-implantation mouse embryos show correct timing but altered levels of gene expression. Mol Reprod Dev. 70(2):146–154.
- Shamaghdari B, Dalman A, Zarei M, Amiri-Yekta A, Hadi M, Eftekhari-Yazdi P. 2013. Gene expression and developmental state of mouse cloned embryos after treatment with histone deacetylase inhibitor, suberoylanilide hydroxamic acid (SAHA). 14th Royan Congress on Reproductive Biomedicine and 8th Royan Nursing and Midwhfery Seminar. Int J Fertil Steril. 7 (Supplement 1):81.
- Sim B-W, Park C-W, Kang MH, Min KS. 2017. Abnormal gene expression in regular and aggregated somatic cell nuclear transfer placentas. BMC Biotechnol. 17(1):34.
- Sun JM, Cui KQ, Li ZP, Lu XR, Xu ZF, Liu QY, Huang B, Shi DS. 2017. Suberoylanilide hydroxamic acid, a novel histone deacetylase inhibitor, improves the development and acetylation level of miniature porcine handmade cloning embryos. Reprod Domest Anim. 52(5):763–774.
- Teperek M, Miyamoto K. 2013. Nuclear reprogramming of sperm and somatic nuclei in eggs and oocytes. Reprod Med Biol. 12(4):133–149.
- Totonchi M, Shahhoseini M, Moghadam MM, Baharvand H. 2007. Stem cell epigenetic. Yakhteh. 9(1):51–66.
- Tsuji Y, Kato Y, Tsunoda Y. 2009. The developmental potential of mouse somatic cell nuclear-transferred oocytes treated with trichostatin A and 5-aza-2'-deoxycytidine. Zygote. 17(2):109–115.
- Wang F, Kou Z, Zhang Y, Gao S. 2007. Dynamic reprogramming of histone acetylation and methylation in the first cell cycle of cloned mouse embryos. Biol Reprod. 77(6):1007–1016.
- Wang Y, Su J, Wang L, Xu W, Quan F, Liu J, Zhang Y. 2011. The effects of 5-aza-2'- deoxycytidine and trichostatin A on gene expression and DNA methylation status in cloned bovine blastocysts. Cell Reprogram. 13(4):297–306.
- Wen BQ, Li J, Li JJ, Tian SJ, Sun SC, Qi X, Cai WT, Chang QL. 2014. The histone deacetylase inhibitor Scriptaid improves in vitro developmental competence of ovine somatic cell nuclear transferred embryos. Theriogenology. 81(2):332–339.
- Whitworth KM, Prather RS. 2010. Somatic cell nuclear transfer efficiency: how can it be improved through nuclear remodeling and reprogramming? Mol Reprod Dev. 77(12):1001–1015.
- Xiong XR, Lan Dl, Li J, Wang Y, Zhong JC. 2015. Sodium butyrate improves the cloned yak embryo viability and corrects gene expression patterns. Zygote. 23(1):19–26.
- Yamanaka K, Sugimura S, Wakai T, Kawahara M, Sato E. 2009. Acetylation level of histone H3 in early embryonic stages affects subsequent development of miniature pig somatic cell nuclear transfer embryos. J Reprod Dev. 55(6):638–644.
- Yoshida N, Brahmajosyula M, Shoji S, Amanai M, Perry AC. 2007. Epigenetic discrimination by mouse metaphase II oocytes mediates asymmetric chromatin remodeling independently of meiotic exit. Dev Biol. 301(2):464–477.
- Zhai Y, Zhang Z, Yu H, Su L, Yao G, Ma X, Li Q, An X, Zhang S, Li Z. 2018. Dynamic Methylation changes of DNA and H3K4 by RG108 improve epigenetic reprogramming of somatic cell nuclear transfer embryos in pigs. Cell Physiol Biochem. 50(4):1376–1397.
- Zhang X, Gao S, Liu X. 2021. Advance in the role of epigenetic reprogramming in somatic cell nuclear transfer-mediated embryonic development. Stem Cells Int. 2021:6681337.