ABSTRACT
Microplastics (MPs) present a pressing environmental concern, posing formidable challenges to their effective elimination from water systems. Bioadsorbents offer a promising, eco-friendly, and cost-effective alternative to traditional removal methods. This review analyzes 206 research papers (2016-January 2024) to explore recent advances in bioadsorbent design and application for microplastic removal. We examine the sources and ecological impacts of MPs, then delve into the most studied bioadsorbents: biochar (51.9%), biomass-derived activated carbon (7.4%), synthetic sponges/aerogels (25.9%), and graphene-based materials (14.8%). The review critically analyzes factors influencing MP adsorption by these materials, including MP type and size, adsorbent properties, and experimental conditions. Reported removal efficiencies ranged from 31% to 100%, with polystyrene (52%) being the most common test polymer. Finally, the intricate mechanisms of MP-bioadsorbent interaction are discussed. We highlight key challenges and future research directions for optimising and scaling up bioadsorbent-based MP removal, acknowledging the early stage of this promising and sustainable approach.
1. Introduction
Microplastics (MPs) have become a serious environmental problem due to their irrefutable prevalence in air, soil, and particularly, the aquatic ecosystem (Nguyen et al. Citation2023). They are a major environmental problem, as they can be found in all parts of the world, including the oceans, rivers, and lakes which has resulted in a decrease in water quality over the last few decades (Senathirajah and Palanisami Citation2023). They are intentionally produced for commercial purposes, such as in cosmetics, or derived from the fragmentation and degradation of larger plastic debris (Arenas et al. Citation2021; Goh et al. Citation2022). Microplastics pose a worldwide concern, and in developing economies, their quantities are steadily rising, reaching an alarming level with each passing day (Egessa et al. Citation2020; Nantaba et al. Citation2021). As a result, MPs have emerged as a significant focal point in wastewater treatment research, primarily owing to their capacity to adsorb onto toxic substances, their nonbiodegradability, and their potential carcinogenic properties (Tumwesigye et al. Citation2023). Due to their small size, microplastics have the potential to infiltrate the human food chain via the consumption of seafood and terrestrial food items, potentially endangering human health and hindering economic development (Rose et al. Citation2023). The elimination of anthropogenic micropollutants, including those originating from various sources such as domestic, industrial, agricultural, and urban sectors, is recognised as one of the foremost global challenges today, as highlighted by (Sher et al. Citation2021). Consequently, a multidisciplinary approach, encompassing the restriction of MPs release and advancements in removal technologies, is imperative for the effective management of environmental preservation and the well-being of humankind.
Although wastewater treatment plants (WWTPs) are presently not specifically designed for microplastic removal, several studies suggest that conventional treatment technologies such as grit chambers, primary sedimentation, dissolved air flotation, coagulation, and membrane disc-filters can improve removal of MPs (Bayo, Olmos, and López-Castellanos Citation2020; Goh et al. Citation2022). Nevertheless, due to the substantial quantities processed and the reuse of sludge through land application, these conventional wastewater treatments still release MPs into both aquatic and terrestrial ecosystems (Lv et al. Citation2019; J. Sun et al. Citation2019).
Utilisation of biomass-derived adsorbents in water pollution control has been studied and reported by several researchers (Adeleye et al. Citation2023; Galvão et al. Citation2021; Hao et al. Citation2021; Olugbenga et al. Citation2023; Soffian et al. Citation2022). This is mainly due to their microporous form of carbon with a well-developed pore structure, pore volume, high internal surface area, and consequently a high adsorption capacity (Galvão et al. Citation2021). They have functional groups that can be used as-prepared or after chemical modification to provide binding sites for adsorption of various contaminants in water prompting its application as a potential alternative to the conventional and advanced water treatment technologies (Ngeno et al. Citation2022). Commercially, adsorbents such as activated carbons have been widely obtained from non-renewable sources such as coal, lignite, and peat. These exhibit a low mineral content, high carbon content, as well as a high porosity, which suit them as precursors for the production of adsorbents (Jjagwe et al. Citation2021). However, due to the limited and non-renewable nature of coal, the development of adsorbents from other low-cost renewable resources or any solid waste rich in carbon, such as biomass, has become a research focus (Mohammaad and Kijevcanin Citation2023).
Biomass materials are mostly composed of carbon-rich chemical compounds, which make them more suitable for the production of bioadsorbents (Jjagwe et al. Citation2021). Furthermore, in developing countries such as Uganda, agricultural waste and aquatic biomass, present a huge potential as precursors for the production of bioadsorbents due to their wide availability, low-cost and their carbonaceous nature (Mohammaad and Kijevcanin Citation2023; Santoso et al. Citation2020). lists the biomass materials used for microplastic removal. illustrate the various biomass-derived adsorbents employed in these processes and the distribution of polymers utilised in different experiments, respectively. The polymers include Polystyrene (PS), Polyhydroxyalkanoate (PHA), Polyethylene (PE), Polyamide (PA), Polypropylene (PP), Nylon (NYL), Polyvinyl Chloride (PVC), High-Density Polyethylene (HDPE), and unspecified polymers (NS).
Figure 2. Polymers employed in microplastic removal experiments.
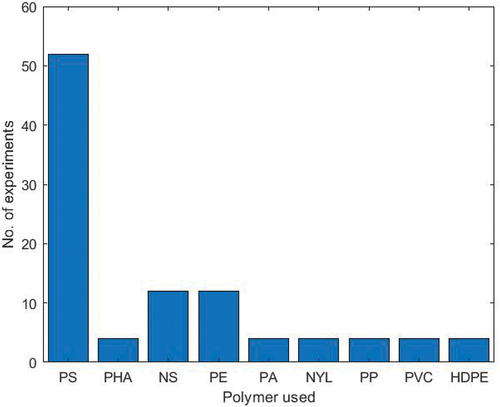
Table 1. Biomass materials used for microplastics removal.
With the sagacious selection of biomass materials, preparation methods, and heedful control of preparation and adsorption conditions, biomass-derived adsorbents exhibit superior adsorption performance, as compared to their commercial counterparts (Tran et al. Citation2019).
This article highlights the growing importance of biomass-derived adsorbents for microplastic (MP) removal from water ecosystems. We present the first comprehensive review on this topic, aiming to elucidate both the removal mechanisms and the factors influencing efficiency. By thoroughly discussing these aspects, we identify knowledge gaps and propose promising future research directions in this field.
2. Methodology
Databases of Google Scholar and Web of Science were searched through selected keywords to obtain original articles, review articles, book chapters, and scientific reports for review purposes to contribute to this work. The keywords were searched individually and/or in combinations of up to three terms. The most prevalent keywords included bioadsorbents such as activated carbon and biochar, agricultural waste materials, water treatment, adsorption kinetics, microplastic removal, biomass materials for microplastic removal, isotherms, and mechanisms, physical activation, chemical activation, thermal carbonisation, contaminants of emerging concern such as microplastics, and regeneration. Boolean operators AND, “, and () were mainly employed to obtain the most relevant articles of the search subject. Studies were mainly selected if the adsorbent under investigation was from biomass waste(s). Another selection criterion was based on the general aspects that cut across all bioadsorbents such as material suitability for bioadsorbent production, pretreatment, carbonisation, and activation, as well as adsorption mechanisms. Due to the vast amount of literature available concerning the production of bioadsorbents from biomass-based materials, most articles published from 2016 to date were considered. To gain a more comprehensive understanding of the field’s evolution, we extended our search beyond the 2016-January 2024 timeframe for relevant studies. We utilised databases like PubMed, Web of Science, and Scopus to identify earlier research published before 2016. Our search terms included ‘microplastic removal’, ‘microplastic adsorption’, and ‘properties of bioadsorbents for microplastic removal from water ecosystems’. This broader approach yielded three valuable pre-2016 studies exploring the potential of biomass materials as Micropollutant adsorbents. Articles published before 2016 were only included if; 1) they were deemed necessary to support/contradict other studies, and 2) the contents were exclusive and worth highlighting. Titles of over 400 papers were scanned and about 300 papers were screened before relevant scientific publications amounting to 206 papers were eventually selected for this review. In this study, a total of 103 papers reviewed focused on microplastic removal. These papers offered valuable insights into removal efficiency, mechanisms, and the various factors influencing the adsorption of microplastics onto biomass-derived adsorbents.
2.1. Bioadsorbents for removal of microplastics from aquatic ecosystems
Bioadsorbents are natural or modified materials, typically of biological origin, that possess the capability to adsorb or bind various substances, including pollutants, contaminants, or specific molecules from aqueous systems (Volesky Citation2007). Bioadsorbents utilise functional groups or biological components to enhance the adsorption process, making them valuable tools in applications aimed at removing or recovering specific substances such as microplastics from complex mixtures (Volesky Citation2001). Several bioadsorbents that have been used to remove microplastics from aquatic ecosystems have been investigated. This section critically examines the most common biomass-derived adsorbents and their removal efficiencies for microplastics.
2.2. Biomass-derived activated carbon
Activated carbon (AC) is a highly porous form of carbon that has been processed to improve its pore structure, pore volume, internal surface area, and consequently adsorption capacity (Galvão et al. Citation2021; Hao et al. Citation2021; Lu et al. Citation2020). The AC also exhibits functional groups on its surface, which influences the solution pH, and consequently the adsorption process (Alharbi et al. Citation2020; Jjagwe et al. Citation2021). Deployed across a diverse spectrum of applications, including water treatment and purification, activated carbon capitalises on adsorption mechanisms, encompassing both physisorption and chemisorption, to effectively transfer contaminants from the aqueous phase to the solid surface (Dai, Liu, et al. Citation2020; Lima et al. Citation2016). Its outstanding adsorption capacity and ease of use position it as a compelling alternative to conventional and advanced water treatment technologies (Liu et al. Citation2020), promising significant advancements in the field.
ACs have been mostly made from non-renewable sources such as coal, lignite, and peat, however, within developing countries, agricultural residues like maize cobs and aquatic biomass such as water hyacinth hold substantial promise as precursors for the production of activated carbon (Thakur et al. Citation2020). This is attributed to their abundance, affordability (Santoso et al. Citation2020; Tran et al. Citation2019), and their carbonaceous composition (Karri, Sahu, and Meikap Citation2020; Kaur, Kaur, and Kaur Citation2020). Additionally, using biomass wastes to make ACs provides a more sustainable way to dispose of waste than open burning or landfilling (El Naga et al. Citation2019).
Studies on the removal of MPs from aquatic ecosystems using biomass-derived ACs are still in their early stages. However, the results so far are promising. Arenas et al investigations reported a maximum adsorption capacity and removal efficiency of 6.33 mg/g and 98%, respectively for polystyrene (PS) when employing Granular Activated Carbon (GAC) derived from coconut shells (Arenas et al. Citation2021), as shown in . Furthermore, Siipola et al., discovered that activated carbons derived from pine bark treated with 30% and 40% steam exhibited exceptional retention capabilities for the larger microplastics that were examined (Siipola, Romar, and Lassi Citation2022).
Figure 3. (a) Effect of initial PS nanoplastics concentration and contact time on the adsorption capacity of 5 g/L GAC. (b) Effect of initial PS nanoplastics concentration on the removal efficiency at 5 g/L GAC. Reproduced with permission of http://creativecommons.org/licenses/by-nc-nd/4.0/. from a study by Arenas et al., (Citation2021). Nanoplastics adsorption and removal efficiency by granular activated carbon used in drinking water treatment process. Science of the Total Environment, 791, 148175. Retrieved from https://doi.org/10.1016/j.Scitotenv.2021.148175.
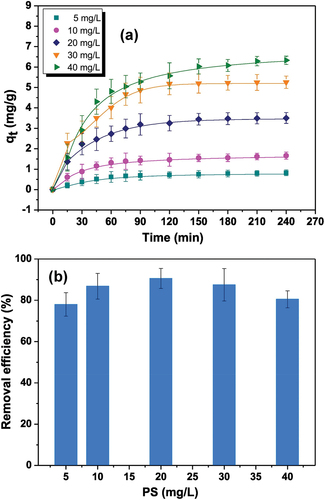
In a separate investigation, the utilisation of commercial GAC alongside thermal regeneration, resulted in an impressive 92.8% removal efficiency for MPs exhibiting a specific gravity lower than that of water, and falling within the size range of 20–50 µm. Furthermore, within the same study, it was observed that GAC treatment offered distinct advantages in the removal of MPs with a lower specific gravity than water, including polyethylene and polypropylene, while also proving effective across a diverse range of MP sizes (Kim and Park Citation2021). Additionally, regarding the efficiency of MPs removal, Pivokonský et al., achieved an impressive 88% cumulative removal rate by equipping the drinking water treatment plant with a standard GAC filtration system (Pivokonský et al. Citation2020).
Currently, there is a notable gap in research pertaining to the removal of MPs from aquatic ecosystems using biomass-derived activated carbon. There exists a compelling urgency to delve into the effectiveness of chemically modified biomass-derived activated carbons for the removal of MPs. Thus, I advocate for the initiation of studies aimed at evaluating the removal efficiency of a broader range of microplastic polymers, extending beyond just polystyrene, by utilising activated carbons derived from various biomass feedstocks. As such, it becomes imperative to embark on a comprehensive investigation into the removal of MPs using activated carbons sourced from biomass.
2.3. Biochar-based adsorbents
The utilisation of biochar as a novel adsorbent material has gained considerable attention. Biochar, a highly porous and carbon-rich substance produced through biomass pyrolysis (Dong et al. Citation2023), has shown great promise in adsorbing and removing MPs from aquatic ecosystems. An investigation by Ganie and co-authors reported a removal efficiency of 46% when bagasse biochar pyrolysed at 550 °C was used to remove MPs from aquatic systems and this was due to its porous structure and high surface area (Ganie et al. Citation2021). A removal efficiency greater than 70% of the polystyrene (PS) microspheres from an aqueous solution was shown when magnetic biochar was used (Wang, Sedighi, and Lea-Langton Citation2020). Furthermore, (Wang et al. Citation2021a) reported that Iron-modified biochar has magnetic extractability to easily and quickly remove MPs from aquatic ecosystems. Similarly (Singh et al. Citation2021), examined the removal of MPs using corncob biochar, and a removal efficiency of 80% was reported. Kumar et al also investigated the adsorption of MPs under various environmental conditions using biochars synthesised from different biomasses, including corn straw, hardwood, pine and spruce bark, and corncob. They reported removal efficiencies greater than 60% for all the materials, for non-specified MPs (Kumar et al. Citation2023). Additionally, when biochars derived from jujube biomass was investigated for nylon and polyethylene removal from contaminated water in a fixed-bed column, a removal efficiency greater than 80% of MPs was reported (Ahmad et al. Citation2023). Several investigations into the application of biochars derived from diverse biomass sources for polystyrene microplastic removal have consistently showcased remarkable efficacy (Ganie et al. Citation2021), reported a removal efficiency of 99%, while (Shi et al. Citation2023a) achieved 95.2%. Similarly (Hanif et al. Citation2023), and (Li et al. Citation2023) both observed a removal efficiency of 97% and (Wu et al. Citation2022) demonstrated a removal efficiency of 99%. The use of biochar for MPs removal is still in its infancy, but the initial results are encouraging. With further research and development, biochar surfaces can be chemically modified to increase their surface area, hydrophobicity, and affinity for MPs, making it more effective at removing microplastics from aquatic ecosystems. Exploratory studies investigating how biochar can effectively eliminate microplastics from aquatic ecosystems in the last five years are summarised in .
Table 2. Studies on biochar efficacy in microplastics removal from aquatic environments.
2.4. Chemically synthesized sponge/aerogel biomass-derived materials
Sponge refers to a kind of material with 3D porous structured network (Wang et al. Citation2021b). Utilising chemically synthesised sponge materials proves highly efficient in the removal of diverse water pollutants (Risch and Adlhart Citation2021; Sun et al. Citation2020, Citation2021). Additionally, recent research has explored the use of plant protein-based materials as source materials for the production of sponge materials, aimed at adsorbing MPs present in aquatic environments (Pan et al. Citation2023). In their research study, Wang et al chemically cross-linked plant proteins to create a green sponge material with high mechanical properties that are both natural and biodegradable (Z. Wang et al. Citation2021b) ().
Figure 4. SEM images of the cross-section of Oat protein sponges (OPS) coded according to 0, 2%, 4%, and 6% (w/v) NaCl (OPS-0 (a), OPS-2 (b), OPS-4 (c) and OPS-6 (d) respectively. Reproduced with permission from Elsevier from a study by Wang et al. (Citation2021b). Fatigue resistance, re-usable and biodegradable sponge materials from plant protein with rapid water adsorption capacity for microplastics removal. Chemical Engineering Journal, 415, 129006. https://doi.org/10.1016/j.cej.2021.129006.
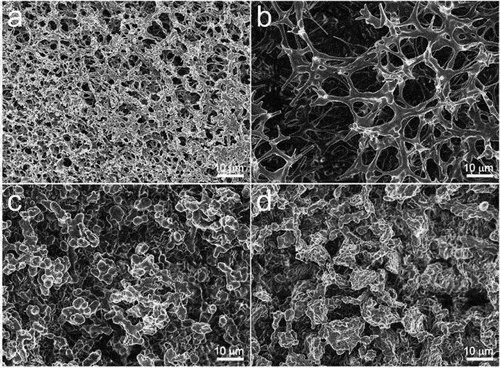
Figure 5. SEM images of (a1, a2) original Loofah sponge LS; (b1, b2) Loofah sponge/beeswax LS-B(1) and Loofah sponge palm wax LS-P(1); (c1, c3) LS-B(2) and (c2, c4) LS-P(2). Reproduced with permission from Elsevier from a study by Ha et al. (Citation2023). Loofah plant—Derived biodegradable superhydrophobic sponge for effective removal of oil and microplastic from water. Environmental Technology & Innovation, 32, 103265. https://doi.org/10.1016/j.eti.2023.103265.
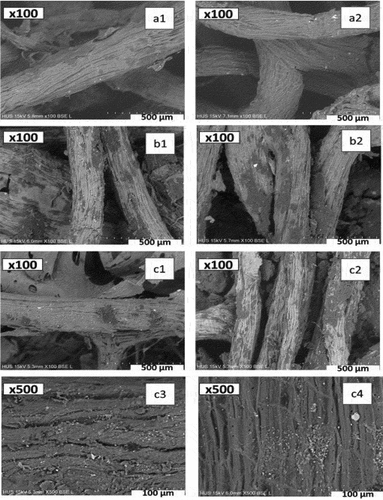
The protein sponge’s exceptional adsorption capacity for MPs, achieving an impressive removal efficiency of up to 81.2%, was attributed to the numerous highly reactive side chains found on the amino acid residues (Wang et al. Citation2021b). Additionally, chitin-based sponges combined with O-C3N4 and chitosan were used to create sponge materials, all of which exhibited satisfactory performance and could be reused. Each of these materials achieved a removal efficiency exceeding 70% for pure polystyrene, carboxylate-modified polystyrene and amine-modified polystyrene MP particles (Sun et al. Citation2020, Citation2021). Moreover, two varieties of environmentally friendly super hydrophobic sponges, sourced from the loofah plant were investigated for their capacity to eliminate MPs from aquatic environments, yielding a removal rate exceeding 80% for polystyrene MPs (Ha et al. Citation2023). To attain a more comprehensive understanding of the adsorption capabilities of sponges derived from biomass in real water systems, future research should explore the elimination of MPs with varying sizes and distinct chemical characteristics, such as charge and functional groups. Studies showcasing the use of sponges derived from biomass for MP removal in the period between 2018 and 2023 are summarised in .
Table 3. Studies showcasing the use of sponges derived from biomass for MP removal.
2.5. Biomass-based graphene materials
Graphene oxide (GO) has garnered significant interest owing to its outstanding characteristics, serving as a biosensor (Kafi, Paul, et al. Citation2020), biomaterial (Kafi, Aktar, et al. Citation2020), and an effective adsorbent for metal ions and organic pollutants. This efficacy arises from the abundant presence of oxygen atoms on its surface, primarily in the form of epoxy, hydroxyl, and carboxyl groups (Sheng et al. Citation2018; Sun et al. Citation2020; Yuan et al. Citation2020). Yuan and co-authors investigated the elimination of MPs from water using three-dimensional reduced graphene oxide as the adsorbent, and they found that it achieved a remarkable adsorption capacity of 617.28 mg/g for microplastics (Yuan et al. Citation2020). Peng et al. examined the removal of polystyrene using graphene-like carbon-assembled layered double-oxide material and reported an adsorption capacity and efficiency of polystyrene of 209.39 mg/g and 80%, respectively (Peng et al. Citation2022). In his research group, Dey examined the removal of high-density polyethylene (HDPE)-based MPs from wastewater using a graphene oxide-polyvinyl alcohol-based composite membrane and reported a removal efficiency of 95% for HDPE-based MPs (Dey, Jamal, and Uddin Citation2023). Although there has been considerable interest in the potential of biomass-based graphene materials for the removal of MPs from aquatic ecosystems, it is evident that their utilisation remains largely underexplored and underutilised. Despite their promising adsorption capabilities and environmental benefits, there is a need for further research to harness the full potential of these materials for microplastics removal. A summary of the removal efficiencies for some of the biomass-derived adsorbents used for the removal of microplastics is presented in .
3. Insight into the interaction between microplastics and bioadsorbents
In recent years, the pervasive issue of MPs pollution in aquatic ecosystems has garnered increasing concern from scientists, environmentalists, and policymakers worldwide. As these small plastic particles continue to infiltrate our lakes, rivers oceans, and even drinking water sources, the need to develop effective strategies for their removal and mitigation has become paramount. One such strategy involves the use of bioadsorbents as natural or modified materials with the ability to attract and bind MPs. Despite the low solubility of microplastics (MPs) in water systems, their interaction with bioadsorbents can be facilitated through electron transfer mechanisms, leading to the formation of chemical bonds. Additionally, electrostatic attractions between oppositely charged ions, arising from the transfer of electrons between atoms, may give rise to ionic bonds. These electrostatic interactions are instrumental in the adsorption of microplastics onto bioadsorbent surfaces, thereby enhancing removal efficiency. This section endeavours to comprehensively investigate the interaction between adsorbents derived from diverse biomass sources and microplastics during the removal process. Furthermore, an examination of the interplay among various removal mechanisms is discussed.
3.1. π-π bond interaction
The π-π bond interaction, a weak force commonly observed between aromatic rings, facilitates the binding of the adsorbent and adsorbate through electron transfer between the donor and acceptor electrons. This mechanism serves as a prominent factor in the adsorption of various carbonaceous materials onto pollutants during the adsorption process (Cheng et al. Citation2021). In the study by Sun et al., a separate research project was conducted to address the removal of microplastics, including polystyrene (PS), carboxylate-modified polystyrene (PS-COOH), and amine-modified polystyrene (PS-NH2), from water. They accomplished this by employing an environmentally friendly adsorbent comprising chitin and Graphene oxide (ChGO). The study’s findings revealed the presence of π−π interactions between graphene oxide (GO) and the aromatic ring of microplastics (Sun et al. Citation2020). Similarly, Yuan et al. documented the presence of π-π interaction during the adsorption process of polystyrene microplastics onto a three-dimensional reduced graphene oxide matrix (3D RGO) (Yuan et al. Citation2020). The comprehension of π-π bond interactions within the context of microplastic removal from aquatic ecosystems using biomass-derived activated carbon remains relatively underexplored and warrants further in-depth research.
3.2. Electrostatic interactions
As outlined by Cheng et al., the core concept underpinning the formation of an ionic bond is electrostatic interaction, encompassing both attractive and repulsive forces. An ionic bond emerges as a chemical linkage resulting from the electrostatic attraction between anions and cations, which arises following the gain and loss of electrons by atoms (Cheng et al. Citation2021). Existing research has consistently demonstrated that electrostatic interactions are of paramount significance in the process of adsorbing micropollutants, such as microplastics, onto bioadsorbents (Chen et al. Citation2018; Paunovic et al. Citation2019). In a study conducted by Arenas et al., they investigated the adsorption capacity and removal efficiency of polystyrene microplastic particles using granular activated carbon (GAC) as the adsorbent material. Their findings revealed that, when exposed to ultrapure water, the primary mechanism responsible for the adsorption and subsequent removal of microplastics was the electrostatic attraction between the positively charged polystyrene microplastics and the negatively charged GAC (Arenas et al. Citation2021). In a parallel study, researchers explored the effectiveness of a biodegradable superhydrophobic sponge crafted from the loofah plant in removing polystyrene microplastics. Notably, at a pH of 6, the sponge’s surface exhibited a positive charge, while the polystyrene microplastics possessed a negative charge. This favourable electrostatic attraction between the two surfaces at pH 6 outperformed other pH conditions, indicating a promising potential for microplastic removal (Ha et al. Citation2023).
In a separate research endeavour, a comprehensive investigation was undertaken to explore the removal of microplastics, including polystyrene (PS), carboxylate-modified polystyrene (PS-COOH), and amine-modified polystyrene (PS-NH2), from water. This study employed an environmentally friendly adsorbent comprising chitin and Graphene oxide (ChGO). The findings shed light on the significant role of electrostatic interactions in the removal process, specifically between the amino group of chitin and the carboxyl group of PS-COOH, as well as among the carboxyl groups of Graphene oxide (GO) and the amino group of PS-NH2 (Sun et al. Citation2020). Furthermore, researchers studied the removal of imitated polystyrene (PS) microplastics from water, employing a three-dimensional reduced graphene oxide (3D RGO) structure. The results of the study revealed that 3D RGO exhibited a substantial and smooth graphite layer, facilitating a π–π effect that exerted an attractive force on the macromolecular PS microplastics, characterised by π electrons. Consequently, the adsorption of PS microplastics onto the 3D RGO surface was primarily attributed to the strong π–π interaction between the carbon ring of 3D RGO and the benzene ring present in the PS microplastics (Yuan et al. Citation2020). Moreover, Wang et al. proposed an efficient adsorbent comprising of Mg/Zn-modified magnetic biochar designed for the removal of polystyrene microplastic (PS-MPs). The study’s findings revealed that under neutral conditions, both PS microplastic and biochar adsorbents exhibited negative surface charge, consistent with prior (Maliwan, Pungrasmi, and Lohwacharin Citation2021; Zhao et al. Citation2021). Consequently, the adsorption of PS-MPs onto Mg/Zn modified magnetic biochar was attributed to the presence of the metal oxides and metal hydroxides attached to the biochar’s surface. These metal components exhibited a positive charge when in contact with water, thereby enhancing microplastic adsorption through electrostatic interaction (Wang et al. Citation2021a). While earlier studies have primarily concentrated on the removal of polystyrene microplastics using bioadsorbents, there is now an urgent need to explore the electrostatic mechanism in relation to a wide range of other microplastic polymers.
3.3. The interplay of hydrogen bonding interactions and functional groups
Functional groups which consist of specific atoms or atom groups, play a pivotal role in defining the chemical characteristics of organic compounds. These groups exhibit the ability to interact with each other, and some of them possess the capability to establish robust hydrogen bonds (Vijay, Zipse, and Sastry Citation2008). Due to their propensity for forming hydrogen bonds, these functional groups exhibit high bond energy, rendering them resistant to separation. Through the formation of hydrogen bonds, they have strong bond energy and are not easy to separate (Peng et al. Citation2022). Previous studies have shown that microplastics can be adsorbed onto bioadsorbent surfaces through the formation of hydrogen bonds.
In an independent research endeavour, scientists conducted a thorough investigation into the effectiveness of an environmentally friendly adsorbent, Chitin and graphene oxide (ChGO), for the extraction of microplastics, specifically encompassing polystyrene (PS), carboxylate-modified polystyrene (PS-COOH), and amine-modified polystyrene (PS-NH2), from water. The results of this study revealed remarkable hydrogen bond interactions between the oxygen-rich functional groups present in chitin or GO and the amine group within PS-NH2 as well as the carboxyl group within PS-COOH (Cheng et al. Citation2021). Likewise, the identification of a hydroxyl functional group on the modified corncob (HNO3/H2SO4) was observed to have a pivotal impact on the adsorption of Polystyrene microplastics (PS-MPs) onto biochar. This was attributed to the formation of hydrogen bonds, providing additional insight into why the adsorption of PS-MPs onto modified biochar surpasses that onto fresh biochar (Magid et al. Citation2021). Additionally, the adsorption of fluorescent-orange amine-modified polystyrene beads (fluo-NP, 100 nm) onto coffee grounds was driven primarily by hydrogen bonding interactions between the amine group on fluo-NP and the hydroxyl and carboxyl groups present on the coffee grounds. These interactions proved to be robust, operating effectively over a wide pH range (Yen et al. Citation2022). In another study, it was found that hydrogen bonding was also formed between Polystyrene microplastics (PS-MPs) and goethite, contributing to higher adsorption of PS-MPs on goethite than magnetite (Zhang et al. Citation2020). The effect of hydrogen bonding interactions and functional groups on microplastic adsorption using bioadsorbents represent a critical aspect of understanding and mitigating microplastic pollution in aquatic environments. These intricate interactions, often mediated by the surface chemistry of both microplastics and adsorbent materials, play a significant role in the fate and removal of microplastics from aquatic systems.
3.4. Hydrophobic interactions
When non-polar groups, such as C-H bonds, are present within a molecule, they induce a mutual repulsion among water molecules, giving rise to what is known as hydrophobic interaction, as described by (Xie et al. Citation2020). This hydrophobic interaction mechanism is also a prevalent phenomenon observed in the adsorption process of microplastics by bioadsorbents. Previous studies have explored the impact of hydrophobic iron nanoparticles, which were surface-modified with hexadecyltrimethoxysilane, on the extraction of microplastics from environmental water samples. The findings indicated that microplastics were effectively extracted, primarily due to the hydrophobic interactions (Grbic et al. Citation2019). In a groundbreaking study, Tang et al. achieved a significant milestone by synthesising magnetic carbon nanotubes (M – CNTs) to serve as adsorbents for the efficient removal of microplastics, including polyethylene (PE), polyethylene terephthalate (PET), and polyamide (PA) from aqueous solutions. The remarkable success in adsorbing microplastics with magnetic carbon nanotubes was primarily attributed to the compelling hydrophobic interactions at play (Tang et al. Citation2021). Additionally, hydrophobic interaction played a more important role in Polystyrene microplastic (PS-MPs) adsorption to fresh corncob biochar (Magid et al. Citation2021). llustrates potential mechanisms through which biochar may adsorb emerging pollutants, such as microplastics.
Figure 7. Possible mechanisms of biochar for emerging pollutants adsorption. Reproduced with permission of https://creativecommons.org/licenses/by/4.0/. from a study by Dong et al. (Citation2023). Biochar for the removal of emerging pollutants from aquatic systems: a review. International Journal of Environmental Research and Public Health, 20(3), 1679. https://doi.org/10.3390/ijerph20031679.
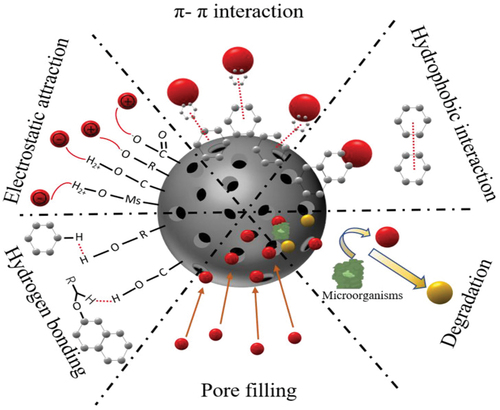
The investigation into hydrophobic interactions related to microplastic adsorption by bioadsorbents has yielded valuable insights regarding the potential effectiveness of these natural materials in combating microplastic pollution. However, there is still need to learn more about these interactions and how they work. Further research should delve deeper into the complexities of hydrophobic interactions, encompassing factors such as bioadsorbent surface modifications, particle sizes, polymers beyond polystyrene and environmental variables.
4. Factors affecting microplastic removal efficiency from aquatic ecosystems
The removal of MPs from aquatic environments using bioadsorbents is a complex process influenced by a myriad of factors, ranging from the physical and chemical properties of the particles themselves to environmental conditions and the presence of organisms. This multi-faceted issue demands a comprehensive exploration of the various factors affecting microplastics removal to develop effective strategies for mitigating their environmental impact. This section, therefore, delves into the key factors that influence the fate and removal of MPs from aquatic ecosystems.
4.1. Characteristics of bioadsorbents
Bioadsorbents with varying characteristics can lead to differing outcomes in their application for the remediation of polluted water (Dong et al. Citation2023). The properties of bioadsorbents are known to undergo alterations due to preparation methods, raw material type, modifications, and ageing techniques. These alterations result in differences in surface functional groups, pH levels, and specific surface areas (Guo et al. Citation2022; Meilani et al. Citation2021; Siipola, Romar, and Lassi Citation2022). Siipola and colleagues conducted a study on coconut shell biochar, examining its properties using various methods. They found that the specific surface area and pore volume achieved through supercritical water gasification of the biomass-based materials were significantly higher, increasing by 78-fold and 43-fold, respectively, compared to those obtained through pyrolysis (Siipola, Romar, and Lassi Citation2022). The performance characteristics of bioadsorbents have also been reported to be influenced by preparation conditions such as temperature. At elevated temperatures, Lui and colleagues observed a reduction in biochar’s polarity and hydrophilicity, accompanied by a gradual increase in its aromaticity and specific surface area (Liu et al. Citation2022). Additionally, when the pyrolysis temperature increased, the pH of four different biochar types exhibited a gradual slowing down rate at which it increased due to the breakdown of cellulose, hemicellulose, and lignin within the raw material (Cheng et al. Citation2021; Dong et al. Citation2023). In the study conducted by Ganie et al, different pyrolysis temperatures (350,550, and 750°C) were employed to produce bagasse biochar for the removal of MPs from aquatic environments. It was observed that biochar pyrolysed at 750°C exhibited the most effective removal of MPs, primarily attributed to its porous structure and extensive surface area (Ganie et al. Citation2021). Furthermore, Wang and co-authors noted that biochar produced at 500°C exhibited improved efficacy in removing and immobilising microplastics during the column transport tests due to the abundant honeycomb structures within the biochar ().
Figure 8. Microplastic immobilization mechanisms: (a) “stuck”, (b) “trapped”, and (c) “entangled”. Corresponding ESEM images: (d and g) sand filter; biochar filter (e, f, h) and optical microscope image (i). Reproduced with permission from Elsevier from a study by Zheng Wang, Majid Sedighi, Amanda Lea-Langton, Filtration of Microplastic Spheres by Biochar: Removal Efficiency and Immobilization Mechanisms, Water Research (Citation2020), https://doi.org/10.1016/j.watres.2020.116165.
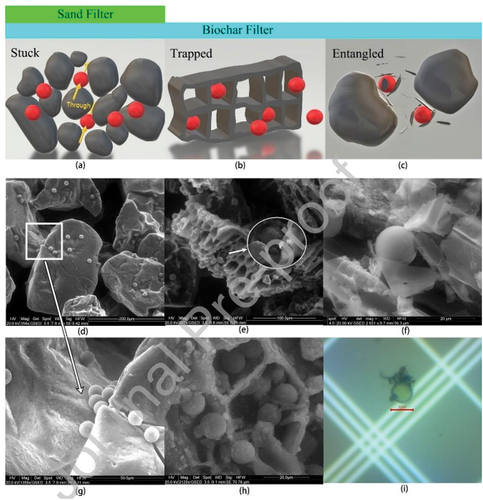
It is also worth emphasising that the efficiency of microplastics removal varied among bioadsorbents derived from distinct biomass sources (Ahmad et al. Citation2023; Hanif et al. Citation2023; Kumar et al. Citation2023; Pan et al. Citation2023; Wu et al. Citation2022). Exploring the influence of bioadsorbent characteristics on microplastics removal warrants additional research, particularly in investigating how bioadsorbent properties, such as biomass type, preparation methods, and conditions, affect the removal of microplastics from aquatic ecosystems.
4.2. Quantity of the adsorbent
The quantity of adsorbent plays a crucial role in removing pollutants from aquatic ecosystems (Dai, Wang, et al. Citation2020; Yen et al. Citation2022). Investigators examined the impact of adsorbing MPs from aqueous solutions using two sets of coffee ground concentrations, ranging from 5 to 50 g/l. The research, conducted at a pH level of 7, with a contact duration of 120 minutes and a fixed MPs concentration of 100 mg/L, revealed that as the coffee ground concentration decreased from 25 g/L to 5–25 g/L, MPs removal rates declined from 74% to a range of 53–66%. Furthermore, elevating the coffee ground concentration from 25 g/L to 50 g/L, did not augment nanoplastics (NPs) removal and led to a reduction in equilibrium adsorption capacity (Yen et al. Citation2022) as illustrated in . Arenas and the research team examined how varying the concentration of the adsorbent affected the adsorption of MPs across different levels of granular activated carbon (GAC) doses, ranging from 3 to 15 g/L while keeping the MPs’ concentration fixed at 30 mg/L. The findings indicated that MP adsorption occurred rapidly initially but gradually became less efficient as the adsorbent concentration increased. Moreover, the results demonstrated that the adsorption capacity (measured in mg/g) increased as the adsorbent concentration decreased, with the highest MP adsorption achieved at a GAC dose of 3 g/L (Arenas et al. Citation2021). Recent research indicates that elevating the adsorbent concentration can notably enhance the removal of microplastics by generating supplementary binding sites for the adsorbed particles (Kumar et al. Citation2023).
Figure 9. Effect of coffee grounds concentration on the removal of fluo-NP particles. Batch assays were carried out with a fluo-NP concentration of 100 mg/L, a pH of 7, and various concentrations of coffee grounds. The test tubes were shaken in an incubator for 120 min at 120 rpm and 25 ◦C. qe (mg/g) = the amount of fluo-NP adsorbed onto coffee grounds at equilibrium. The data represent the mean ± standard deviation of four replicates from two different sources of coffee grounds. Reproduced with permission from Elsevier from a study by Yen et al. (Citation2022). Removal of nano-sized polystyrene plastic from aqueous solutions using untreated coffee grounds. Chemosphere, 286, 131863. https://doi.org/10.1016/j.chemosphere.2021.131863.
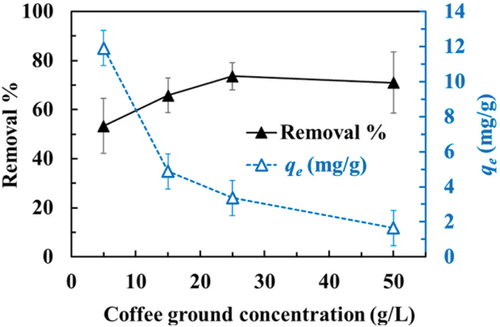
Nevertheless, an excessive amount of adsorbent can result in its clumping together, diminishing the number of accessible adsorption sites (Alghamdi et al. Citation2019; Qu et al. Citation2021; Zhang et al. Citation2019). In addition, at high concentrations of adsorbents, there is the possibility that MP particles might not completely cover the active sites as reported by Kausar and co-authors (Kausar et al. Citation2019). Another study demonstrated that elevating the biochar concentration from 2 g/L to 20 g/L resulted in a reduction of diclofenac adsorption by biochar, decreasing from 107% to 25% (Lonappan et al. Citation2018). According to (Weber and Quicker Citation2018), the agglomeration capacity of biochar was more dependent on the raw material. Biochar prepared from raw materials with high lignin content was more prone to agglomeration than biochar produced from raw materials with low lignin content. This could result from lignin’s naturally strong adhesion and its incomplete breakdown during pyrolysis (Föhr et al. Citation2017). Hence ascertaining the optimal quantity of the adsorbent is of paramount importance, not only for optimising its adsorption efficiency but also for guaranteeing the cost-effectiveness of its utilisation (Liang et al. Citation2016). Additionally, there is need to gain deeper insights into the adsorbent’s behaviour at various concentrations when tackling MPs other than polystyrene aquatic environments.
4.3. Surface chemistry and modification of bioadsorbents
Exploring surface chemistry and altering biomass-based adsorbents play crucial roles in the field of environmental science and engineering, especially when it comes to addressing pollution and implementing effective remediation strategies. These tailored adsorbents, sourced from natural materials, have shown impressive capabilities in capturing MPs from aquatic environments. Mg/Zn-modified magnetic biochar adsorbents from pinewood sawdust were assessed for their effectiveness in removing MPs. The results indicated removal efficiencies of 94.81%, 98.75%, and 99.46% for magnetic biochar, Mg-modified magnetic biochar, and Zn-modified magnetic biochar, respectively (Wang et al. Citation2021a). Similarly, oxidised corncob biochar exhibited a higher MP removal rate (>90%) compared to standard biochar, attributed to its greater abundance of hydroxyl groups (Singh et al. Citation2021). Wanga and colleagues conducted research focused on developing an exceptionally porous sponge by chemically modifying oat protein with epichlorohydrin. This innovative sponge demonstrated remarkable efficiency in removing MPs, achieving an impressive 81.2% removal rate at room temperature, mainly attributed to the formation of chemical crosslinks between distinct protein aggregates via their hydroxyl groups (Wang et al. Citation2021b). In a different investigation, the surface of a natural loofah sponge was effectively modified using a straightforward dip-coating method, which involved the application of two kinds of natural wax (bee wax and palm wax). The outcomes of this study demonstrated that the modified sponges exhibited an exceptional ability to capture MPs from aqueous suspensions under continuous stirring, achieving an adsorption capacity ranging from 381 to 569 mg/g and effectively removing over 99% of the MPs (Ha et al. Citation2023).
The removal can be understood by considering two distinct interactions occurring at the sponge surface and microplastic particles: Inter-particle interactions and the binding energy at the solid-liquid interface between the sponge and microplastic particles (Rius-Ayra et al. Citation2022). Additionally, simple and environmentally friendly magnetic biochars were successfully prepared by utilising ball-milling to combine biochar with Fe3O4 nanoparticles. This innovative approach aimed at eliminating both unmodified and amine-modified polystyrene MPs. The results revealed a removal efficiency of 43.67% for the unmodified MPs and an even higher efficiency of 82.73% for the amine-modified MPs (Shi et al., Citation2023b). During the same study, a removal efficiency of 57.02% was reported for carboxylate-modified MPs. The improved surface characteristics of the bioadsorbents after modification, which encompassed surface chemistry and charge, played a crucial role in efficiently eliminating MPs from aquatic environments (Dong et al. Citation2023; Lyu et al. Citation2020; Pan et al. Citation2023; Rong et al. Citation2022). The modification of bioadsorbents and the careful consideration of surface chemistry are pivotal strategies in the quest to remove MPs from aquatic ecosystems. These approaches leverage the natural adsorption capabilities of bioadsorbents while tailoring their surface to enhance interactions with MPs. By optimising surface properties, such as functional groups and charges, I can significantly enhance the efficiency and selectivity of MPs removal.
4.4. Initial microplastic concentration
One of the critical factors influencing the adsorption efficiency of adsorbents is the initial concentration of MPs. Arenas et al. conducted experiments to explore how the initial concentration of MPs affects their adsorption onto GAC and subsequent removal. The experiments were conducted under constant GAC concentration (5 g/L) but with varying initial MPs concentrations, ranging from 5 to 40 mg/L, in ultrapure water at a pH of 7.4 ± 0.1. The choice of a 5 g/L GAC concentration aimed to strike a balance between efficient removal, adsorption capacity, and favourable turbidity measurement conditions. The findings revealed that as the initial MPs concentration and contact time increased, the adsorption capacity in mg/g also increased (Arenas et al. Citation2021). This phenomenon can be attributed to the rising concentration gradient of MPs, which enhances the mass driving force effect, thus promoting the diffusion of MPs from the solution to the GAC (Yu et al. Citation2021). As illustrated in , it was observed that nanoplastics (NPs) exhibited rapid adsorption during the initial phase of the process, followed by a decrease in efficiency as the adsorption progressed. This trend aligns with the idea that the majority of adsorbent sites were accessible for adsorption at the outset, gradually reaching saturation as these sites became occupied over time (Qiu et al. Citation2022; Sun et al. Citation2022; Thang et al. Citation2019). In order to assess the maximum adsorption capacity, MPs removal was evaluated across a range of initial concentrations (25–200 mg/L), while maintaining a consistent set of conditions: pH at 7, a contact time of 120 minutes, and a coffee ground dosage of 25 g/L. As observed, in accordance with previous studies (Dai, Wang, et al. Citation2020; Huang, Yen, and Liao Citation2021; Yen et al. Citation2022), an increase in the initial MPs particle concentration corresponded to a higher equilibrium adsorption capacity for coffee grounds. Furthermore (Yuan et al. Citation2020), noted that as microplastic concentration increased, the efficiency of polystyrene microplastic removal declined, especially when the adsorption capacity approached saturation.
Figure 10. (a) Effect of initial fluo-NP concentration on fluo-NP adsorption using coffee grounds. Batch assays were carried out with a coffee grounds concentration of 25 g/L at a pH of 7, and various concentrations of fluo-NP. The test tubes were shaken in an incubator for 120 min at 120 rpm and 25 ◦C. qe (mg/g) = the amount of fluo-NP adsorbed onto coffee grounds at equilibrium. The data represent the mean ± standard deviation of four replicates from two different sources of coffee grounds. Reproduced with permission from Elsevier from a study by Yen et al. (Citation2022). Removal of nano-sized polystyrene plastic from aqueous solutions using untreated coffee grounds. Chemosphere, 286, 131863. https://doi.org/10.1016/j.chemosphere.2021.131863. (b) Effect of different initial concentrations on the adsorption of PS microplastics on 3D RGO. Reproduced with permission from IWA publishing from a study by Yuan et al. (Citation2020). Study on the adsorption of polystyrene microplastics by three-dimensional reduced graphene oxide. Water Science and Technology, 81(10), 2163–2175. https://doi.org/10.2166/wst.2020.269.
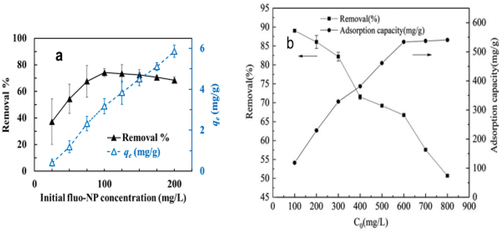
The adsorption process, particularly when employing adsorbents derived from biomass, exhibits a significant dependence on the initial concentration of MPs. It has been noted that as the initial MPs concentration increases, there is a noticeable reduction in adsorption capacity. These findings emphasise the critical need to carefully consider and optimise the initial MPs concentration in environmental remediation strategies.
4.5. Effect of pH and zeta potential
Understanding how the adsorption capacity of the material is impacted by solution pH and zeta potential is crucial for assessing their suitability in environmental applications and gaining valuable mechanistic insights. The primary way pH impacts adsorption efficiency is by altering the surface charge of both microplastics and the adsorbent material (Wang et al. Citation2021b). Tran et al, conducted a study investigating how variations in pH levels influence the removal of microplastics. They found that at a pH of 6, sponges exhibited positively charged surfaces, while polystyrene microplastics displayed negatively charged surfaces. This resulted in a strong electrostatic attraction between them, which was more pronounced compared to when pH levels differed (Tran et al. Citation2019). Additionally, in his research team, Ganie observed that optimal interaction between the bioadsorbent and microplastics was achieved due to the lower magnitude of negative zeta potential and reduced quantity of negatively charged functional groups (Ganie et al. Citation2021). According to (Yuan et al. Citation2020) at pH 2, positive charges on three-dimensional reduced graphene oxide (3D RGO) repelled positively charged MPs, limiting their removal and reducing 3D RGO’s adsorption capacity. Conversely, at pH 4, slight negative charges on MPs attracted them to positively charged 3D RGO, driven by electrostatic forces. At pH 6, increased negative charges on MPs maximised electrostatic attraction, achieving the highest MPs removal efficiency as shown in .
Figure 11. (a) Effect of different initial pH values on the adsorption of microplastics on 3D RGO. (b) Zeta potentials of 3D RGO and PS microplastics in different pH values. Reproduced with permission from IWA publishing from a study by Yuan et al. (Citation2020). Study on the adsorption of polystyrene microplastics by three-dimensional reduced graphene oxide. Water Science and Technology, 81(10), 2163–2175. https://doi.org/10.2166/wst.2020.269..
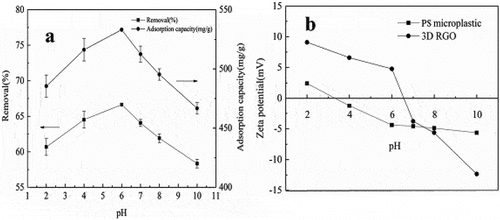
Moreover, an analysis of the surface charge alterations of activated carbon in ultrapure water across a range of pH values revealed that the activated carbon predominantly exhibited a negative surface charge (Arenas et al. Citation2021). This negative charge facilitated the electrostatic attraction of positively charged MP particles. In a study where magnetic biochar was derived from pine wood at 500°C for MP removal, it was found that the highest efficiency of MP removal by magnetic biochar occurred in solutions with a weakly acidic or neutral pH (Shi et al. Citation2023a). Additionally, Shi and their research team examined the elimination of MPs by employing a composite derived from magnetic biochar that has been modified with cetyltrimethylammonium bromide. At a pH of 11, the removal rates dropped to 67.4% for polystyrene and 40.7% for carboxylate-modified polystyrene (Shi et al. Citation2023b). In summary, the investigation into the impact of pH and zeta potential on adsorption of MPs reveals that the surface charge of the adsorbent varies depending on the type of biomass material used. Furthermore, there exists a notable correlation between the pH and zeta potential of the adsorbent and its surface chemistry. The literature reviewed highlights varying pH levels associated with different rates of MPs adsorption underscoring the intricate interplay of these variables in shaping the adsorption characteristics of MPs in aquatic environment. Moreover, recent studies have predominately focused on the removal of polystyrene MPs. Therefore, it is essential to establish pH-related surface charges when assessing the elimination of MPs from other polymer types. Consequently, there is an imperative need for further research to optimise these parameters for MPs removal.
5. The role of isotherm modeling
Isotherm modelling is a critical tool for understanding how microplastics (MPs) interact with biomass-derived adsorbents. It helps us optimise the adsorption process by providing insights into the interaction between the adsorbent surfaces and MP contaminants. This section delves into the profound significance of isotherm modelling within the context of MPs adsorption employing biomass-derived adsorbents. A study on magnetic biochar (MBC) for MP removal found that the process followed a pseudo-second-order kinetic model, indicating a time-dependent interaction and reliance on available binding sites. Additionally, the adsorption capacity increased with higher MP dosage, and the Langmuir isotherm model best fit the data (R2 >0.897), suggesting a monolayer adsorption process (J. Wang et al. Citation2021a). Similar conclusions were drawn in another study utilising sugarcane bagasse-derived biochar (BC) at varying temperatures for nanoplastics (NPs) removal. Results indicated that BC-750 adhered to the Langmuir isotherm model, suggesting a monolayer nature of sorption, indicative of homogenised sorption sites for NPs (Ganie et al. Citation2021). In a separate investigation involving the functionalization of natural loofah sponge with bee wax and palm wax for PS microplastics removal, both Langmuir and Freundlich isotherm models depicted excellent data fitting. However, a marginally higher fitting correlation coefficient for the Langmuir model suggested a dominant monolayer adsorption mechanism (Ha et al. Citation2023). Lastly, in the development of green sponge material utilising chemically cross-linked plant proteins for MPs adsorption, Langmuir modelling showcased superior representativeness over Freundlich modelling, affirming a monolayer physical adsorption mechanism for MPs onto Oat protein sponges (Z. Wang et al. Citation2021b). These studies highlight the importance of isotherm modelling in optimising the use of biomass-derived adsorbents for microplastic removal. The specific model that best fits the data helps us understand the underlying mechanisms and improve the efficiency of this approach.
6. Regeneration and reusability of bioadsorbents
Regenerating bioadsorbents is crucial for their practical application across various industries. There exists a robust demand for bioadsorbents with exceptional reusability in industrial contexts, driven by the significant economic benefits they offer (Chong, Soh, and Yong Citation2023). Maintaining a high removal efficiency over multiple adsorption-desorption cycles is vital, necessitating minimal loss of active sites in bioadsorbents (Tee, Gok, and Yong Citation2022). Therefore, careful selection of eluents for the desorption process is essential to develop cost-effective bioadsorbents with high reusability for industrial wastewater treatment. These eluents should be eco-friendly, efficient, and inexpensive, ensuring they do not damage the surface of bioadsorbents (Chong, Soh, and Yong Citation2023). The most commonly used eluents for reusability experiments are alcohols, acids, and alkalis. Cellulose nanofibers (CNF)/3-glycidyloxypropyltrimethoxysilane (GPTMS)/polyethyleneimine (PEI) aerogel was used for removal of polystyrene microspheres (1 μm). The researchers reported that the aerogels can maintain a high removal efficiency of polystyrene microspheres for at least five adsorption-desorption cycles (Zhuang et al. Citation2023). On the other hand, regeneration of Mg/Zn modified magnetic biochar adsorbents for microplastic removal was studied to assess the recovery performance, through the adsorption experiment by applying the same condition of the crude magnetic biochar (MBC) (10 mg adsorbents: 100 mL microplastic solutions). The removal efficiencies of microplastics by regeneration of three magnetic biochars show no significant decrease, which are all higher than 94%, after 5 cycles (Wang et al. Citation2021a). In another study were an efficient and reusable composite was prepared from cetyltrimethylammonium bromide (CTAB) modified magnetic biochar (CMB) for the removal of polystyrene (PS) and carboxylate-modified polystyrene (CPS) nanoparticles from water, removal efficiencies of PS and CPS were 90.2% for PS and 94.8% for CPS when CMB was recycled five times (Shi et al. Citation2023b).
7. Conclusion and future prospects
Research on the removal of MPs from aquatic ecosystems is in its early stages, and there are no targeted MPs removal processes currently in use in real-world aquatic systems. Water treatment facilities, while crucial in mitigating the release of MPs into the environment, currently lack the capability to efficiently eliminate small-sized MPs particles. This results in the continuous discharge of millions of MPs into aquatic ecosystems. Research on the utilisation of chemically modified biochars, activated carbons, sponges, and graphene materials for MPs removal is still insufficient. These materials can be chemically altered to increase their surface area, hydrophobicity, and affinity for MPs, making them more effective at removing MPs from aquatic ecosystems. Techniques such as methylation of amino groups, amination of hydroxyl groups, and esterification of carboxyl groups hold promising potential. The zeta potential, pH, and surface chemistry have been identified as critical factors in the removal of microplastics. This is because they can alter the surface charge and functional groups of the adsorbents, thus enhancing the mechanisms for removing MPs. However, there is a need for further research to comprehensively understand and optimise the interplay of these factors to develop more effective microplastic removal strategies.
The review of the literature has shown that the efficiency of microplastics removal varies among bioadsorbents derived from different biomass sources. Investigating how bioadsorbent characteristics, such as biomass type, preparation methods, and conditions, influence the removal of MPs from aquatic ecosystems is crucial and warrants additional research. Additionally, the concentration of adsorbents plays a significant role in MPs removal. Increasing the adsorbent concentration can significantly enhance the removal of MPs, but excessive amounts can lead to clumping together, reducing the number of accessible adsorption sites. Therefore, determining the optimal quantity of adsorbent is paramount, not only for optimising its efficiency but also for ensuring cost-effectiveness.
Insights into the interaction between MPs and biomass-derived adsorbents have revealed that efficient removal mechanisms depend on various factors, including surface properties, adsorbent structure, the affinity of biomass-derived materials to microplastics, and the inherent characteristics of both the adsorbent and microplastics. However, there is still much to explore in terms of optimising these materials and elucidating their complex interactions with microplastics.While much research has focused on the removal of polystyrene MPs, it is essential to investigate the effectiveness of bioadsorbents in removing other types of MPs polymers. A deeper understanding of the mechanisms governing MPs’ adsorption when using bioadsorbents in aquatic environments is still in its early stages, with limited large-scale practical applications. This study contributes significantly to future research on microplastics (MPs) removal from aquatic ecosystems. It highlights the early stage of research in this field and the lack of targeted removal processes in real-world settings. By identifying materials such as chemically modified biochars and activated carbons as promising options for MPs removal, the study emphasises the need for further investigation into biomass characteristics and preparation methods. It also underscores the importance of factors like surface chemistry and adsorbent concentration in optimising removal efficiency. Additionally, the study calls for further research to understand the complex interactions between MPs and biomass-derived adsorbents, paving the way for the development of effective and scalable removal strategies in aquatic environments.
Highlights
Emerging biomass-based adsorbents for the removal of microplastics from aquatic ecosystems
Surface modification/treatment methods discussed
Mechanisms of interaction between microplastics and bioadsorbents
Factors affecting microplastics removal efficiency from aquatic systems
Future prospects of biomass-based adsorbents for microplastics removal from aquatic systems
Authors contribution
The contribution of the authors is as follows:
Mulindwa Patrick: Investigation, conceptualisation, Methodology, validation, formal analysis and writing original draft.
Antonio Jose Expósito: Validation, critical review & editing.
Job S. Kasule: Investigation, conceptualisation and design, validation, critical review & editing.
Florence Nantaba: Validation, critical review & editing.
John Wasswa: Funding sourcing, validation, project management, critical review & editing.
All the authors approved of the final version and are accountable for all aspects of the work.
Disclosure statement
No potential conflict of interest was reported by the author(s).
Data availability statement
Data sharing is not applicable to this article as no new data were created or analysed in this study.
Additional information
Funding
Notes on contributors
Patrick Mulindwa
Mr. Patrick Mulindwa is a PhD candidate and assistant lecturer in the Department of Chemistry at Makerere University’s School of Physical Sciences. With a background in Industrial Chemistry and a Master of Science specializing in Food Processing and Storage, he is deeply involved in applied research. His expertise includes postharvest technology, bioenergy, value addition, and wastewater treatment. Currently, his doctoral research focuses on removing microplastics from wastewater, reflecting his dedication to tackling environmental issues. Patrick’s diverse interests also encompass biofuel production, water quality, food safety, and postharvest technology.
Job S. Kasule
Dr. Job. S. Kasule is a lecturer in the Department of Chemistry of Makerere University. He holds a Ph.D. in Chemical Engineering from West Virginia University, Morgantown, WV in the USA, a Master of Science in Chemical Engineering from Clarkson University, NY and a Bachelors degree in Industrial Chemistry from Makerere University, Kampala Uganda. His research interests are in the areas of Mathematical modeling, design, and simulation of process systems, CO2 capture and utilization processes, alternative and sustainable energy sources, Environmental pollution prevention, material sciences for energy storage among other areas.
Florence Nantaba
Dr. Florence Nantaba is a lecturer in the Department of Chemistry of Makerere University. She holds a Ph.D. in Chemistry, Master of Science in Chemistry and Bachelor of Science in Chemistry of Makerere University. She is one of the few female faculty in the Department of Chemistry and a role model to many female students aspiring to pursuing a career in science. Her research interests are in the study of source and fate of persistent organic pollutants, emerging pollutants, pharmaceutical wastes fate and removal from water bodies, environmental chemistry, analytical chemistry and their application in sustainable manufacturing. Dr. Nantaba also has research interests in the oil and gas processes pollution prevention and drug formulation studies.
John Wasswa
Dr. John Wasswa is a senior faculty in the Department of Chemistry of Makerere University, Kampala. He holds a Ph.D. in Chemistry, Master of Science in Chemistry and Bachelor of Science in Chemistry of Makerere University. He has been the Chair of the Department of Chemistry for the last eight years where he has showcased distinguished service to the Department and the University at large. His research is in the areas of Environmental chemistry, Analytical chemistry, behavior and removal of persistent organic pollutants, emerging pollutants from the environmental ecosystems, remediation of natural wetlands, water quality improvement, air quality research, oil and gas pollution.
Antonio Jose Expósito
Dr. Antonio Jose Expósito is a distinguished researcher and educator at the University of Bath, UK, specializing in catalyst design and reactor engineering. He holds a Doctor of Philosophy in Chemical and Environmental Engineering from the Universidad de Castilla-La Mancha (2014-2017), reflecting his solid academic foundation. Dr. Expósito’s research interests are broad and impactful, encompassing the development of innovative catalysts for applications in energy production, wastewater treatment, microplastic removal, and fine chemical synthesis.
References
- Adeleye, A. T., M. M. Bahar, M. Megharaj, and M. M. Rahman. 2023. “Recent Developments and Mechanistic Insights on Adsorption Technology for Micro-And Nanoplastics Removal in Aquatic Environments.” Journal of Water Process Engineering 53:103777. https://doi.org/10.1016/j.jwpe.2023.103777.
- Ahmad, M., N. M. A. Lubis, M. Usama, J. Ahmad, M. I. Al-Wabel, H. A. Al-Swadi, M. I. Rafique, and A. S. F. Al-Farraj. 2023. “Scavenging Microplastics and Heavy Metals from Water Using Jujube Waste-Derived Biochar in Fixed-Bed Column Trials.” Environmental Pollution 335:122319. https://doi.org/10.1016/j.envpol.2023.122319.
- Alghamdi, A. A., A.-B. Al-Odayni, W. S. Saeed, A. Al-Kahtani, F. A. Alharthi, and T. Aouak. 2019. “Efficient Adsorption of Lead (II) from Aqueous Phase Solutions Using Polypyrrole-Based Activated Carbon.” Materials 12 (12): 2020. https://doi.org/10.3390/ma121220202020.
- Alharbi, N. S., B. Hu, T. Hayat, S. O. Rabah, A. Alsaedi, L. Zhuang, and X. Wang. 2020. “Efficient Elimination of Environmental Pollutants Through Sorption-Reduction and Photocatalytic Degradation Using Nanomaterials.” Frontiers of Chemical Science and Engineering 14 (6): 1124–1135. https://doi.org/10.1007/s11705-020-1923-z.
- Arenas, L. R., S. R. Gentile, S. Zimmermann, and S. Stoll. 2021. “Nanoplastics Adsorption and Removal Efficiency by Granular Activated Carbon Used in Drinking Water Treatment Process.” Science of the Total Environment 791:148175. https://doi.org/10.1016/j.scitotenv.2021.148175.
- Bayo, J., S. Olmos, and J. López-Castellanos. 2020. “Microplastics in an Urban Wastewater Treatment Plant: The Influence of Physicochemical Parameters and Environmental Factors.” Chemosphere 238:124593. https://doi.org/10.1016/j.chemosphere.2019.124593.
- Chen, T., L. Luo, S. Deng, G. Shi, S. Zhang, Y. Zhang, O. Deng, L. Wang, J. Zhang, and L. Wei. 2018. “Sorption of Tetracycline on H3PO4 Modified Biochar Derived from Rice Straw and Swine Manure.” Bioresource Technology 267:431–437. https://doi.org/10.1016/j.biortech.2018.07.074.
- Cheng, N., B. Wang, P. Wu, X. Lee, Y. Xing, M. Chen, and B. Gao. 2021. “Adsorption of Emerging Contaminants from Water and Wastewater by Modified Biochar: A Review.” Environmental Pollution (Barking, Essex: 1987) 273:116448. https://doi.org/10.1016/j.envpol.2021.116448.
- Chong, Z. T., L. S. Soh, and W. F. Yong. 2023. “Valorization of Agriculture Wastes as Biosorbents for Adsorption of Emerging Pollutants: Modification, Remediation and Industry Application.” Results in Engineering 17:100960. https://doi.org/10.1016/j.rineng.2022.100786.
- Dai, Y., M. Liu, J. Li, S. Yang, Y. Sun, Q. Sun, W. Wang, L. Lu, K. Zhang, and J. Xu. 2020. “A Review on Pollution Situation and Treatment Methods of Tetracycline in Groundwater.” Separation Science and Technology 55 (5): 1005–1021. https://doi.org/10.1080/01496395.2019.1577445.
- Dai, Y., W. Wang, L. Lu, L. Yan, and D. Yu. 2020. “Utilization of Biochar for the Removal of Nitrogen and Phosphorus.” Journal of Cleaner Production 257:120573. https://doi.org/10.1016/j.jclepro.2020.120573.
- Dey, T. K., M. Jamal, and E. Uddin. 2023. “Fabrication and Performance Analysis of Graphene Oxide-Based Composite Membrane to Separate Microplastics from Synthetic Wastewater.” Journal of Water Process Engineering 52:103554. https://doi.org/10.1016/j.jwpe.2023.103554.
- Dong, M., L. He, M. Jiang, Y. Zhu, J. Wang, W. Gustave, S. Wang, Y. Deng, X. Zhang, and Z. Wang. 2023. “Biochar for the Removal of Emerging Pollutants from Aquatic Systems: A Review.” International Journal of Environmental Research and Public Health 20 (3): 1679. https://doi.org/10.3390/ijerph20031679.
- Egessa, R., A. Nankabirwa, H. Ocaya, and W. G. Pabire. 2020. “Microplastic Pollution in Surface Water of Lake Victoria.” Science of the Total Environment 741:140201. https://doi.org/10.1016/j.scitotenv.2020.140201.
- El Naga, A. O. A., M. El Saied, S. A. Shaban, and F. Y. El Kady. 2019. “Fast Removal of Diclofenac Sodium from Aqueous Solution Using Sugar Cane Bagasse-Derived Activated Carbon.” Journal of Molecular Liquids 285:9–19. https://doi.org/10.1016/j.molliq.2019.04.062.
- Föhr, J., T. Ranta, J. Suikki, and H. Soininen. 2017. “Manufacturing of Torrefied Pellets without a Binder from Different Raw Wood Materials in the Pilot Plant.” Wood Res 62 (3): 481–494.
- Fu, J., N. Liu, Y. Peng, G. Wang, X. Wang, Q. Wang, M. Lv, and L. Chen. 2023. “An Ultra-Light Sustainable Sponge for Elimination of Microplastics and Nanoplastics.” Journal of Hazardous Materials 456:131685. https://doi.org/10.1016/j.jhazmat.2023.131685.
- Galvão, R. B., A. A. da Silva Moretti, F. Fernandes, and E. K. Kuroda. 2021. “Post-Treatment of Stabilized Landfill Leachate by Upflow Gravel Filtration and Granular Activated Carbon Adsorption.” Environmental Technology 42 (26): 4179–4188. https://doi.org/10.1080/09593330.2020.1746838.
- Ganie, Z. A., N. Khandelwal, E. Tiwari, N. Singh, and G. K. Darbha. 2021. “Biochar-Facilitated Remediation of Nanoplastic Contaminated Water: Effect of Pyrolysis Temperature Induced Surface Modifications.” Journal of Hazardous Materials 417:126096. https://doi.org/10.1016/j.jhazmat.2021.126096.
- Goh, P., H. Kang, A. Ismail, W. Khor, L. Quen, and D. Higgins. 2022. “Nanomaterials for Microplastic Remediation from Aquatic Environment: Why Nano Matters?” Chemosphere 299:134418. https://doi.org/10.1016/j.chemosphere.2022.134418.
- Grbic, J., B. Nguyen, E. Guo, J. B. You, D. Sinton, and C. M. Rochman. 2019. “Magnetic Extraction of Microplastics from Environmental Samples.” Environmental Science & Technology Letters 6 (2): 68–72. https://doi.org/10.1021/acs.estlett.8b00671.
- Guo, L., L. Zhao, Y. Tang, J. Zhou, and B. Shi. 2022. “Switching the Free Radical Based Peroxydisulfate Activation to the Nonradical Pathway by a Chrome Shaving–Derived Biochar for the Efficient Degradation of Tetracycline.” Chemical Engineering Journal 435:135189. https://doi.org/10.1016/j.cej.2022.135189.
- Ha, T. T. V., N. M. Viet, P. T. Thanh, and V. T. Quan. 2023. “Loofah Plant—Derived Biodegradable Superhydrophobic Sponge for Effective Removal of Oil and Microplastic from Water.” Environmental Technology & Innovation 32:103265. https://doi.org/10.1016/j.eti.2023.103265.
- Hanif, M. A., N. Ibrahim, F. A. Dahalan, U. F. Ali, M. Hasan, A. W. Azhari, and A. A. Jalil. 2023. “Microplastics in Facial Cleanser: Extraction, Identification, Potential Toxicity, and Continuous-Flow Removal Using Agricultural Waste–Based Biochar.” Environmental Science and Pollution Research 30 (21): 60106–60120. https://doi.org/10.1007/s11356-023-26741-8.
- Hao, M., M. Qiu, H. Yang, B. Hu, and X. Wang. 2021. “Recent Advances on Preparation and Environmental Applications of MOF-Derived Carbons in Catalysis.” Science of the Total Environment 760:143333. https://doi.org/10.1016/j.scitotenv.2020.143333.
- Hsieh, L., L. He, M. Zhang, W. Lv, K. Yang, and M. Tong. 2022. “Addition of Biochar as Thin Preamble Layer into Sand Filtration Columns Could Improve the Microplastics Removal from Water.” Water Research 221:118783. https://doi.org/10.1016/j.watres.2022.118783.
- Huang, M.-L., P.-L. Yen, and V. H.-C. Liao. 2021. “A Combined Approach to Remediate Cadmium Contaminated Sediment Using the Acidophilic Sulfur-Oxidizing Bacterial SV5 and Untreated Coffee Ground.” Chemosphere 273:129662. https://doi.org/10.1016/j.chemosphere.2021.129662.
- Jjagwe, J., P. W. Olupot, E. Menya, and H. M. Kalibbala. 2021. “Synthesis and Application of Granular Activated Carbon from Biomass Waste Materials for Water Treatment: A Review.” Journal of Bioresources and Bioproducts 6 (4): 292–322. https://doi.org/10.1016/j.jobab.2021.03.003.
- Kafi, M. A., K. Aktar, M. Todo, and R. Dahiya. 2020. “Engineered Chitosan for Improved 3D Tissue Growth Through Paxillin-FAK-ERK Activation.” Regenerative Biomaterials 7 (2): 141–151. https://doi.org/10.1093/rb/rbz034.
- Kafi, M. A., A. Paul, A. Vilouras, and R. Dahiya. 2020. “Mesoporous Chitosan Based Conformable and Resorbable Biostrip for Dopamine Detection.” Biosensors and Bioelectronics 147:111781. https://doi.org/10.1016/j.bios.2019.111781.
- Karri, R. R., J. Sahu, and B. Meikap. 2020. “Improving Efficacy of Cr (VI) Adsorption Process on Sustainable Adsorbent Derived from Waste Biomass (Sugarcane Bagasse) with Help of Ant Colony Optimization.” Industrial Crops and Products 143:111927. https://doi.org/10.1016/j.indcrop.2019.111927.
- Kaur, P., P. Kaur, and K. Kaur. 2020. “Adsorptive Removal of Imazethapyr and Imazamox from Aqueous Solution Using Modified Rice Husk.” Journal of Cleaner Production 244:118699. https://doi.org/10.1016/j.jclepro.2019.118699.
- Kausar, A., K. Naeem, T. Hussain, H. N. Bhatti, F. Jubeen, A. Nazir, and M. Iqbal. 2019. “Preparation and Characterization of Chitosan/Clay Composite for Direct Rose FRN Dye Removal from Aqueous Media: Comparison of Linear and Non-Linear Regression Methods.” Journal of Materials Research and Technology 8 (1): 1161–1174. https://doi.org/10.1016/j.jmrt.2018.07.020.
- Kim, K. T., and S. Park. 2021. “Enhancing Microplastics Removal from Wastewater Using Electro-Coagulation and Granule-Activated Carbon with Thermal Regeneration.” Processes 9 (4): 617. https://doi.org/10.3390/pr9040617.
- Kumar, R., A. Verma, R. J. Rakib, P. K. Gupta, P. Sharma, A. Garg, P. Girard, and T. M. Aminabhavi. 2023. “Adsorptive Behavior of Micro(nano)plastics Through Biochar: Co-Existence, Consequences, and Challenges in Contaminated Ecosystems.” Science of the Total Environment 856:159097. https://doi.org/10.1016/j.scitotenv.2022.159097.
- Li, J., X. Chen, S. Yu, and M. Cui. 2023. “Removal of Pristine and Aged Microplastics from Water by Magnetic Biochar: Adsorption and Magnetization.” Science of the Total Environment 875:162647. https://doi.org/10.1016/j.scitotenv.2023.162647.
- Liang, C., G. Gascó, S. Fu, A. Méndez, and J. Paz-Ferreiro. 2016. “Biochar from Pruning Residues as a Soil Amendment: Effects of Pyrolysis Temperature and Particle Size.” Soil and Tillage Research 164:3–10. https://doi.org/10.1016/j.still.2015.10.002.
- Lima, L., B. E. Baêta, D. R. Lima, R. J. Afonso, S. F. De Aquino, and M. Libânio. 2016. “Comparison Between Two Forms of Granular Activated Carbon for the Removal of Pharmaceuticals from Different Waters.” Environmental Technology 37 (11): 1334–1345. https://doi.org/10.1080/09593330.2015.1114030.
- Liu, D., Q. Xie, X. Huang, C. Wan, F. Deng, D. Liang, and J. Liu. 2020. “Backwashing Behavior and Hydrodynamic Performances of Granular Activated Carbon Blends.” Environmental Research 184:109302. https://doi.org/10.1016/j.envres.2020.109302.
- Liu, J., X. Che, X. Huang, Y. Mo, Y. Wen, J. Jia, H. Zhou, and B. Yan. 2022. “The Interaction Between Biochars from Distinct Pyrolysis Temperatures and Multiple Pollutants Determines Their Combined Cytotoxicity.” Chemosphere 296:133999. https://doi.org/10.1016/j.chemosphere.2022.133999.
- Lonappan, L., T. Rouissi, S. K. Brar, M. Verma, and R. Y. Surampalli. 2018. “Adsorption of Diclofenac Onto Different Biochar Microparticles: Dataset – Characterization and Dosage of Biochar.” Data in Brief 16:460–465. https://doi.org/10.1016/j.dib.2017.10.041.
- Lu, Z., W. Sun, C. Li, W. Cao, Z. Jing, S. Li, X. Ao, C. Chen, and S. Liu. 2020. “Effect of Granular Activated Carbon Pore-Size Distribution on Biological Activated Carbon Filter Performance.” Water Research 177:115768. https://doi.org/10.1016/j.watres.2020.115768.
- Lv, X., Q. Dong, Z. Zuo, Y. Liu, X. Huang, and W.-M. Wu. 2019. “Microplastics in a Municipal Wastewater Treatment Plant: Fate, Dynamic Distribution, Removal Efficiencies, and Control Strategies.” Journal of Cleaner Production 225:579–586. https://doi.org/10.1016/j.jclepro.2019.03.321.
- Lyu, H., S. Xia, J. Tang, Y. Zhang, B. Gao, and B. Shen. 2020. “Thiol-Modified Biochar Synthesized by a Facile Ball-Milling Method for Enhanced Sorption of Inorganic Hg2+ and Organic CH3Hg+.” Journal of Hazardous Materials 384:121357. https://doi.org/10.1016/j.jhazmat.2019.121357.
- Magid, A. S. I. A., M. S. Islam, Y. Chen, L. Weng, J. Li, J. Ma, and Y. Li. 2021. “Enhanced Adsorption of Polystyrene Nanoplastics (PSNPs) Onto Oxidized Corncob Biochar with High Pyrolysis Temperature.” Science of the Total Environment 784:147115. https://doi.org/10.1016/j.scitotenv.2021.147115.
- Maliwan, T., W. Pungrasmi, and J. Lohwacharin. 2021. “Effects of Microplastic Accumulation on Floc Characteristics and Fouling Behavior in a Membrane Bioreactor.” Journal of Hazardous Materials 411:124991. https://doi.org/10.1016/j.jhazmat.2020.124991.
- Meilani, V., J.-I. Lee, J.-K. Kang, C.-G. Lee, S. Jeong, and S.-J. Park. 2021. “Application of Aluminum-Modified Food Waste Biochar as Adsorbent of Fluoride in Aqueous Solutions and Optimization of Production Using Response Surface Methodology.” Microporous and Mesoporous Materials 312:110764. https://doi.org/10.1016/j.micromeso.2020.110764.
- Mohammaad, A., and M. Kijevcanin. 2023. “Synthesis of Activated Carbons from Water Hyacinth Biomass and its Application as Adsorbents in Water Pollution Control.” Journal of the Serbian Chemical Society 88 (1): 69–82. https://doi.org/10.2298/JSC212121006M.
- Nantaba, F., W.-U. Palm, J. Wasswa, H. Bouwman, H. Kylin, and K. Kuemmerer. 2021. “Temporal Dynamics and Ecotoxicological Risk Assessment of Personal Care Products, Phthalate Ester Plasticizers, and Organophosphorus Flame Retardants in Water from Lake Victoria, Uganda.” Chemosphere 262:127716. https://doi.org/10.1016/j.chemosphere.2020.127716.
- Ngeno, E. C., K. E. Mbuci, M. C. Necibi, V. O. Shikuku, C. Olisah, R. Ongulu, H. Matovu, P. Ssebugere, A. Abushaban, and M. Sillanpää. 2022. “Sustainable Re-Utilization of Waste Materials as Adsorbents for Water and Wastewater Treatment in Africa: Recent Studies, Research Gaps, and Way Forward for Emerging Economies.” Environmental Advances 9:100282. https://doi.org/10.1016/j.envadv.2022.100282.
- Nguyen, P.-D., Q.-V. Tran, T.-T. Le, Q.-H. Nguyen, T.-C. Kieu-Le, and E. Strady. 2023. “Evaluation of Microplastic Removal Efficiency of Wastewater-Treatment Plants in a Developing Country, Vietnam.” Environmental Technology & Innovation 29:102994. https://doi.org/10.1016/j.eti.2022.102994.
- Olugbenga, O. S., P. G. Adeleye, S. B. Oladipupo, A. T. Adeleye, and K. I. John. 2023. “Biomass-Derived Biochar in Wastewater Treatment-A Circular Economy Approach.” Waste Management Bulletin 1 (4): 1–14. https://doi.org/10.1016/j.wmb.2023.07.007.
- Pan, Y., S.-H. Gao, C. Ge, Q. Gao, S. Huang, Y. Kang, G. Luo, et al. 2023. “Removing Microplastics from Aquatic Environments: A Critical Review.” Environmental Science and Ecotechnology 13:100222. https://doi.org/10.1016/j.ese.2022.100222.
- Paunovic, O., S. Pap, S. Maletic, M. A. Taggart, N. Boskovic, and M. T. Sekulic. 2019. “Ionisable Emerging Pharmaceutical Adsorption Onto Microwave Functionalised Biochar Derived from Novel Lignocellulosic Waste Biomass.” Journal of Colloid and Interface Science 547:350–360. https://doi.org/10.1016/j.jcis.2019.04.011.
- Peng, G., M. Xiang, W. Wang, Z. Su, H. Liu, Y. Mao, Y. Chen, and P. Zhang. 2022. “Engineering 3D Graphene-Like Carbon-Assembled Layered Double Oxide for Efficient Microplastic Removal in a Wide pH Range.” Journal of Hazardous Materials 433:128672. https://doi.org/10.1016/j.jhazmat.2022.128672.
- Pivokonský, M., L. Pivokonská, K. Novotná, L. Čermáková, and M. Klimtová. 2020. “Occurrence and Fate of Microplastics at Two Different Drinking Water Treatment Plants within a River Catchment.” Science of the Total Environment 741:140236. https://doi.org/10.1016/j.scitotenv.2020.140236.
- Qiu, B., Q. Shao, J. Shi, C. Yang, and H. Chu. 2022. “Application of Biochar for the Adsorption of Organic Pollutants from Wastewater: Modification Strategies, Mechanisms and Challenges.” Separation and Purification Technology 300:121925. https://doi.org/10.1016/j.seppur.2022.121925.
- Qu, J., Y. Liu, L. Cheng, Z. Jiang, G. Zhang, F. Deng, L. Wang, W. Han, and Y. Zhang. 2021. “Green Synthesis of Hydrophilic Activated Carbon Supported Sulfide nZVI for Enhanced Pb(ii) Scavenging from Water: Characterization, Kinetics, Isotherms and Mechanisms.” Journal of Hazardous Materials 403:123607. https://doi.org/10.1016/j.jhazmat.2020.123607.
- Risch, P., and C. Adlhart. 2021. “A Chitosan Nanofiber Sponge for Oyster-Inspired Filtration of Microplastics.” ACS Applied Polymer Materials 3 (9): 4685–4694. https://doi.org/10.1021/acsapm.1c00799.
- Rius-Ayra, O., A. Biserova-Tahchieva, V. Sansa-López, and N. Llorca-Isern. 2022. “Superhydrophobic PDMS Coated 304 Stainless-Steel Mesh for the Removal of HDPE Microplastics.” Progress in Organic Coatings 170:107009. https://doi.org/10.1016/j.porgcoat.2022.107009.
- Rong, X., X. Chen, P. Li, C. Zhao, S. Peng, H. Ma, and H. Qu. 2022. “Mechanically Durable Anti-Bacteria Non-Fluorinated Superhydrophobic Sponge for Highly Efficient and Fast Microplastic and Oil Removal.” Chemosphere 299:134493. https://doi.org/10.1016/j.chemosphere.2022.134493.
- Rose, P. K., M. Jain, N. Kataria, P. K. Sahoo, V. K. Garg, and A. Yadav. 2023. “Microplastics in Multimedia Environment: A Systematic Review on its Fate, Transport, Quantification, Health Risk, and Remedial Measures.” Groundwater for Sustainable Development 20:100889. https://doi.org/10.1016/j.gsd.2022.100889.
- Santoso, E., R. Ediati, Y. Kusumawati, H. Bahruji, D. Sulistiono, and D. Prasetyoko. 2020. “Review on Recent Advances of Carbon Based Adsorbent for Methylene Blue Removal from Waste Water.” Materials Today Chemistry 16:100233. https://doi.org/10.1016/j.mtchem.2019.100233.
- Senathirajah, K., and T. Palanisami. 2023. “Strategies to Reduce Risk and Mitigate Impacts of Disaster: Increasing Water Quality Resilience from Microplastics in the Water Supply System.” ACS ES&T Water 3 (9): 2816–2834. https://doi.org/10.1021/acsestwater.3c00206.
- Sheng, G., C. Huang, G. Chen, J. Sheng, X. Ren, B. Hu, J. Ma, et al. 2018. “Adsorption and Co-Adsorption of Graphene Oxide and Ni(ii) on Iron Oxides: A Spectroscopic and Microscopic Investigation.” Environmental Pollution 233:125–131. https://doi.org/10.1016/j.envpol.2017.10.047.
- Sher, F., K. Hanif, A. Rafey, U. Khalid, A. Zafar, M. Ameen, and E. C. Lima. 2021. “Removal of Micropollutants from Municipal Wastewater Using Different Types of Activated Carbons.” Journal of Environmental Management 278 (Pt 2): 111302. https://doi.org/10.1016/j.jenvman.2020.111302.
- Shi, Q., S. Guo, J. Tang, H. Lyu, C. Ri, and H. Sun. 2023a. “Enhanced Removal of Aged and Differently Functionalized Polystyrene Nanoplastics Using Ball-Milled Magnetic Pinewood Biochars.” Environmental Pollution 316:120696. https://doi.org/10.1016/j.envpol.2022.120696.
- Shi, Y., J. Du, T. Zhao, B. Feng, H. Bian, S. Shan, J. Meng, P. Christie, M. H. Wong, and J. Zhang. 2023b. “Removal of Nanoplastics from Aqueous Solution by Aggregation Using Reusable Magnetic Biochar Modified with Cetyltrimethylammonium Bromide.” Environmental Pollution 318:120897. https://doi.org/10.1016/j.envpol.2022.120897.
- Siipola, V., H. Romar, and U. Lassi. 2022. “Microplastic Removal from Water and Wastewater by Carbon-Supported Materials.” In Sustainable Biochar for Water and Wastewater Treatment, edited by D. Mohan, C. Pittman Jr., and T. E. Mlsna, 361–393. Elsevier.
- Singh, N., N. Khandelwal, Z. A. Ganie, E. Tiwari, and G. K. Darbha. 2021. “Eco-Friendly Magnetic Biochar: An Effective Trap for Nanoplastics of Varying Surface Functionality and Size in the Aqueous Environment.” Chemical Engineering Journal 418:129405. https://doi.org/10.1016/j.cej.2021.129405.
- Soffian, M. S., F. Z. A. Halim, F. Aziz, M. A. Rahman, M. A. M. Amin, and D. N. A. Chee. 2022. “Carbon-Based Material Derived from Biomass Waste for Wastewater Treatment.” Environmental Advances 9:100259. https://doi.org/10.1016/j.envadv.2022.100259.
- Sun, C., Z. Wang, L. Chen, and F. Li. 2020. “Fabrication of Robust and Compressive Chitin and Graphene Oxide Sponges for Removal of Microplastics with Different Functional Groups.” Chemical Engineering Journal 393:124796. https://doi.org/10.1016/j.cej.2020.124796.
- Sun, C., Z. Wang, H. Zheng, L. Chen, and F. Li. 2021. “Biodegradable and Re-Usable Sponge Materials Made from Chitin for Efficient Removal of Microplastics.” Journal of Hazardous Materials 420:126599. https://doi.org/10.1016/j.jhazmat.2021.126599.
- Sun, J., X. Dai, Q. Wang, M. C. Van Loosdrecht, and B.-J. Ni. 2019. “Microplastics in Wastewater Treatment Plants: Detection, Occurrence and Removal.” Water Research 152:21–37. https://doi.org/10.1016/j.watres.2018.12.050.
- Sun, M., X.-Z. Wang, R.-Y. Xiong, X. Chen, L.-F. Zhai, and S. Wang. 2023. “High-Performance Biochar-Loaded MgAl-Layered Double Oxide Adsorbents Derived from Sewage Sludge Towards Nanoplastics Removal: Mechanism Elucidation and QSAR Modeling.” Science of the Total Environment 901:165971. https://doi.org/10.1016/j.scitotenv.2023.165971.
- Sun, Y., S. M. Shaheen, E. F. Ali, H. Abdelrahman, B. Sarkar, H. Song, J. Rinklebe, X. Ren, Z. Zhang, and Q. Wang. 2022. “Enhancing Microplastics Biodegradation During Composting Using Livestock Manure Biochar.” Environmental Pollution 306:119339. https://doi.org/10.1016/j.envpol.2022.119339.
- Tang, Y., S. Zhang, Y. Su, D. Wu, Y. Zhao, and B. Xie. 2021. “Removal of Microplastics from Aqueous Solutions by Magnetic Carbon Nanotubes.” Chemical Engineering Journal 406:126804. https://doi.org/10.1016/j.cej.2020.126804.
- Tee, G. T., X. Y. Gok, and W. F. Yong. 2022. “Adsorption of Pollutants in Wastewater via Biosorbents, Nanoparticles and Magnetic Biosorbents: A Review.” Environmental Research 212:113248. https://doi.org/10.1016/j.envres.2022.113248.
- Thakur, V., E. Sharma, A. Guleria, S. Sangar, and K. Singh. 2020. “Modification and Management of Lignocellulosic Waste as an Ecofriendly Biosorbent for the Application of Heavy Metal Ions Sorption.” Materials Today: Proceedings, February 19-21, 2020, Chandigarh, India 32:608–619.
- Thang, P. Q., K. Jitae, B. L. Giang, N. M. Viet, and P. T. Huong. 2019. “Potential Application of Chicken Manure Biochar Towards Toxic Phenol and 2,4-Dinitrophenol in Wastewaters.” Journal of Environmental Management 251:109556. https://doi.org/10.1016/j.jenvman.2019.109556.
- Tong, M., T. Li, M. Li, L. He, and Z. Ma. 2020. “Cotransport and Deposition of Biochar with Different Sized-Plastic Particles in Saturated Porous Media.” The Science of the Total Environment 713:136387. https://doi.org/10.1016/j.scitotenv.2019.136387.
- Tran, H. N., Y.-C. Wen, Y.-F. Wang, and S.-J. You. 2019. “Highly Efficient Removal of Hazardous Aromatic Pollutants by Micro-Nano Spherical Carbons Synthesized from Different Chemical Activation Methods: A Comparison Study.” Environmental Technology 40 (11): 1376–1391. https://doi.org/10.1080/09593330.2017.1422551.
- Tumwesigye, E., C. F. Nnadozie, F. C. Akamagwuna, X. S. Noundou, G. W. Nyakairu, and O. N. Odume. 2023. “Microplastics as Vectors of Chemical Contaminants and Biological Agents in Freshwater Ecosystems: Current Knowledge Status and Future Perspectives.” Environmental Pollution (Barking, Essex: 1987) 330:121829. https://doi.org/10.1016/j.envpol.2023.121829.
- Vijay, D., H. Zipse, and G. N. Sastry. 2008. “On the Cooperativity of cation− π and Hydrogen Bonding Interactions.” The Journal of Physical Chemistry B 112 (30): 8863–8867. https://doi.org/10.1021/jp804219e.
- Volesky, B. 2001. “Detoxification of Metal-Bearing Effluents: Biosorption for the Next Century.” Hydrometallurgy 59 (2–3): 203–216. https://doi.org/10.1016/S0304-386X(00)00160-2.
- Volesky, B. 2007. “Biosorption and Me.” Water Research 41 (18): 4017–4029. https://doi.org/10.1016/j.watres.2007.05.062.
- Wang, J., C. Sun, Q.-X. Huang, Y. Chi, and J.-H. Yan. 2021a. “Adsorption and Thermal Degradation of Microplastics from Aqueous Solutions by Mg/Zn Modified Magnetic Biochars.” Journal of Hazardous Materials 419:126486. https://doi.org/10.1016/j.jhazmat.2021.126486.
- Wang, Z., M. Sedighi, and A. Lea-Langton. 2020. “Filtration of Microplastic Spheres by Biochar: Removal Efficiency and Immobilisation Mechanisms.” Water Research 184:116165. https://doi.org/10.1016/j.watres.2020.116165.
- Wang, Z., C. Sun, F. Li, and L. Chen. 2021b. “Fatigue Resistance, Re-Usable and Biodegradable Sponge Materials from Plant Protein with Rapid Water Adsorption Capacity for Microplastics Removal.” Chemical Engineering Journal 415:129006. https://doi.org/10.1016/j.cej.2021.129006.
- Weber, K., and P. Quicker. 2018. “Properties of Biochar.” Fuel 217:240–261. https://doi.org/10.1016/j.fuel.2017.12.054.
- Wu, J., C. Yang, H. Zhao, J. Shi, Z. Liu, C. Li, and F. Song. 2022. “Efficient Removal of Microplastics from Aqueous Solution by a Novel Magnetic Biochar: Performance, Mechanism, and Reusability.” Environmental Science and Pollution Research 30 (10): 26914–26928. https://doi.org/10.1007/s11356-022-24130-1.
- Xie, L., D. Yang, Q. Lu, H. Zhang, and H. Zeng. 2020. “Role of Molecular Architecture in the Modulation of Hydrophobic Interactions.” Current Opinion in Colloid & Interface Science 47:58–69. https://doi.org/10.1016/j.cocis.2019.12.001.
- Yen, P.-L., C.-H. Hsu, M.-L. Huang, and V. H.-C. Liao. 2022. “Removal of Nano-Sized Polystyrene Plastic from Aqueous Solutions Using Untreated Coffee Grounds.” Chemosphere 286:131863. https://doi.org/10.1016/j.chemosphere.2021.131863.
- Yu, J., H. Feng, L. Tang, Y. Pang, J. Wang, J. Zou, Q. Xie, Y. Liu, C. Feng, and J. Wang. 2021. “Insight into the Key Factors in Fast Adsorption of Organic Pollutants by Hierarchical Porous Biochar.” Journal of Hazardous Materials 403:123610. https://doi.org/10.1016/j.jhazmat.2020.123610.
- Yuan, F., L. Yue, H. Zhao, and H. Wu. 2020. “Study on the Adsorption of Polystyrene Microplastics by Three-Dimensional Reduced Graphene Oxide.” Water Science and Technology 81 (10): 2163–2175. https://doi.org/10.2166/wst.2020.269.
- Zhang, P., Y. Li, Y. Cao, and L. Han. 2019. “Characteristics of Tetracycline Adsorption by Cow Manure Biochar Prepared at Different Pyrolysis Temperatures.” Bioresource Technology 285:121348. https://doi.org/10.1016/j.biortech.2019.121348.
- Zhang, Y., Y. Luo, X. Guo, T. Xia, T. Wang, H. Jia, and L. Zhu. 2020. “Charge Mediated Interaction of Polystyrene Nanoplastic (PSNP) with Minerals in Aqueous Phase.” Water Research 178:115861. https://doi.org/10.1016/j.watres.2020.115861.
- Zhao, B., X. Xu, R. Zhang, and M. Cui. 2021. “Remediation of Cu (II) and Its Adsorption Mechanism in Aqueous System by Novel Magnetic Biochar Derived from Co-Pyrolysis of Sewage Sludge and Biomass.” Environmental Science and Pollution Research 28 (13): 16408–16419. https://doi.org/10.1007/s11356-020-11811-y.
- Zhu, G., J. Wang, X. Wang, Y. Liu, W. Wu, H. Xiao, L. Huang, H. Dai, X. Zhou, and H. Bian. 2023. “Aerogels Fabricated from Wood-Derived Functional Cellulose Nanofibrils for Highly Efficient Separation of Microplastics.” ACS Sustainable Chemistry & Engineering 11 (38): 13928–13938. https://doi.org/10.1021/acssuschemeng.3c02582.
- Zhu, N., Q. Yan, Y. He, X. Wang, Z. Wei, D. Liang, H. Yue, Y. Yun, G. Li, and N. Sang. 2022. “Insights into the Removal of Polystyrene Nanoplastics Using the Contaminated Corncob-Derived Mesoporous Biochar from Mining Area.” Journal of Hazardous Materials 433:128756. https://doi.org/10.1016/j.jhazmat.2022.128756.
- Zhuang, J., M. Pan, Y. Zhang, F. Liu, and Z. Xu. 2023. “Rapid Adsorption of Directional Cellulose Nanofibers/3-Glycidoxypropyltrimethoxysilane/polyethyleneimine Aerogels on Microplastics in Water.” International Journal of Biological Macromolecules 235:123884. https://doi.org/10.1016/j.ijbiomac.2023.123884.
- Zhuang, J., N. Rong, X. Wang, C. Chen, and Z. Xu. 2022. “Adsorption of Small Size Microplastics Based on Cellulose Nanofiber Aerogel Modified by Quaternary Ammonium Salt in Water.” Separation and Purification Technology 293:121133. https://doi.org/10.1016/j.seppur.2022.121133.