ABSTRACT
Recent progress in mammalian cell culture process has resulted in significantly increased product titers, but also a substantial increase in process- and product-related impurities. Due to the diverse physicochemical properties of these impurities, there is constant need for new technologies that offer higher productivity and improved economics without sacrificing the process robustness required to meet final drug substance specifications. Here, we examined the use of new synthetic adsorptive hybrid filters (AHF) modified with the high binding capacity of quaternary amine (Emphaze™ AEX) and salt-tolerant biomimetic (Emphaze™ ST-AEX) ligands for clearance of process-related impurities like host cell protein (HCP), residual DNA, and virus. The potential to remove soluble aggregates was also examined. Our aim was to develop a mechanistic understanding of the interactions governing adsorptive removal of impurities during filtration by evaluating the effect of various filter types, feed streams, and process conditions on impurity removal. The ionic capacity of these filters was measured and correlated with their ability to remove impurities for multiple molecules. The ionic capacity of AHF significantly exceeded that of traditional adsorptive depth filters (ADF) by 40% for the Emphaze™ AEX and by 700% for the Emphaze™ ST-AEX, providing substantially higher reduction of soluble anionic impurities, including DNA, HCPs and model virus. Nevertheless, we determined that ADF with filter aid provided additional hydrophobic functionality that resulted in removal of higher molecular weight species than AHF. Implementing AHF demonstrated improved process-related impurity removal and viral clearance after Protein A chromatography and enabled a two-step purification process. The consequences of enhanced process performance are far reaching because it allows the downstream polishing train to be restructured and simplified, and chromatographic purity standards to be met with a reduced number of chromatographic steps.
Introduction
Medicine has experienced substantial changes in the past decade as biologic medications have enabled successive generations of advanced therapies against diseases such as cancer, diabetes, arthritis, and colitis. There are currently more than 50 antibody-based therapeutics, including biosimilars, approved for treatment of medical conditions around the world, and over 500 more moving through pre-clinical and clinical pipelines.Citation1,2 To reach the market, the safety, clinical efficacy, and quality of the product must be demonstrated, and adequate manufacturing capacity must be available at all stages of the process. Two critical aspects of biopharmaceutical product manufacturing are the time-complexity quotient of producing Phase 1 and 2 clinical material and production cost of commercial material in large quantities. The former is concerned with speed and the ability to overcome challenges related to product and process biochemistry and resourcing within the context of a fast-moving candidate pipeline. The performance and success of the latter hinges on the cost of goods (COGs) and productivity per facility per year (g/sqft/yr). While it may not be immediately apparent, both stages of the process greatly benefit from a platform approach to bioprocess engineering with a high degree of robustness, and compact and highly productive manufacturing systems.
Within the past two decades, a great deal of investment and advancement has been made in cell-culture process for biologics product expression.Citation3-6 These upstream advancements have put considerable pressure on purification processes,Citation6,7 which involve isolating the target protein from a complex mixture that includes host cell proteins (HCPs), residual DNA, aggregates, media components, virus, and endotoxins. Higher titer correlates with increased amounts of these impurities, and poses both capacity and impurity challenges to downstream purification.Citation8 Furthermore, increasing cell densities, longer cell-culture duration, and higher product titers have challenged the basic design of the downstream train to deal with additional cell mass, cell debris, HCP, DNA, adventitious and endogenous viruses, and other impurities like media components.Citation5,9 The ability to overcome these obstacles necessitates the development of new materials with unique properties, such as membranes with novel retention or very low-fouling characteristics, chromatographic ligands with unique binding characteristics, materials designed to facilitate non-traditional unit operations (e.g., precipitation, flocculation, high capacity adsorptive filters, and charged ultrafiltration membranes), that have the potential to dramatically improve downstream processing, both in terms of capacity and impurity removal capability. Furthermore, in addition to newer materials with superior characteristics, innovative methods of operating purification processes are desirable.Citation10,11 The development of laterally fed membrane adsorbers is a recent advance wherein significant improvement was observed by changing the flow-geometry of existing membrane adsorber chemistry.Citation12 Other groups have leveraged process parameters like column loadingCitation13 or wash conditions during chromatography.Citation14
Biologics purification processes are commonly designed around the primary-capture-step architecture, which typically involves an affinity-based chromatography step. In a monoclonal antibody (mAb) product purification platform, the capture step is especially convenient because it is a Protein A affinity step.Citation10,15 Yet, in a modern industrial mAb purification process, the 95–98% purity of Protein A-eluted product with respect to DNA and HCP is insufficient, and a complex and expensive polishing train is still required. Complexes of DNA and DNA-histone have been reported to be a major component of cell culture fluid interfering with protein chromatography.Citation8,16,17 While Protein A and the subsequent viral inactivation removes most of those impurities, remaining levels of process- and product-related impurities present significant challenges downstream. Previous studies have demonstrated that most impurities that co-purify with the antibody do so by associating with the antibody itself.Citation18,19 HCPs associate with antibodies through complex modes of interaction employing a wide variety of forces like electrostatic interactions, hydrogen bonds, hydrophobic interactions or van der Waal's forces.Citation14 Proteomic-based approaches to investigate the profiles of HCPs present in harvest cell culture fluid (HCCF) and the eluates from Protein A column led to identification of specific populations that exhibit strong interaction with antibodies.Citation18-26
It was recently reported that clarification of mAb-containing Chinese hamster ovary (CHO) cell culture fluid using an adsorptive filter utilizing a Q functionalized non-woven material technology can significantly improve mAb purity eluted from the Protein A capture.Citation27 Several groups have demonstrated the ability of adsorptive filters to lower HCP and DNA levels in a Protein A pool.Citation28-31 In a recent study, Iskra et al. demonstrated increased robustness of xenotropic murine leukemia virus (xMuLV) clearance across a downstream anion-exchange (AEX) chromatography step due to enhanced impurity removal from adsorptive filters after Protein A pool.Citation32 Adsorptive filters have also been shown to provide viral clearance capability by either electrostatic adsorption or a combination of adsorption and mechanical entrapment.Citation33-36 However, implementation of adsorptive filters for claimable impurity and virus clearance in GMP manufacturing processes has been hindered by: 1) relatively poor and variable binding capacity (no consistency), 2) lack of testing methods to demonstrate the post-usage filter integrity, and 3) poor understanding of viral clearance mechanism.Citation36
This work investigates the application of a new generation of adsorptive filters to industrially relevant mAb product purification processes, and explores their effect on product purity post capture. Our focus was on gaining a qualitative mechanistic understanding of the interactions governing adsorptive removal of impurities during filtration by evaluating the effect of various filter types, feed streams, and process conditions on impurity removal. The AEX capacity of adsorptive hybrid filter (AHF) technology significantly exceeds that of conventional adsorptive depth filters (ADF) filters, providing substantially higher reduction of soluble anionic impurities including DNA and anionic HCPs. The particular technology of interest for post-Protein A polishing application is a hybrid Emphaze™ ST-AEX (EMP ST-AEX) prototype technology, which utilizes a guanidinium-functionalized polyamide membrane protected by a Q functionalized non-woven material, similar to that used by Castro-Forero et al.Citation27 that also demonstrated viral clearance log reduction value (LRV) of greater than 4 LRV at higher salt concentrations. One of the strategies that these high purities enable is the use of single-use AEX technologies, such as adsorptive filters, in the polishing train even at full manufacturing scale. This study demonstrates the viability of replacing the depth filtration and AEX steps with a compact and highly effective use technology to achieve a simplified and cost-effective process. To our knowledge, this is the first study to demonstrate removal of HCP, DNA and model virus from four molecules (three recombinant mAbs and a fusion protein), using an adsorptive filter technology that replaced both traditional depth filtration and a flow-through anion exchanger, thus enabling process compression. This demonstrated the practical utility of the technology, as it expands on data obtained using model feed streams. Moreover, the train incorporating the AHF did not incorporate a dilution/conductivity adjustment step, and provided a final process pool of much higher concentrations prior to virus filtration and ultra/diafiltration (formulation).
Results
Our aim was to demonstrate process compression using easy to implement, single use AHFs like the commercial EMP AEX with the quaternary ammonium functionality and the pre-commercial EMP ST-AEX that contains a novel guanidinium-based membrane along with the quaternary ammonium (Q) functionalized hydrogel bed. Both of these devices are capable of reducing process and product related impurities, and offer robust performance in a wider range of operating conditions. The specifics of the biologics used in this study are provided in . The ADFs we used were the Zeta Plus™ 60SP05A and the 90ZB05A filters from 3M; the AHFs were the EMP AEX and the EMP ST-AEX, also obtained from 3M. The physical characteristics of these filters is provided in . Our work focused on the capacity of ADFs and AHFs in terms of their binding capacity to bovine serum albumin (BSA), their capability to remove HCP and DNA, and their capability to remove high molecular weight (HMW) aggregates.
Table 1. Molecule Properties and Impurity Level in post viral inactivation (VI) Protein A eluate neutralized (PAEN) pools.
Table 2. Adsorptive Depth Filters (ADFs) and adsorptive hybrid filters (AHFs) in lenticular format used for the study.
BSA binding capacity
The target location of the ADFs or the AHFs was after neutralizing the viral inactivated Protein A pool. At this stage, several HCPs precipitate. Since the isoelectric point (pI) of most populations of HCPs tend to be between 4.5 and 7,Citation14,19 a model protein like BSA was used to obtain binding capacity data with ADFs and AHFs. The EMP ST-AEX hybrid filter had the highest binding capacity for BSA (52 ± 4 mg/mL) among the AHF, and about an order of magnitude larger than any of the commercially available ADF (0–5 mg/mL) (). In addition, it was the most salt tolerant; BSA capacity was 30 mg/mL and 20 mg/mL at conductivities of 15 and 30 mS/cm salt conditions, respectively, indicating only a 33% reduction in BSA binding for a 2-fold increase in conductivity. In contrast, EMP AEX demonstrated the same binding capacity as EMP ST-AEX at a conductivity of 5 mS/cm. Increasing the conductivity to 30 mS/cm led to complete flow-through of the BSA. This is a significant finding because the major HCPs have an average pI around 5.0 and are negatively charged at neutral to near neutral pH. BSA, being a model protein, provides direct insight into the anticipated HCP binding using ADFs and AHFs.
Enhancing impurity clearance by optimizing neutralization of Post-Protein A pools
We tested various adsorptive filters in combination with optimized neutralization pH to demonstrate process simplification. All molecules were subjected to preparative-scale purification using Protein A chromatography with optimized Protein A washes and optimized neutralization pH identified in . The HCP and DNA levels in viral inactivated (VI) and subsequent pH adjusted post 0.2 µm filtration pools for mAb A is shown in , with data clearly demonstrating an optimum pH of 6 for minimized impurity levels. Whether the mAb of interest co-precipitated with the other proteins is a concern. This was not found to happen, as demonstrated in data shown in .
Figure 2. Post Viral Inactivation (VI) Neutralization pH Optimization. Neutralization to optimal pH after low pH hold for viral inactivation leads to selective precipitation of HCPs. Shown above are the recovery of mAb and HCP/DNA levels in viral inactivated and neutralized bulk samples post 0.2 µm filtration for mAb A.
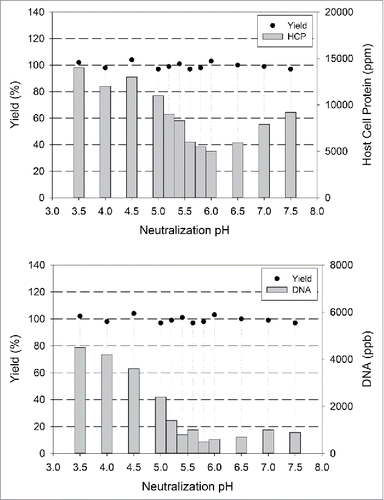
The hydraulics of the ADFs and AHFs at the neutralization pH (pH range 5 - 6) are provided for mAb A in . As is evident from , the ADFs and AHFs did not show a significant increase in resistance even at a filtration flux of 300 LMH, indicating that the high capacity does not come at the cost of high hydraulic resistance.
Figure 3. Resistance profiles as a function of throughput for neutralized mAb A bulk samples. Shown above are the resistance levels for neutralized bulk samples with different filters with 0.2 µm filtration as the refrence used for comparison. The data was collected at a filtration flux of 300 LMH for all the filters.
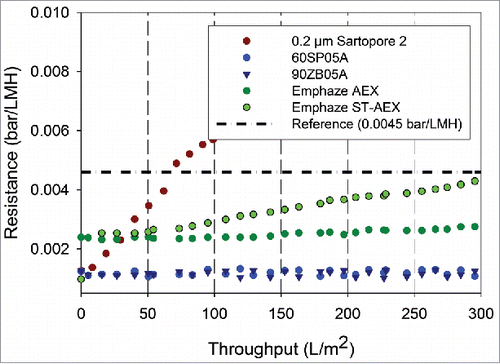
Process-related impurity (HCP and DNA) removal with adsorptive filters
Levels of process-related impurities (HCPs and DNA) and product-related impurities (HMW species) in adsorptive filtrate samples were monitored as a function of loading. and show HCP and HMW levels in the filtrate as a function of loading. Higher removal was observed for AHF as opposed to ADF for mAb A, mAb B, and mAb C at a terminal loading of 200 L/m2. EMP ST-AEX demonstrated the highest HCP removal compared with other filters with the fold reduction values (FRV) achieved greater than 10 for most molecules. EMP AEX (4–5 FRV) and Zeta Plus 90ZB05A (2–4 FRV) also showed significant HCP removal in the Protein A eluate neutralized (PAEN) pool. Additional analyses showed that very little HCP was removed across the Zeta Plus 60SP05A, as expected, because of the larger pores and, thus, lower accessible charge density of the filter. It was also observed that HCP clearance is higher at higher supernatant pH, which is consistent with an interaction between negatively charged HCP and positively charged ADF as more HCPs will be negatively charged at higher pH.
Figure 4. Breakthrough profiles for HCP and HMW levels in adsorptive filterate. HCP and HMW content in filtrate samples for mAb A (HCP: 5000 ± 1000 ppm and HMW: 6.9 ± 0.6%) at a target loading of 200 L/m2. (A) Relative HCP levels were compared across filters as a function of increased loadings. (B) Relative HMW levels were compared were compared across filters as a function of increased loadings.
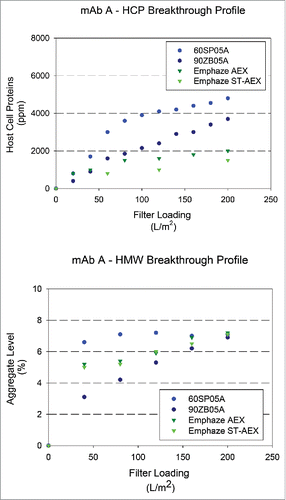
Figure 5. Breakthrough profiles for HCP and HMW levels in adsorptive filterate. HCP and HMW content in filtrate samples for mAb B (HCP: 2500 ± 400 ppm and HMW: 4.5 ± 0.2%) at a target loading of 200 L/m2. (A). Relative HCP levels were compared across filters as a function of increased loadings. (B). Relative HMW levels were compared were compared across filters as a function of increased loadings.
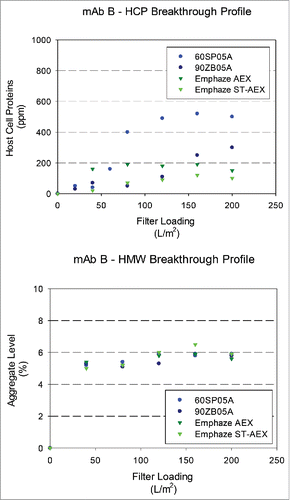
Figure 6. Breakthrough profiles for HCP and HMW levels in adsorptive filterate. HCP and HMW content in filtrate samples for mAb C (HCP: 28000 ± 8000 ppm and HMW: 9.1 ± 0.9%) at a target loading of 200 L/m2. (A). Relative HCP levels were compared across filters as a function of increased loadings. (B). Relative HMW levels were compared were compared across filters as a function of increased loadings.
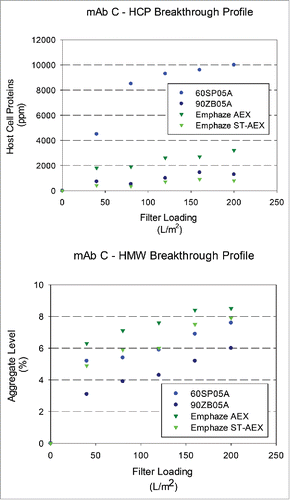
EMP ST-AEX demonstrated the highest impurity removal and no breakthrough at a loading of 200 L/m2 (corresponding to an approximate capacity of 3 kg of mAb per liter of EMP ST-AEX media). It was also observed that HCP level for EMP ST-AEX was pH-independent (Supplementary Information). These observations strongly suggest that the HCP and DNA removal is governed predominantly by electrostatic interactions, but additional interactions such as hydrogen bonding also play an important role. also shows the HCP levels for ST-AEX were not different versus three pH levels of 5, 6,7 (p > 0.05) or pH levels of 4, 5,6 (p > 0.05), giving a HCP content in the filtrate pool between 100 and 450 ppm regardless of the pH used in the study. In contrast, HCP clearance by EMP AEX was sensitive to the pH used for the PAEN pools. Data in shows the variation of DNA LRV as a function of pH for the ADF and AHF. The DNA LRV corresponded well with the ionic capacity. The 60SP05A ADF had the lowest LRV, with the LRV being a maximum at pH 6. The 90ZB05A ADF, EMP AEX and EMP ST-AEX showed the same LRV for DNA (p > 0.05), and this was not a function of pH (p > 0.05).
Figure 7. HCP content as a function of pH at a target loading of 200 L/m2. Host cell protein (HCP) content in filtrate samples remains lower at higher supernatant pH, which is consistent with an interaction between negatively charged HCP and positively charged filters. (A). Relative HCP levels were compared across runs for mAb A. (B). Relative HCP levels were compared across runs for mAb B.
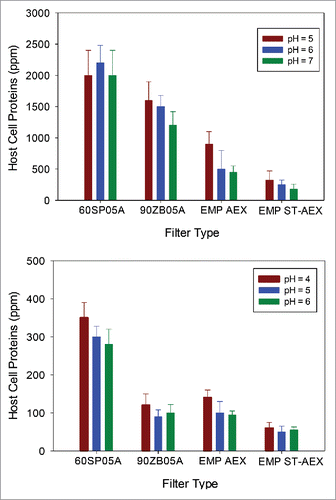
Figure 8. Reduced DNA levels as a function of pH at a target loading of 200 L/m2 for mAb A. DNA levels insensitive to higher supernatant pH, which is consistent with interaction of highly negatively negatively charged DNA and positively charged filter.
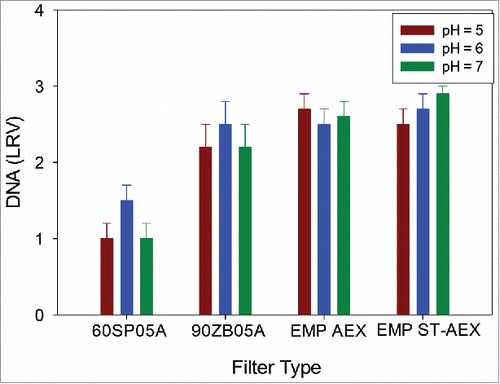
Data for HCP removal at different salt levels showed that the HCP levels for EMP ST-AEX were similar across different salt levels (), whereas this was not the case for EMP AEX. There was at least a 5-fold reduction (FRV) even at a loading of 5 kg/L, which demonstrates that the EMP ST-AEX has a higher HCP removal capacity resulting from salt tolerance ().
Figure 9. HCP levels in EMP ST-AEX filtrate pool as a function of salt concentration for mAb A and mAb B. HCP levels in EMP ST-AEX filtrate pools as a function of salt concentration at three different loadings (1, 3, and 5 kg/L). Data suggests improved HCP removal at higher salt concentration
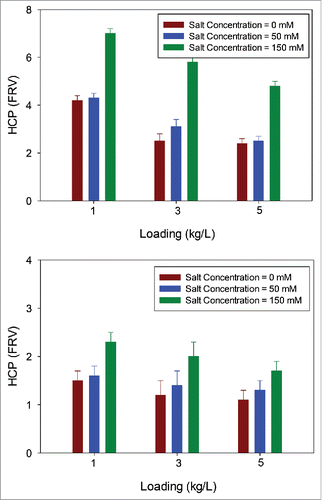
Figure 10. Aggregate Profiles of Protein A before and after ADF (90ZB05A) The size-exclusion chromatography (SEC) assay method quantifies the percent distribution of aggregates species (HMW1, HMW2, and HMW3) for mAb A and mAb C. 90 ZB05A shows removal most of HMW1 peak but not HMW2 and HMW3 species. HMW1 species showed higher hydrophobicity than HMW2 and HMW3 determined by hydrophobic interaction liquid chromatography (HIC-HPLC), suggesting the removal of HMW1 by ADF may be due to the hydrophobic interaction.
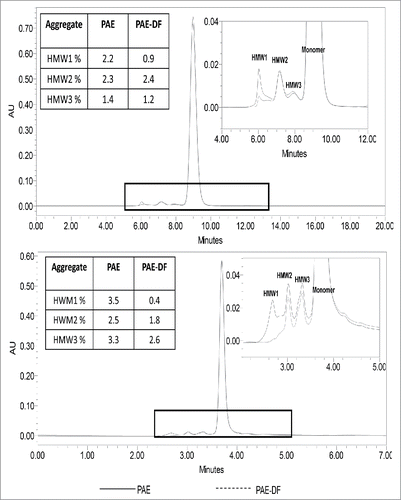
Aggregate removal with adsorptive depth filters
Our data did not show significant aggregate removal using the AHFs. However, we observed the traditional ADF demonstrated aggregate removal that was a function of the loading to the ADF (–). As described in the Materials and Methods section, aggregates were identified using SEC-HPLC. Samples were collected from various fractions of filtrate analyzed for HMW levels. As shown in –, HMW levels were found to be lower in initial fractions and then reached a saturation level with Zeta Plus 90ZB05A ADF.
Viral Clearance using adsorptive hybrid filters
Process capability to remove viral contaminants from biological process streams is an integral part of the manufacturing process during the purification of biologics.Citation37-41 Current regulations require that the manufacturing process demonstrate the ability to clear model viruses to ensure the safety of these cell-line-derived products prior to approval, with additional built-in safety for robustness.Citation42-44 AEX chromatography has been frequently applied as a viral clearance step during downstream processing, in addition to impurity clearance of molecular species such as HCP and DNA.Citation44 This necessitates the claimable viral clearance capability from AHF to eliminate the AEX step. This strategy is appropriate when the therapeutic protein has a pI higher than the virus particle, as is the case for most IgG mAbs. Process conditions such as pH and conductivity are then set so that the acidic virus particles are charged negatively and bind to the membrane, while the basic protein product is positively charged and flows through. The positively charged anion exchanger adsorbs the negatively charged virus particles and repels the positively charged protein product by electrostatic charge repulsion, allowing it to flow through. Previously, it has been demonstrated that greater than 3–4 log reduction of viruses can be achieved by using ADF post Protein A.Citation33,34,45 With the advent of new AHFs, Muller et al. have demonstrated even higher clearance of viruses by filtration post low pH viral inactivation and neutralization.Citation46 Nonetheless, validation of ADFs is difficult for viral clearance due to the inherit media variations at micron length scale and below. Depth filters that are marketed toward viral reduction (such as 3M Zeta Plus VR series) have a defined specification on the amount of accessible charge that a given bed volume contains, but not on the uniformity of media or charge distribution across the filter at sub-micron level. This significantly limits the log reduction value (LRV) that one can validate with such a filter.
Unlike ADFs, AHFs are completely synthetic with molecular-level architectural media uniformity, and can be validated using similar methods to those used with chromatography columns or membrane adsorbers. Integrity of the AHF filters was tested pre-use and post-use by pressuring (with wetted membrane) the upstream part of the device to 20 psi and looking for pressure drop within a 30 second interval. In every case, the pressure fell no more than 0.1 psi within 30 second period. Such a minor pressure drop is, typically, attributed to the air slipping past the peristaltic pump lobes. This test was intended to discriminate between defective and intact capsules and to detect major bypasses, large holes and faulty assembly. Moreover, the non-woven material cannot be integrity tested using membrane methods, such as a pressure hold test, because the pressure differential across the nonwoven material itself is very low in all typical process conditions and effectively measures properties of the membrane that is backing the nonwoven material. Therefore, the ion exchange capacity (ligand density) was developed to test the integrity of anionic AHF that was demonstrated to be correlated with viral LRV (data not shown). Viral clearance is supposed to be completely driven by electrostatic interactions for AHFs, as opposed to hybrid mechanism of size exclusion, electrostatic and hydrophobic interaction in case of ADFs, and, therefore, ion exchange capacity test is more appropriate for AHFs.
In order to demonstrate the viral clearance, AHFs were analyzed for clearance of bacteriophage PR772 and ϕX174 at low and high conductivities: low salt (20 mM Tris) and high salt (20 mM Tris, 150 mM NaCl). While the pIs of PR772 (3.8–4.2) and ϕX174 (6.6–7.2) differ from typical mammalian model viruses such as MuLV (5.8) and MMV (6.2),Citation43,47,48 they are in the same general range, that is, mildly acidic to neutral. Whether phage can be representative of mammalian viruses when studying viral clearance is controversial. In either case, any data that furthers the mechanistic understanding of adsorptive filters may lead to better process optimization. Despite the difference in dynamic binding capacity, AHF based on the Q ligand performed the same, clearance fell in the range 5.6–6.4 LRV (p<0.05; 95% confidence interval) in buffer with no added salt and was not different from 0 LRV (p > 0.05) upon addition of 150 mM salt for the small phage ϕX174 and large phage PR772. This indicates that Q ligand had sufficient capacity to successfully clear the virus at lower conductivities consistent with the BSA and HCP binding. However, there was no capacity at higher conductivities, suggesting poor salt tolerance. Despite the higher conductivity for the samples tested with AHF based on the guanidinium ligand, LRV was less affected by the presence of salt. In particular, clearance fell in the range 5.9–6.5 LRV (p<0.05, 95% confidence interval) for both low and high conductivities. A previous study with salt tolerant membrane adsorbers, which were surface modified with guanidinium ligands similarly observed higher LRV values for ϕX17449 (up to an LRV of 8.8 with 0 mM added NaCl and 7.1 for 150 mM added NaCl).
Also, ϕX174 and PR772 were tested with mAb A, mAb B and mAb C where it demonstrated > 4LRV at the optimum pH (pH of solution > pI of phage). The average virus log reduction value (LRV) of the small phage ϕX174 and large phage PR772 at various loadings, pH, and salt levels is given in . It was shown that EMP ST-AEX effectively cleared PR772 above pH 4.2 and 4 mS/cm condition for mAb A and mAb B, achieving LRV greater than 6 (LOD). The ST-AEX hybrid filter was also effective for PR772 removal at higher conductivities (15 mS/cm), achieving LRV greater than 6.
Table 3. PR772 and ΦX174 clearance in Post Protein A pools for three mAbs with adsorptive hybrid filters (AHFs).
Discussion
Here, we examined the use of synthetic AHF as an alternative to chromatographic polishing steps for the purification of biologics. This is the first comprehensive study to demonstrate the use of AHF to reduce HCP, DNA and virus and demonstrate process compression using mAbs. Data was obtained for model protein (BSA) binding as a function of ionic strength for adsorptive filters (AHFs and traditional ADFs) to estimate the HCP removal that would be possible during biologics purification. As expected, ADFs demonstrated low BSA binding capacities that is consistent with the lower impurity removal observed with process-related impurities in purification of biologics. In contrast, the AHF (EMP ST-AEX) demonstrated an almost 20x higher binding capacity of BSA compared with the ADFs, with a good degree of salt tolerance. Data was then obtained using three mAbs post Protein A and viral inactivation as a function of pH and conductivity to observe the HCP, DNA and viral clearance as a function of these conditions. The EMP ST-AEX provided significant HCP, DNA and model viral clearance comparable to or exceeding the performance of membrane adsorbers. An integrity and validation test is provided for the EMP STAEX. On the contrary, the ADFs provided better removal of product-related impurities like HMW aggregates, the removal being a strong function of loading. This was attributed to hydrophobic interactions of the HMW aggregates with the hydrophobic media components, primarily diatomaceous earth, used in ADFs. Process compression was confirmed by the experimental data, which also showed that there was no in-process dilution in the process that contained the AHF. This helped in debottlenecking and allowed for an increased protein concentration post purification. In this way, chromatographic purity was achieved with a reduced number of chromatographic steps, making AHF an attractive option for bioprocessing.
BSA binding capacity experiments were performed to understand the potential of HCP removal using adsorptive filters. The hypothesis was that, if the adsorption of HCP to the ADF or AHF was purely electrostatic, then a model protein with a similar pI as the HCP should provide similar binding to the ADF and AHF. We found that the BSA binding capacity at pH 7.4 was a very strong function of conductivity for the ADFs and AHFs, but much less so for the EMP ST-AEX. Thus, it is expected that the HCP removal capacity would be the highest for EMP ST-AEX compared with the other ADFs and AHFs if the interactions were strictly electrostatic.
It must be noted, however, that the correlation of HCP removal to BSA binding capacity cannot be determined only through electrostatic interactions. Riordan et al.Citation50 have examined the structural properties of various ligands, including the quaternary amine (Q), which contains one positive quaternary amine charge; agmatine (AGM), containing two primary amine groups, and two imine groups; tris-2-amino ethyl amine (TAEA), which contains three primary amine groups and one tertiary amine group, as well as polycations like polyhexamethylene biguanide (PHMB), which contains 12 guanidium positive charges per unit, and a primary amine-based polycation like polyethylene imine (PEI). In the analysis of binding capacity and HCP removal, they found that impurity removal is a function of both the charge density on the impurity, and the charge density on the ligand functionalized surface. The free energy of interaction was also found to depend on secondary interactions like hydrogen bonding; PHMB, containing biguanide groups, demonstrated the best ability to form hydrogen bonds. In addition, molecular structure was identified as an important parameter to enhance salt tolerance and impurity binding. All these interactions resulted in PHMB having the highest binding capacity compared with a polycation like PEI. This explains the divergence of binding capacity of the EMP ST-AEX and EMP AEX with increasing conductivity. The EMP ST-AEX utilizes guanidinium functionality along with the quaternary amine (Q), as opposed to the EMP AEX, which has a quaternary amine functionality. In contrast, ADF did not have significant BSA binding capacity, with Zeta Plus 90ZB05A having a slightly higher capacity than 60SP05A. An important observation was the negligible decline in BSA binding capacity (albeit significantly lower than the EMP ST-AEX) even at higher salt concentration for 90ZB05A, which suggests that the hydrophobic interactions play an important role in impurity removal for the traditional ADF (). The data in also shows that changes in the flow-rate (or the filtration flux) do not affect the binding capacity.
Several other groups have reported that there can be significant precipitation and turbidity in product pools during the neutralization of post-Protein A step.Citation14,51 Components that are generally responsible for precipitation at low pH (or during pH adjustment) include product-related species such as HMW aggregates and process-related molecular impurities such as HCPs and DNA.Citation14,52-54 Increased process-related impurities depends upon several factors, e.g., cell line, cell culture duration, viable cell density, viability, metabolite levels in the cell culture, molecule type. The optimum pH for neutralization that resulted in removal of high levels of HCPs and DNA was found to be very molecule specific. However, neutralization pH studies on mAb B, mAb C and fusion protein post viral inactivation yielded results similar to mAb A, with an optimum pH range between 5 and 6, as the majority of HCPs and residual DNA are unstable in acidic pH range between 4.5 and 7.0. In all cases, there was an increase in turbidity of the pools with an increase in pH, and the peak turbidity started decreasing when the pH reached optimum pH (data not shown). Strongly correlating with this turbidity, filtration of the samples resulted in removal of HCPs and DNA from the pool.
Our studies also demonstrated that turbidity associated with Protein A pools and neutralization thereafter was not necessarily an undesirable phenomenon. The yield at all neutralization pHs remained close to 100% (), indicating that the turbidity was a result of impurity precipitation, and not product precipitation. This is usually a consequence of HCPs becoming unstable when the product pool pH is at or close to their pIs, and thus provides a way to further reduce the impurities by filtration. When used in combination with other separation techniques like adsorptive filtration during post-Protein A chromatography, optimal neutralization of product pool post Protein A with filtration can lead to significant removal of HCPs and DNA to drug substance specifications. This essentially eliminated the need for multiple polishing steps downstream of Protein A. On the other hand, precipitation at any point in the bioprocess is difficult to control, so great care must be taken to understand the nature of precipitation and the technical and regulatory risk that it adds to a commercial process.
Our data on process-related impurity removal using ADF and AHF showed pooled HCP removal as a function of loading. The lowest break through of HCP was observed specifically for the AHF EMP ST-AEX. EMP ST-AEX is a hybrid system, utilizing guanidinium functionalized polyamide membrane, protected by a Q functionalized non-woven material (similar to that used by Castro-Forerro et al.Citation27 Guanidinium ligand, unlike quaternary ammonium or primary amine chemistry, is able to retain charge in a much wider range of conditions due to highly stabilized resonance structure. It is a biomimetic ligand that utilizes the same AEX group as the amino acid arginine (Arg). Arg is one of 3 cationic amino acids that has a delocalized cationic group charged over a wide pH range (pKa = 12.48). It also forms salt bridges with carboxyl residues of aspartic acid and glutamic acid that involve both electrostatic and hydrogen bonding interactions. The majority of interactions are in-plane, enabling bidentate hydrogen bonding interactions. It is known that arginine-carboxyl interactions account for nearly 40% of ion pairs within proteins, and are important in controlling protein conformation, it is our hypothesis that these play a significant role in HCP removal with EMP ST-AEX ligand. The Q functional nonwoven upstream of the guanidinium functionalized membranes is capable of protecting the membrane from turbidity and DNA in PAEN pools, enabling an additional degree of robustness.
Our data for the variation of the HCP and DNA removal capability demonstrate the sensitivity of ADFs and AHFs to typical operating conditions employed in the mAb manufacturing process. Depending on the bioreactor volume and the Protein A loading, it is possible that the loading on the ADF or AHF would potentially change. Thus, maximizing performance at minimum area becomes important. The 5-fold reduction (FRV) even at a loading of 5 kg/L demonstrates that the EMP ST-AEX has a higher HCP removal capacity resulting from salt tolerance (). The insensitivity of the EMP ST AEX to ionic conditions is also very important because it would also extend the allowable operating ionic strength range for the process and reduce buffer dilution requirements.
At higher salt concentrations, electrostatic interactions between the impurities and surface are weakened due to charge shielding by counter-ions, making adsorption energetically unfavorable. Thus, the charge shielding renders the ADF and the EMP AEX low binding capacities at higher salt concentrations. Secondary interactions like hydrogen bonding increase the magnitude of the total interaction energy between the impurities and the surface, making adsorption possible under conditions where electrostatics alone would not. The guanidinium group may have been particularly effective as a salt-tolerant ligand because its large, delocalized charge allows it to transiently form two hydrogen bonds. This hypothesis is supported by previous reports, and this study extends the observation of Riordan's workCitation55 to mAb purification and demonstrate process compression.Citation49,56
In all other cases, there was no significant decrease in the levels of HMW species. ADFs are made of composite materials of cellulose fibers, diatomaceous earth and positively-charged binder. In general, two distinct mechanisms are involved in impurity removal by depth filters, namely mechanical entrapment and adsorption via either electrostatic or hydrophobic interactions.Citation28-31 Diatomaceous earth-based depth filters have been demonstrated to remove impurities through hydrophobic interactions.Citation29
We hypothesized that the decrease of HMW1 levels in Zeta Plus 90ZB05A filtrate could be related to differences in hydrophobicity, as determined by hydrophobic interaction chromatography-high performance liquid chromatography (HIC-HPLC). The HMW1, a high-order aggregation species, mainly is a complex composed of product aggregate and high level of impurities including HCPs and DNA. The HMW2 and HMW3 mainly are product dimer and intermediate, respectively. More interestingly, it was found that the HMW1 species showed higher hydrophobicity than HMW2 and HMW3 measured by HIC-HPLC, suggesting the removal of HMW1 by depth filtration may be due to the hydrophobic interaction between the HMW1 and the depth filter. This clearly suggests that diatomaceous earth-based ADFs have an advantage in impurity removal because they provide additional interactions compared with AHFs. The nature of HMW1 species were similar for mAb A and mAb C, explaining the trend of HMW1 removal using ADF for these mAbs. In the case of mAb B, there was no HMW removal using ADF or AHF, indicating that the interactions between the aggregate species and the ADF media were not significant enough to aid in separation (). To confirm if these findings were “true” and not artifacts of the SEC method, the yield of the mAb was calculated using both the A280 (total protein), the titer method and the SEC chromatograms. Consistent to the HMW removal, a yield reduction was observed anywhere between 4 and 10% depending on the mAb. The HMW removal using ADF was an interesting observation, but we did not further investigation the nature of HMW removal.
The viral clearance using AHFs provided important practical data on virus LRV using AHF, summarized in . These results were similar to what we obtained in the current study with regard to potential effect of pH and conductivity on PR772 clearance in buffer (without the presence of the mAb). There was no ϕX174 removal between pH 4.2 and 6.5, which clearly indicated that electrostatic interactions played a major removal in phage clearance. Since the pI of ϕX174 is roughly around 6.8, it had minimal interaction with EMP ST-AEX ligand, thereby resulting in non-significant removal of phage for mAb A. At a pH value of 7.5, EMP ST-AEX demonstrated a LRV greater than 4, but lesser than obtained in buffer for mAb A, suggesting that there is competitive binding with the protein product, which had an pI of around 7.2–7.8. The LRV increased even further in the presence of higher salt for ϕX174, improving from 4 to 5 LRV for mAb A, which suggests phage has stronger binding at higher conductivities. This was further confirmed by higher LRV values (> 6) obtained for ϕX174 with mAb C. This observation is counter-intuitive and opposite of that reported by Riordan et al.Citation50,55 The reason for higher viral clearance at higher conductivities was not further examined mechanistically. There are 2 possible explanations for the discrepancy in observations. One is that, at higher salt, the virus outcompeted the mAb for binding sites. The second explanation is the difference in linker structure and grafting density between the guanidinium ligand utilized by EMP ST-AEX prototype and that used by Riordan et al. Citation55 However, all observations are consistent with a hypothesis that interactions other than electrostatic interactions become significant in the presence of salt. We did not test this hypothesis because it was beyond the scope of this study. Our observation on viral clearance in conjunction with the HCP and DNA removal is significant because it demonstrates the ability of AHFs to remove process-related impurities consistently. This observation, and the magnitudes of LRVs, have not been previously reported.
Regarding the importance and need for viral clearance, ADFs are difficult to qualify and claim as viral reduction tools because of their inconsistency. This problem was overcome in this work by using high-capacity salt tolerant AHFs that provide HCP, DNA and viral clearance that is less sensitive to variations in conductivity compared with ADFs and an AHF like EMP AEX. The capability to cover the purpose of an anion-exchange polishing step with a positively charged AHF allows the redesign of a three chromatography step mAb purification platform into a more efficient and economical two-step process while maintaining product purity, quality, and safety.
We have emphasized here that AHFs with new and improved salt tolerant chemistries can aid in process compression by reducing the number of steps. In , data for a comparison between a traditional three-step chromatographic process (Protein A, ADF, CEX, AEX) are compared with a compressed process with AHF (Protein A, AHF, CEX). Note that in the traditional process, the ADF provided some impurity clearance, but it was mostly a clarification step to remove the precipitated impurities. A polishing step after CEX was required to provide further purification and viral clearance. In the current train with AHF, the salt tolerant EMP STAEX AHF took care of clarification, purification and viral clearance. As a result, a CEX step was required only to reduce aggregates. The specifics are provided below.
Table 4. Comparison of conventional four-step purification of mAb A to the proposed two-step purification incorporating the adsorptive hybrid filter (AHF). Note that viral clearance data are not presented, but they are comparable in both these steps.
The train for the traditional three-column process consisted of Protein A chromatography, viral inactivation and depth filtration using an ADF (Zeta Plus 90ZB05A), CEX chromatography, dilution to adjust conductivity of the eluate, followed by a flow-through membrane chromatography step with an anion-exchange membrane, loaded to 1 kg mAb A/LMembrane. The train for the new process incorporating the AHF consisted of Protein A chromatography, viral inactivation, filtration using AHF (EMP ST-AEX) loaded to 3 kg mAb A/LBed, followed by CEX chromatography. The new train did not involve any dilution or depth filtration using an ADF. The final process attributes like HCP, DNA, residual Protein A and HMW levels were in line with the drug substance standards for both the processes. In addition to this, the new process produced mAb A at 8 g/L, while the traditional three-column process produced mAb A at 3 g/L post the membrane chromatography step. Thus, the product was 2.7x concentrated after the new process compared with the traditional process. Thus, in addition to the impurities meeting drug substance standards, the new process clearly improved productivity. A higher concentration implied a higher mass loading on the virus filter and a shorter processing time for the UF/DF step to concentrate mAb A to 65 g/L.
We have therefore demonstrated that newer materials with new chemistries indeed work better for purification purposes, and have the capacity to overcome traditional boundaries in effective production of biologics. The EMP ST AEX AHF provided clarification, purification and viral clearance capability, eliminating the use of traditional depth filters for post PAEN pools, and anionic exchange membrane adsorbers for further polishing. In addition, synthetic media used in AHFs with molecular-level architectural media uniformity, can be validated using similar methods to those used with chromatography columns or membrane adsorbers. This was demonstrated by integrity tests, including non-destructive pressure hold and ion exchange capacity tests that overcome limitations of ADFs for a claimable impurity clearance. We believe that, with the development of new chemistries and materials to handle hydrophobic impurities, aggregate removal using AHFs will become a reality, leading to the development of a single-step chromatographic purification process.
Materials and methods
Chinese hamster ovary cell culture conditions
Proprietary Chinese hamster ovary (CHO) cell lines engineered from either DG44 parental cells or GS parental cells were used to produce the harvests containing recombinant mAbs of IgG1 subclass (mAb A, mAb B, mAb C) and a fusion protein (FcP). All cells were cultured using a chemically defined, animal component-free media. The bioreactors were operated in a fed-batch mode with continuous feeds based on maintaining glucose concentrations in the range of 2–4 g/L. Titers were measured using a Protein A HPLC system (Agilent 1100, Waters Corporation, USA) with an established reference standard. The bioreactors were harvested using two-stage depth filtration comprising the Zeta Plus™ family (10SP05A/90ZB05A, 3M, USA) followed by 0.2 µm filter capsule (Sartorius, USA). The HCCF was used for further processing.
Protein A chromatography
MabSelect Protein A resin (GE Healthcare Bio-Sciences, Marlborough, USA) was used for all capture chromatography experiments. Preparative scale column-based chromatography experiments were performed using an ÄKTA Avant instrument (GE Healthcare Bio-Sciences, Marlborough, USA) controlled by Unicorn 6.3 software. All columns were packed in the lab according to the resin manufacturer's recommendations. After sanitization, the equilibration of the resin was performed using phosphate-buffered saline (PBS) at pH 7.4 followed by loading with HCCF. The resin was then subjected to PBS wash followed by an acidic pH wash using 50 mM sodium acetate (pH 5.0–6.0) before eluting the mAb using 50 mM sodium acetate buffer at pH < 4.0.
Viral inactivation and neutralization
Following elution, the mAb pool from Protein A chromatography was subjected to viral inactivation by holding at low pH (3.4 to 4.0) for 1 hour at room temperature, followed by neutralization to a desired pH in a range between 4.0 and 7.0. Titration was performed using 0.1 N HCl or 2 M Tris. Post neutralization, filtration was performed using two different types of adsorptive filters. The first is the traditional ADF from Zeta Plus™ family (60SP05A, 90ZB05A from 3M, USA). The second is a fully synthetic AHF technology from Emphaze™ family – commercial Emphaze™ AEX (EMP AEX) and pre-market prototype technology Emphaze™ ST-AEX (EMP ST-AEX) hybrid filters. All the devices used were 25 cm2 Biocap devices with luer fittings. ZetaPlus™ capsules has a slightly variable bed volume due to variation in the thickness of wet-laid media, but all were 20 mL ± 1 mL. EMP AEX Q functional media bed volume was 20 mL per device. EMP ST-AEX was assembled in a customer 25 mm diameter holder that is custom module with luer fittings, and contained 0.9 mL of Q functional non-woven on top of 0.3 mL of guanidinium functional membrane.
Adsorptive filtration
Following pH adjustment, feed streams (Neutralized Protein A pool) were loaded onto ADF and AHF filters at a flux of 300 L/m2/h (LMH) (unless otherwise specified) to a target loading of 200 L/m2 (unless otherwise specified). AHF combines three technologies: anion-exchange functional polymers, fine-fiber nonwoven materials, and multi-zone membranes to deliver an all-synthetic clarifying product containing both an AEX ligand and a highly asymmetric bioburden-reduction membrane. During filtration, differential pressures were monitored as a function of loading, and filter resistance was calculated as the ratio of differential pressure to filtration flux. Filtration experiments were performed using a PendoTECH instrument (PendoTECH, Princeton, NJ) controlled by NFFSS software. Unless noted, equilibration of the filter was performed using PBS at pH 7.2 with approximately 30 bed volumes (100 L/m2) followed by loading with neutralized Protein A pool sat a loading of 200 L/m2 followed by a PBS chase at 5 bed volumes (15 L/m2).
Ion-exchange capacity
The ionic capacity was measured by flushing the adsorptive filters with 5 bed volumes of 0.1 M NaOH to bind the OH− with the quaternary ammonium or guanidinium moieties and then drained. The drained depth filter was then rinsed with water for injection (WFI), followed by flushing with 1 M NaCl to displace the OH− ions. The hydroxide ions were then titrated against 0.1M HCl to a phenolphthalein end point (pink to colorless). The ionic capacity was measured as (VHCl CHCl)/(VFiltrateVMedia).
Reversible dynamic protein binding capacity: Bind and elute method
Reversible dynamic adsorption capacities for Zeta Plus 60SP05A, 90ZB05A (20 mL bed volume), EMP AEX (8 mL bed volume), and EMP ST-AEX (1.2 mL Q + guanidinium media bed volume) were measured using an ÄKTA Avant 120 chromatography system. Each respective device was attached to an ÄKTA Avant chromatography system. Detailed information is provided in the supplementary information. Briefly, a flow rate of 2 bed volumes (BV)/min was maintained during all steps. A typical injection protocol for a single run was as follows: A protein solution, 3.33 mg BSA/mL was loaded in buffer A (10 mM PBS, pH 7.2) until 100% breakthrough was achieved. To remove unbound protein, devices were washed immediately following protein loading using 10 BVs of buffer A. 10 BV's of buffer E (1 M NaCl in buffer A, adjusted to pH 4.0 with 0.1 M hydrochloric acid) was used for elution. Reversible binding capacities were calculated from the mass of protein in the elution peak, as determined from an independent calibration curve, and the volume of the media bed. Reversible dynamic binding capacities were measured between two and four times for each device, and capacity was reported as the average of the measurements.
The devices described above were also used to determine the effect of ionic strength on reversible binding capacities. Ionic strength was varied by decreasing or increasing buffer concentration in protein binding buffer A. Binding buffers with increased conductivities were prepared with 20, 50, 100 and 150 mM NaCl added to buffer A. The flow rate was 2BV/min. In order to compare our bind-and-elute method for measuring dynamic capacity with a standard method, dynamic binding capacities from protein breakthrough curves were also determined. Dynamic binding capacities were calculated at 10% breakthrough as measured by UV absorbance at 280 nm. Three flow rates, 2BV/min, 4BV/min and 6BV/min, for each of the Zeta Plus 60SP05A, 90ZB05A, EMP AEX, and EMP ST-AEX devices were measured. A UV trigger is used to switch from sample load to wash such that 10% breakthrough was achieved.
Bacteriophage ϕX174 and PR772 preparation
AHFs were evaluated for clearance of ϕX174 and PR772 at elevated salt conditions to gain insights into the physical ligand characteristics that lead to salt tolerance. Bacteriophage ϕX174 and the bacterial host Escherichia coli. C (ATTC 13706) were obtained from the Felix d'Herelle Reference Center for Bacterial Viruses (Quebec, Canada). Bacteriophage ϕX174 was propagated and purified using previously published methods.Citation57 Briefly, 0.1 L of NB media (8 g/L of Difco™ Nutrient Broth (BD Biosciences, USA) plus 5 g/L of NaCl) was seeded with 2.5% (v/v) of an E. coli C culture grown overnight aiming for initial OD600 of 0.05. Overnight culture grew to OD600 of 1.8–2.0. The broth culture was grown at 37°C and 210 rpm until a cell density of about 108 viable bacteria/mL [OD600 = 0.5] was achieved. About 108–109 pfu (plaque-forming units) of ϕX174 was added to E. coli C culture and allowed to propagate in the bacterial culture for 3–6 h at 37°C. When OD600 became smaller than the density by 0.1 unit, the bacterial culture was harvested and centrifuged at 10,000 RCF for 15 min. The supernatant was filtered through a 0.2 μm pore size filter. This supernatant was dialyzed 3 times against 100 volumes of dialysis buffer (20 mM Tris HCl pH 7.0, 10 mM NaCl, 8mM MgSO4) at 4°C. Typical purified titer of ϕX174 was 0.5 × 1010 pfu/mL as determined by plaque method. Phage suspension was stored at 4°C.
The phage PR772 belongs to the Tectiviridae family of icosahedral double-stranded DNA bacteriophages. PR772 has been chosen by the Parenteral Drug Association virus filter task force to be the model bacteriophage to standardize nomenclature for larger-pore-size virus filters. This phage is stable, easy to handle, and high phage titers can be obtained readily. Unlike other members of the family Tectiviridae, production of PR772 does not involve the handling of pathogenic host bacteria. Briefly, the PR772 was produced using the plaque method and purified using dialysis.Citation43 Bacteriophages were grown on the surface of agar plates by the overlay method. For each of 10 to 20 Tryptic Soy Agar plates, 105 PFU of PR772 in 0.1 mL were added to 0.1 mL of overnight bacteria culture and incubated for 5 min at 20 to 25°C. Five milliliters of 50°C soft agar (0.6% agarose in tryptic soy broth) were mixed and poured onto the 10 × 10 cm surface area of one Tryptic Soy Agar plates. After solidification, the plates were incubated at 37°C for 16 h to allow bacterial growth and semi-confluent lysis of the bacterial lawn by PR772. Plaques produced by PR772 were translucent in appearance, indicating incomplete lysis of the surrounding bacteria. The soft agar from all plates was scraped in 50 mL tubes and phages were recovered from it using an equal volume of PBS for 4 h at 20 to 25°C or overnight at 2 to 8°C with gentle agitation on an orbital shaker. The crude washes were centrifuged for 15 min at 10,000 RCF and then filtered through a 0.2 um PES filter for storage or further purification by dialysis against PBS buffer. Typical titer of PR772 was 1 × 1010 PFU/mL and phage suspension was stored at 4°C.
Analytical attributes
Post capture chromatography and filtration, protein concentration were assayed by high-throughput UV-Vis spectroscopy using the DropSense96 polychromatic microplate reader (Trinean, Belgium). UV-Vis spectra were quantified using DropQuant software (Trinean, Belgium). Sub-µm solute size distributions in free solution were characterized by dynamic light scattering (DLS) using a Zetasizer ZS (Malvern Instruments, Worcestershire, UK). The sample (200 µL) was mixed gently for 10 s on a vortex before being placed into a quartz cuvette (ZEN2112, Malvern Instruments) using a gel loading tip to avoid bubbles. SEC was conducted using Waters' Acquity H-class Bio UPLC® to measure the monomer and aggregate content in the product pool. Acquity UPLC® BEH200 SEC 1.7 µm column (4.6×150 mm) was utilized to perform this assay where the mobile phase consisted of 1X PBS at pH 6.8 and was run at a flow rate of 1 mL/min. Quantification of monomer, low molecular weight and the HMW species was performed using Empower software (Waters Corp. Orlando, FL). HIC-HPLC was performed using a Waters Alliance equipped with a PDA detector and a hydrophobic interaction column (TSKgel Butyl-NPR, 4.6 mm × 10 cm, Tosoh Bioscience) to measure the relative hydrophobicity of the aggregates in the product pool. A linear gradient method with a flow rate of 0.5 mL/min was used with mobile phase A (0.1 M potassium phosphate, 0.15 M sodium chloride, pH 6.8, with 2 M ammonium sulfate) and mobile phase B (0.1 M potassium phosphate, 0.15 M sodium chloride, pH 6.8). ELISA for quantification of residual CHO HCP and residual Protein A was conducted by ELISA in a high throughput manner using the Tecan liquid handling system. HCPs were quantified using the CHO Host Cell proteins 3rd generation kit (Cat # F550, Cygnus Technologies, Southport, NC) while residual Protein A was quantified using Repligen Protein A ELISA kit (Cat # 9000–1, Repligen Corporation, USA) according to manufacturer's protocol. Absorbance was measured at 450/650 nm using EnVision Multilabel reader (PerkinElmer. Waltham, MA). Data quantification and analysis was performed using JMP software (SAS Institute Inc. Cary, NC). Residual CHO DNA in the samples were measured using real-time quantitative PCR (RT-PCR). RT-PCR was performed with the 2 x TaqMan Universal PCR Master Mix kit (Life Technologies, USA) and 7900 HT Real Time PCR system (Life Technologies, USA) according the manufacturer's instructions.
Disclosure of potential conflicts of interest
No potential conflicts of interest were disclosed.
Acknowledgments
The authors thank Elizabeth Schutsky, Hannah Doss, Dongyoun Jang and Richard Martel (Bristol-Myers Squibb, Global Manufacturing and Supply) for supporting filtration experiments; Richard Martel and Jita Ray (Bristol-Myers Squibb, Global Manufacturing and Supply) for supporting laboratory scale bioreactor experiments; Sohil Bhavsar, Richard Rodriguez and Jay West (Bristol-Myers Squibb, Global Manufacturing and Supply) for analytical assay support. The authors would also like to thank Dmitri Smirnov and Narina Stepanova from 3M in their support for viral clearance studies.
References
- Elvin JG, Couston RG, van der Walle CF. Therapeutic antibodies: market considerations, disease targets and bioprocessing. Int J Pharm 2013; 440:83-98; PMID:22227342; http://dx.doi.org/10.1016/j.ijpharm.2011.12.039
- Reichert JM. Antibodies to watch in 2016. mAbs 2016; 8:197-204; PMID:26651519; http://dx.doi.org/10.1080/19420862.2015.1125583
- Jain E, Kumar A. Upstream processes in antibody production: evaluation of critical parameters. Biotechnol Adv 2008; 26:46-72; PMID:17920803; http://dx.doi.org/10.1016/j.biotechadv.2007.09.004
- Li F, Vijayasankaran N, Shen A, Kiss R, Amanullah A. Cell culture processes for monoclonal antibody production. mAbs 2010; 2:466-77; PMID:20622510; http://dx.doi.org/10.4161/mabs.2.5.12720
- Chon JH, Zarbis-Papastoitsis G. Advances in the production and downstream processing of antibodies. New Biotechnol 2011; 28:458-63; PMID:21515428; http://dx.doi.org/10.1016/j.nbt.2011.03.015
- Butler M, Meneses-Acosta A. Recent advances in technology supporting biopharmaceutical production from mammalian cells. Appl Microbiol Biotechnol 2012; 96:885-94; PMID:23053101; http://dx.doi.org/10.1007/s00253-012-4451-z
- Shukla AA, Thömmes J. Recent advances in large-scale production of monoclonal antibodies and related proteins. Trends Biotechnol 2010; 28:253-61; PMID:20304511; http://dx.doi.org/10.1016/j.tibtech.2010.02.001
- Gagnon P, Nian R, Lee J, Tan L, Latiff SMA, Lim CL, Chuah C, Bi X, Yang Y, Zhang W, et al. Nonspecific interactions of chromatin with immunoglobulin G and protein A, and their impact on purification performance. J Chromatogr A 2014; 1340:68-78; PMID:24661871; http://dx.doi.org/10.1016/j.chroma.2014.03.010
- Gronemeyer P, Ditz R, Strube J. Trends in upstream and downstream process development for antibody manufacturing. Bioengineering 2014; 1:188; http://dx.doi.org/10.3390/bioengineering1040188
- Kelley BDBG, Lee A. Downstream processing of monoclonal antibodies: current practices and future opportunities. Proc Scale Purificat Antibod 2009:1-23; http://dx.doi.org/10.1002/9780470444894.ch1
- Kelley B. Industrialization of mAb production technology: The bioprocessing industry at a crossroads. mAbs 2009; 1:443-52; PMID:20065641; http://dx.doi.org/10.4161/mabs.1.5.9448
- Ghosh R, Madadkar P, Wu Q. On the workings of laterally-fed membrane chromatography. J Membrane Sci 2016; 516:26-32; http://dx.doi.org/10.1016/j.memsci.2016.05.064
- Liu HF, McCooey B, Duarte T, Myers DE, Hudson T, Amanullah A, Amanullah A, van Reis R, Kelley BD. Exploration of overloaded cation exchange chromatography for monoclonal antibody purification. J Chromatogr A 2011; 1218:6943-52; PMID:21871630; http://dx.doi.org/10.1016/j.chroma.2011.08.008
- Chollangi S, Parker R, Singh N, Li Y, Borys M, Li Z. Development of robust antibody purification by optimizing protein-A chromatography in combination with precipitation methodologies. Biotechnol Bioeng 2015; 112:2292-304; PMID:25950654; http://dx.doi.org/10.1002/bit.25639
- Vunnum S, Vedantham, G, Hubbard, B., Protein A-based affinity chromatography. In Gottshalk U, ed. Process Scale Purification of Antibodies. Hoboken, NJ: John Wiley & Sons, 2009:79-102.
- Nian R, Zhang W, Tan L, Lee J, Bi X, Yang Y, Gan HT, Gagnon P. Advance chromatin extraction improves capture performance of protein A affinity chromatography. J Chromatogr A 2016; 1431:1-7; PMID:26774119; http://dx.doi.org/10.1016/j.chroma.2015.12.044
- Gan HT, Lee J, Latiff SMA, Chuah C, Toh P, Lee WY, Gagnon P. Characterization and removal of aggregates formed by nonspecific interaction of IgM monoclonal antibodies with chromatin catabolites during cell culture production. J Chromatogr A 2013; 1291:33-40; PMID:23598159; http://dx.doi.org/10.1016/j.chroma.2013.03.028
- Shukla AA, Hinckley P. Host cell protein clearance during protein A chromatography: development of an improved column wash step. Biotechnol Prog 2008; 24:1115-21; PMID:19194921; http://dx.doi.org/10.1002/btpr.50
- Levy NE, Valente KN, Choe LH, Lee KH, Lenhoff AM. Identification and characterization of host cell protein product-associated impurities in monoclonal antibody bioprocessing. Biotechnol Bioeng 2014; 111:904-12; PMID:24254318; http://dx.doi.org/10.1002/bit.25158
- Tarrant RD, Velez-Suberbie ML, Tait AS, Smales CM, Bracewell DG. Host cell protein adsorption characteristics during protein A chromatography. Biotechnol Prog 2012; 28:1037-44; PMID:22736545; http://dx.doi.org/10.1002/btpr.1581
- Nogal B, Chhiba K, Emery JC. Select host cell proteins coelute with monoclonal antibodies in protein A chromatography. Biotechnol Prog 2012; 28:454-8; PMID:22275211; http://dx.doi.org/10.1002/btpr.1514
- Sisodiya VN, Lequieu J, Rodriguez M, McDonald P, Lazzareschi KP. Studying host cell protein interactions with monoclonal antibodies using high throughput protein A chromatography. Biotechnol J 2012; 7:1233-41; PMID:22623327; http://dx.doi.org/10.1002/biot.201100479
- Hogwood CE, Bracewell DG, Smales CM. Host cell protein dynamics in recombinant CHO cells: impacts from harvest to purification and beyond. Bioengineered 2013; 4:288-91; PMID:23328085; http://dx.doi.org/10.4161/bioe.23382
- Hogwood CE, Tait AS, Koloteva-Levine N, Bracewell DG, Smales CM. The dynamics of the CHO host cell protein profile during clarification and protein A capture in a platform antibody purification process. Biotechnol Bioeng 2013; 110:240-51; PMID:22806637; http://dx.doi.org/10.1002/bit.24607
- Tait AS, Hogwood CE, Smales CM, Bracewell DG. Host cell protein dynamics in the supernatant of a mAb producing CHO cell line. Biotechnol Bioeng 2012; 109:971-82; PMID:22124969; http://dx.doi.org/10.1002/bit.24383
- Jin M, Szapiel N, Zhang J, Hickey J, Ghose S. Profiling of host cell proteins by two-dimensional difference gel electrophoresis (2D-DIGE): implications for downstream process development. Biotechnol Bioeng 2010; 105:306-16; PMID:19739084; http://dx.doi.org/10.1002/bit.22532
- Castro-Forero AA, Jokondo Z, Voloshin A. Anion-exchange chromatographic clarification: bringing simplification, robustness, and savings to MAb purification; BioProcess International 2015; 13: 52-57
- Yigzaw Y, Piper R, Tran M, Shukla AA. Exploitation of the adsorptive properties of depth filters for host cell protein removal during monoclonal antibody purification. Biotechnol Prog 2006; 22:288-96; PMID:16454522; http://dx.doi.org/10.1021/bp050274w
- Michen B, Diatta A, Fritsch J, Aneziris C, Graule T. Removal of colloidal particles in ceramic depth filters based on diatomaceous earth. Separat Purificat Technol 2011; 81:77-87; http://dx.doi.org/10.1016/j.seppur.2011.07.006
- Charlton HR, Relton JM, Slater NK. Characterization of a generic monoclonal antibody harvesting system for adsorption of DNA by depth filters and various membranes. Bioseparation 1999; 8:281-91; PMID:10786277; http://dx.doi.org/10.1023/A:1008142709419
- Schreffler J, Bailley M, Klimek T, Agneta P, Wiltsie WE, Felo M, Maisey P, Zuo X, Routhier E. Characterization of postcapture impurity removal across an adsorptive depth filter. BioProcess International 2015; 13: 45-57
- Iskra T, Bolton GR, Coffman JL, Godavarti R. The effect of protein A cycle number on the performance and lifetime of an anion exchange polishing step. Biotechnol Bioeng 2013; 110:1142-52; PMID:23138874; http://dx.doi.org/10.1002/bit.24781
- Venkiteshwaran A, Fogle J, Patnaik P, Kowle R, Chen D. Mechanistic evaluation of virus clearance by depth filtration. Biotechnol Prog 2015; 31:431-7; PMID:25683459; http://dx.doi.org/10.1002/btpr.2061
- Zhou JX, Solamo F, Hong T, Shearer M, Tressel T. Viral clearance using disposable systems in monoclonal antibody commercial downstream processing. Biotechnol Bioeng 2008; 100:488-96; PMID:18438883; http://dx.doi.org/10.1002/bit.21781
- Michen B, Meder F, Rust A, Fritsch J, Aneziris C, Graule T. Virus removal in ceramic depth filters based on diatomaceous earth. Env Sci Technol 2012; 46:1170-7; PMID:22191487; http://dx.doi.org/10.1021/es2030565
- Singh N, Arunkumar A, Chollangi S, Tan ZG, Borys M, Li ZJ. Clarification technologies for monoclonal antibody manufacturing processes: Current state and future perspectives. Biotechnol Bioeng 2016; 113:698-716; PMID:26302443; http://dx.doi.org/10.1002/bit.25810
- Zhou J, Dehghani, H. Development of viral clearance strategies for large-scle monoclonal antibody production. In Langer ES, ed. Advances in large scale biomanufacturing adn scale-up production. Rockville, MD: ASM Press/BioPlan Associates Inc., 2007:1-28.
- Shi L, Chen Q, Norling LA, Lau AS, Krejci S, Xu Y. Real time quantitative PCR as a method to evaluate xenotropic murine leukemia virus removal during pharmaceutical protein purification. Biotechnol Bioeng 2004; 87:884-96; PMID:15334415; http://dx.doi.org/10.1002/bit.20198
- Bray J, Brattle, K. Monoclonal Antibody Production: Minimizing virus safety issues. New York, NY: Plenum Publishers, 2004.
- CPMP/BWP/269/95. Note for guidance on virus validation studies. Wharf, London: Canary, 2001.
- Brorson K, Swann PG, Brown J, Wilcox B, Shapiro MA. Considerations for Developing Biopharmaceuticals: FDA Perspective. Modern Biopharmaceuticals: Wiley-VCH Verlag GmbH, 2008:1637-67.
- FDA. FDA Q5A Guidance Document: Viral Safety Evaluation of Biotechnology Products Derived from Cell Lines of Human or Animal Origin. In: Administration FaD, ed.: Federal Register, 1998.
- Lute S, Aranha H, Tremblay D, Liang D, Ackermann HW, Chu B, Moineau S, Brorson K. Characterization of coliphage PR772 and evaluation of its use for virus filter performance testing. Appl Env Microbiol 2004; 70:4864-71; PMID:15294825; http://dx.doi.org/10.1128/AEM.70.8.4864-4871.2004
- Miesegaes G, Lute S, Brorson K. Analysis of viral clearance unit operations for monoclonal antibodies. Biotechnol Bioeng 2010; 106:238-46; PMID:20073086; http://dx.doi.org/10.1002/bit.22662
- Tipton B, Boose JA, Larsen W, Beck J, O'Brien T. Retrovirus and parvovirus clearance from an affinity column product using adsoprtive depth filtration. Biopharm International 2002; 15:43-50.
- Muller D. Integrating AEX hybrid purifier enables twostage chromatography purification of recombinant therapeutic antibodies. Bio Process International Conference. Boston, MA, 2014.
- Dowd SE, Pillai SD, Wang S, Corapcioglu MY. Delineating the specific influence of virus isoelectric point and size on virus adsorption and transport through sandy soils. Appl Env Microbiol 1998; 64:405-10; PMID:9464373
- Strauss DM, Lute S, Tebaykina Z, Frey DD, Ho C, Blank GS, Brorson K, Chen Q, Yang B. Understanding the mechanism of virus removal by Q sepharose fast flow chromatography during the purification of CHO-cell derived biotherapeutics. Biotechnol Bioeng 2009; 104:371-80; PMID:19575414; http://dx.doi.org/10.1002/bit.22416
- Riordan W, Heilmann S, Brorson K, Seshadri K, He Y, Etzel M. Design of salt-tolerant membrane adsorbers for viral clearance. Biotechnol Bioeng 2009; 103:920-9; PMID:19370771; http://dx.doi.org/10.1002/bit.22314
- Riordan WT, Heilmann SM, Brorson K, Seshadri K, Etzel MR. Salt tolerant membrane adsorbers for robust impurity clearance. Biotechnol Prog 2009; 25:1695-702; PMID:19728393; http://dx.doi.org/10.1002/btpr.256
- Shukla AA, Hubbard B, Tressel T, Guhan S, Low D. Downstream processing of monoclonal antibodies—Application of platform approaches. J Chromatogr B 2007; 848:28-39; http://dx.doi.org/10.1016/j.jchromb.2006.09.026
- Kandula S, Babu S, Jin M, Shukla AA. Design of a filter train for precipitate removal in monoclonal antibody downstream processing. Biotechnol Appl Biochem 2009; 54:149-55; PMID:19656082; http://dx.doi.org/10.1042/BA20090181
- Joshi V, Shivach T, Kumar V, Yadav N, Rathore A. Avoiding antibody aggregation during processing: establishing hold times. Biotechnol J 2014; 9:1195-205; PMID:24753430; http://dx.doi.org/10.1002/biot.201400052
- Mazzer AR, Perraud X, Halley J, O'Hara J, Bracewell DG. Protein A chromatography increases monoclonal antibody aggregation rate during subsequent low pH virus inactivation hold. J Chromatogr A 2015; 1415:83-90; PMID:26346187; http://dx.doi.org/10.1016/j.chroma.2015.08.068
- Riordan W, Heilmann S, Brorson K, Seshadri K, He Y, Etzel M. Design of salt-tolerant membrane adsorbers for viral clearance. Biotechnol Bioeng 2009; 103:920-9; PMID:19370771; http://dx.doi.org/10.1002/bit.22314
- Rothbard JB, Jessop TC, Wender PA. Adaptive translocation: the role of hydrogen bonding and membrane potential in the uptake of guanidinium-rich transporters into cells. Adv Drug Del Rev 2005; 57:495-504; PMID:15722160; http://dx.doi.org/10.1016/j.addr.2004.10.003
- Lute S, Bailey M, Combs J, Sukumar M, Brorson K. Phage passage after extended processing in small-virus-retentive filters. Biotechnol Appl Biochem 2007; 48:63; http://dx.doi.org/10.1111/j.1470-8744.2007.tb00620.x