ABSTRACT
Novel biotherapeutic glycoproteins, like recombinant monoclonal antibodies (mAbs) are widely used for the treatment of numerous diseases. The N-glycans attached to the constant region of an antibody have been demonstrated to be crucial for the biological efficacy. Even minor modifications of the N-glycan structure can dictate the potency of IgG effector functions such as the antibody-dependent cell-mediated cytotoxicity (ADCC) and complement-dependent cytotoxicity (CDC).
Here, we present the development of a glycoengineered CHO-K1 host cell line (HCL), stably expressing β1,4-N-Acetylglucoseaminyltransferase III (GnT-III) and α-mannosidase II (Man-II), for the expression of a-fucosylated antibodies with enhanced Fc-mediated effector function. Glycoengineered HCLs were generated in a two-step strategy, starting with generating parental HCLs by stable transfection of CHO-K1 cells with GnT-III and Man-II. In a second step, parental HCLs were stably transfected a second time with these two transgenes to increase their copy number in the genetic background. Generated glycoengineered CHO-K1 cell lines expressing two different mAbs deliver antibody products with a content of more than 60% a-fucosylated glycans. In-depth analysis of the N-glycan structure revealed that the majority of the Fc-attached glycans of the obtained mAbs were of complex bisected type. Furthermore, we showed the efficient use of FcγRIIIa affinity chromatography as a novel method for the fast assessment of the mAbs a-fucosylation level. By testing different cultivation conditions for the pre-glycoengineered recombinant CHO-K1 clones, we identified key components essential for the production of a-fucosylated mAbs. The prevalent effect could be attributed to the trace element manganese, which leads to a strong increase of a-fucosylated complex- and hybrid-type glycans. In conclusion, the novel pre-glycoengineered CHO-K1 HCL can be used for the production of antibodies with high ratios of a-fucosylated Fc-attached N-glycans. Application of our newly developed FcγRIIIa affinity chromatography method during cell line development and use of optimized cultivation conditions can ultimately support the efficient development of a-fucosylated mAbs.
Introduction
The number of approved antibody-based therapeutics is constantly growing, and most of these are IgG1 monoclonal antibodies (mAbs). The biological activity of therapeutic IgGs is determined by two independent mechanisms: antigen recognition and Fc-mediated antibody effector functions, i.e., antibody-dependent cell-mediated cytotoxicity (ADCC) and complement-dependent cytotoxicity (CDC).Citation1-7
The N-glycans attached to the constant region (Fc) of an antibody have been demonstrated to be important for interaction of antibody with FcγRs and complement activation. The Fc-incorporated sugar is generally of the biantennary complex type, and consists of heptasaccharide comprising four N-Acetyl-Glucosamine (GlcNAc) and three mannose (Man) residues, and can be further varied by addition of galactose (Gal) and fucose (Fuc) residues as well as sialic acid (Sia, or N-acetylneuraminic acid, NANA, in human or N-glycolylneuraminic acid, NGNA, in mouse). The first GlcNAc is attached to the Asn297 of the IgG CH2 domain and might be carrying or lacking a Fuc in a α1–6 linkage. Additional variations can be introduced by attachment of bisecting GlcNAc β1–4 (). Such an N-linked oligosaccharide is referred to as a complex type oligosaccharide. In addition, two further general sugar types can be classified, namely a high-mannose or oligomannose and a hybrid type. All three types share a common trimannosyl core structure composed of pentasaccharides (GlcNAc2Man3). In the high-mannose type only mannose binds to the both non-reducing ends of the core structure. The hybrid type is characterized by presence of both high-mannose and complex structures on the either branch of the core structure.Citation8
Figure 1. Composition of a complex oligosaccharide attached to IgG Fc. A: The glycan is covalently linked to asparagine 297 of the heavy chain (EU numbering, according Kabat et al.Citation53). GlcNAc, N-acetylglucosamine; Man, mannose; bisec. GlcNAc, bisecting N-acetylglucosamine; Gal, galactose; Neu5Ac, N-acetyl-neuraminic acid; Fuc, fucose. β1,4 etc.: glycosidic bond. G0, G1, G2: complex type glycan comprising zero, one or two galactose residues added to the core structure. B: Comparison of wild-type and pre-glycoengineered N-glycosylation in CHO cells. High mannose N-glycans will be transferred from the ER to Golgi apparatus by vesicular trafficking. In wild-type CHO cells the Man-II processed glycans are linked with fucose to the proximal GlcNAc of the glycan core structure by alpha-(1,6)-fucosyltransferase 8 (FUT8). In pre-glycoengineered CHO cells the overexpression of Man-II and GnT-III, which is not expressed in wild-type CHO cells, leads to formation of bisecting GlcNAc glycans. By that, the transfer of fucose by FUT8 is substantially reduced by bisecting GlcNAc glycans. The resulting a-fucosylated N-glycans are more potent for inducing antibody-dependent cell-meditated cytotoxicity (ADCC) than fucosylated glycans.
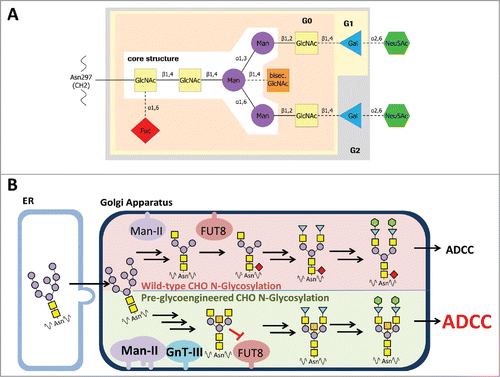
The composition of the Fc-oligosaccharide determines the affinity of the IgG to different receptors and modulates the immune response by preferential interaction with either activating or inhibitory receptor type.Citation9-12 However, in terms of clinical efficacy of therapeutic antibodies, the absence of core fucose in the Fc-attached oligosaccharide plays the most prominent role. Such IgGs show up to 50-fold higher affinity to both FcγRIIIa and FcγRIIIb and, as a result, they have increased ADCC activity.Citation2,9,10,13-15 Thus, it is not surprising that several methods have been established to alter the glycosylation profile and to generate therapeutic antibodies with improved biological functions.Citation2,3,14-17 As of late 2017, two antibodies with engineered glycans have been approved for clinical use and about 20 others have entered clinical trials.Citation3
All of the reported techniques are based on modification of the glycosylation pathway.Citation15 One of the approaches to obtain antibodies with low ratios of core fucose is production of the latter in mammalian expression cells with decreased α-1,6-fucosylation activity. For instance, Lec13, a CHO-derived cell line, was demonstrated to have a deficiency in the function of GDP-mannose 4,6-dehydratase (GMD; one of the enzymes responsible for conversion of GDP-mannose to GDP-fucose).Citation18,19 The YB2/0 rat myeloma cell line is another cell line used for the same purpose, as it was shown to have an impaired activity of α-1,6-fucosyltransferase (FUT8), which catalyzes the transfer of fucose from GDP-fucose to the GlcNAc in an α-1,6 linkage.Citation19,20 However, the unstable glycosylation profiles of these cell lines demonstrated that the partial inhibition of the activities of the respective enzymes was not sufficient for stable production of antibodies lacking core-fucose.Citation19
It was also shown that application of siRNA to silence fucosylation-involved genes resulted in production of antibodies with lower fucose levels.Citation15,21 Zhou et al. reported that antibody expression in the presence of kifunensine, an inhibitor of α-mannosidase I (Man-I), which blocks N-glycan synthesis at the Man8GlcNAc2 (Man8) or Man9GlcNAc2 (Man9) stage, resulted in production of a-fucosylated antibodies, but the majority of the generated N-glycans were of oligomannose type.Citation22
The HCL with knockout of the FUT8 enzyme, which were generated by targeted disruption of both FUT8 alleles, were demonstrated to deliver high yields of fully a-fucosylated antibodies.Citation23
Production of antibodies with increased antibody effector function was also achieved by overexpression of β1,4-N-Acetylglucoseaminyltransferase III (GnT-III) in antibody-producing cells.Citation24 GnT-III catalyzes the transfer of GlcNAc to a core mannose residue in N-linked oligosaccharides via a β1–4 linkage, which results in the formation of a bisected sugar chain. Addition of the bisecting N-acetylglucosamine takes place early in sugar maturation process, and affects central reactions of the biosynthetic pathway, i.e., prevents later addition of the core fucose by α-(1,6)-fucosyltransferase (FUT8) and blocks conversion of hybrid to complex type glycans.Citation17,25
Golgi α-mannosidase II (Man-II) is another enzyme involved in glycoengineering of therapeutic antibodies. Man-II catalyzes the removal of mannosyl linkages of GlcNAcMan5GlcNAc2 and is one of the early enzymes involved in complex oligosaccharide synthesis. Ferrara et al. show that overexpression of Man-II in addition to GnT-III delivered antibody product that is characterized by large amounts of bisected, non-fucosylated oligosaccharides.Citation13 The authors clearly obtained a high a-fucosylation ratio by this approach; however, the co-expression of the respective enzymes was achieved by transient co-transfection. Transiently transfected genetic material might get lost due to environmental factors or cell division, whereas stable expression of all proteins is necessary to retain the productivity and the level of a-fucosylated glycans over the manufacturing process.Citation26
Recent studies reported that media conditions and composition has a significant effect on glycan species produced with CHO cell systems. Cell culture conditions like temperature, pH, and dissolved oxygen have been shown to significantly alter N-glycan structure (reviewed by Hossler et al.Citation27). Low cell cultivation temperature leads to a reduction of branched structures, and sialylated glycans and decreased glycosylation were observed at low (< 6.9) and high (> 8.2) pH values.Citation28,29 Furthermore, it has been shown before that trace metals can modify glycosylation patterns when added to cell culture media. For example, addition of copper (II) chloride and manganese chloride have been shown to increase the sialic acid content and the level of Fc galactosylated species of a CHO-produced antibody, respectively.Citation30,31
In this work, a robust CHO-K1 HCL adapted to growth in suspension in chemically defined medium and developed for standard therapeutic protein expression, was engineered for production of antibodies with enhanced Fc-mediated effector functions. Furthermore, specific modifications of the cultivation conditions and media composition substantially increased the level of favored a-fucosylated hybrid glycan species. In summary, this report describes an efficient pre-glycoengineered CHO HCL glyK1M stably expressing GnT-III and Man-II and a respective suitable production system for simple and efficient cell line development for glycoengineered antibodies.
Results
Glycoengineering of CHO-K1 cells
For glycoengineering, CHO-K1 cells, adapted to growth in suspension in chemically defined medium, were stably transfected with an expression plasmid encoding for rat GnT-III (Mgat3 gene) and human Man-II (MAN2A1 gene). The expression of GnT-III was analyzed by flow cytometry in more than 300 clones that survived the puromycin-driven selection process. In most cases, we observed a fluorescence signal that was stronger than the background signal of untransfected CHO-K1 cells, which did not express the endogenous GnT-IIICitation32 (Supplement Figure S1A). As a result, 66 clones were selected to assess the Man-II expression by quantitative real-time RT-PCR. The qPCR is highly specific for the human Man-II transgene, so we observed no background signal of the endogenous Man-II that is expressed in CHO-K1 cells (Supplement Figure S1B).
Based on the fluorescence activated cell sorting (FACS) and qPCR data, clones with a high expression level of both enzymes were selected for assessment of their potential to generate antibodies with a high proportion of a-fucosylated glycans. In a first experiment, the clones were transfected transiently with an expression plasmid for a humanized antibody (mAb1) and the a-fucosylation level was determined by RP-HPLC (Supplement Figure S2A). In a second experiment, six clones (clone 17, 51, 54, 64, 255 and 260) that had shown the highest a-fucosylation in transient transfection were tested as HCL for cell line development by stable transfection with a standard antibody (MAb2) plasmid. About 60 mAb2-expressing cell lines derived from each HCL were analyzed for a-fucosylation in batch culture. Overall, all clones showed only a moderate a-fucosylation level with an average of 21 to 31%, depending on the HCL (Table I and Supplement Figure S2B). A maximum of 44% a-fucose was measured for two clones derived from HCL 64. Overall, this was considered to be insufficient for an HCL to generate manufacturing cell lines for glycoengineered antibodies.
Thus, in the next step, four of the HCLs (clones 17, 51, 64 and 260; see ) were stably transfected a second time with GnT-III and Man-II, to enhance the expression of both enzymes, and thereby to further increase their potential to generate a-fucosylated antibodies. Again, the HCL candidates were screened by FACS for GnT-III expression and by quantitative real-time RT-PCR for Man-II expression. Subsequently, 60 candidate clones were tested by transient transfection with a plasmid encoding antibody mAb2. The a-fucosylation ratio of the analyzed antibody samples was in a range of 20–56% (). Compared to the first transfection of the HCLs (20–25%), the second stable transfection with GnT-III and Man-II considerably improved the level of a-fucosylation. Eight of these HCLs, producing antibodies with a high a-fucosylation level (41–56%) and showing an adequate growth behavior, were picked for further assessment. gives an overview of the development process and the lineage of the glycoengineered HCLs.
Figure 2. Antibody a-fucosylation rate in host cell lines with GnT-III/Man-II double transfection. A: A-fucosylation level after transient transfection of glycoengineered host cell lines with mAb2 expression plasmid. Antibody was purified by protein A chromatography. The a-fucose ratio was determined by MALDI-TOF MS. The bars in grey represent the one-fold glycoengineered parental host cell lines. The corresponding HCLs that were glycoengineered a second time are depicted in black. CHO-K1 cells were used as control to determine the background of non-glycoengineered HCL (white bar). Clones selected for further evaluation are marked by asterisks. B: A-fucosylation level of mAb2 expressed in clones obtained by stable transfection of two-fold glycoengineered host cell lines. Clones derived from HCLs 17–483, 17–494 and 51–201 were cultivated in 6-well plates. Clones derived from HCLs 64–30, 64–6024, 64–6123, 260–501 and 260–1513 were cultivated in 24-well plates. Antibody was purified by protein A HPLC. The a-fucose ratio was quantified by MALDI-TOF MS.
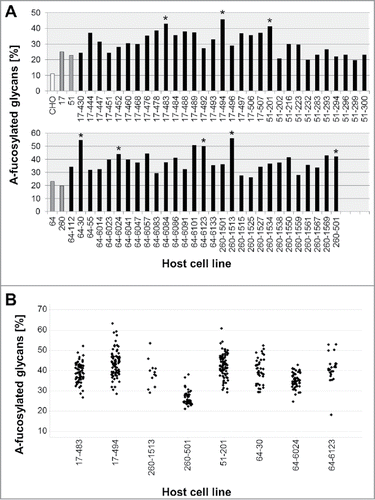
Figure 3. Overview of the development process and the lineage of the glycoengineered host cell lines. Glycoengineered HCLs were generated in a two-step strategy, starting with generating parental HCLs by stable transfection of wild-type CHO-K1 cells (highlighted by blue circle) with GnT-III and Man-II (highlighted by light orange circles). In a second step, parental HCLs were stably transfected a second time with GnT-III and Man-II (highlighted by orange circles). Generated glycoengineered CHO-K1 cell lines were subsequently used to establish stable cell lines (highlighted by green circle) for the expression of a-fucosylated mAbs. The numbers represent the clone naming as listed in .
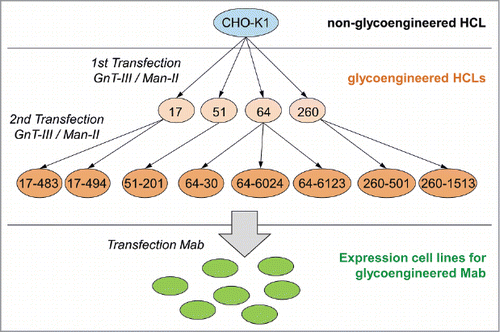
Assessment of host cell line candidates by stable transfection
Eight two-fold glycoengineered HCLs were subjected to stable transfection with a mAb2 expression vector. After a methotrexate-driven selection process, a mean of 50 antibody-expressing cell lines derived from each HCL were expanded to 6- or 24-well plates to analyze the glycosylation by matrix-assisted laser desorption ionization-time of flight mass spectrometry (MALDI-TOF MS) (, Table I). On average, the clones showed an a-fucosylation level between 26 and 44%, depending on the HCL, with a maximum of 63% obtained by a HCL 17-494-derived clone. Compared to the parental HCLs, the second glycoengineering step led to a significant, up to 2.1-fold increase of a-fucosylation observed with HCL-derived antibody-expressing cell lines.
Next, we performed additional glyco-structure analysis of the mAb2 expressed in the selected cell lines. The addressed elements of Fc-attached oligosaccharide included presence/absence of fucose and bisecting GlcNAc, high mannose, hybrid and complex type glycans. The comparative analysis of the glycopattern obtained by expression in pre-glycoengineered cell lines demonstrated, in general, comparable amounts of the glycosylation structures (). As expected for co-expression with GnT-III and Man-II, the majority of the glycans (up to 67%) was represented by complex bisected sugar structures, whereas only small amounts of hybrid or non-bisected (WT) complex glycans were detected. However, we still observed differences in glycosylation pattern, which enabled further ranking of the HCLs. Clone 17–494 was selected as the first candidate for the glycoengineered HCL, generating mAb2 expression cell lines with a-fucose ratio up to 63%. Clone 51–201 demonstrated the second highest a-fucosylation degree, but had a notably enhanced high mannose ratio of 17%, on average, and was not considered further. Therefore, clone 64–30 was chosen as a second candidate for further assessment.
Figure 4. Comparison of glycosylation pattern of antibodies produced in pre-glycoengineered host cell lines. A: Average glycan type distribution. Eight glyco-engineered host cell lines were stably transfected with mAb2 antibody genes. On average, antibody oligosaccharide structures from 49 clones per HCL were analyzed by MALDI-TOF MS and classified into high mannose, hybrid type, non-bisected complex (WT) or bisected complex type glycans. A-fuc, sum of a-fucosylated glycans. Error bars represent standard deviation according to the number of clones tested (as listed in ). B: Stable transfection of HCL 17–494 or 64–30 with mAb3, or co-transfection with mAb3, GnT-III and Man-II (G/M). Glycan composition of 21 to 24 clones per transfection was analyzed by MALDI-TOF MS to calculate the average distribution and a-fucosylation rate. Error bars represent standard deviation according to the number of clones tested (as listed in ).
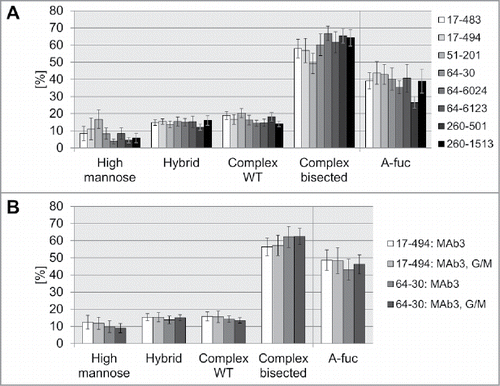
For further examination, the HCL 17–494 and 64–30 were subjected to stable transfection with a different antibody, mAb3. In parallel, we performed a co-transfection of the respective HCLs with GnT-III and Man-II (in addition to mAb3) to evaluate if the a-fucosylation can be further enhanced by overexpression of the enzymes. After the selection procedure, 21 to 24 clones per transfection were expanded into shaken 6-well plates to perform a batch culture, followed by glycopattern analysis by MALDI-TOF MS.
As observed with mAb2, HCL 17–494-derived clones demonstrated a higher a-fucosylation ratio compared to HCL 64-30-derived clones (, ). The co-transfection with GnT-III and Man-II led to only a slight increase of a-fucosylation for HCL 64-30. No increase could be observed for HCL 17-494, demonstrating that mere transfection with antibody plasmid is sufficient for production of antibodies with high a-fucose ratios. Analysis of the obtained glycostructures demonstrated that HCL 17-494-derived clones showed slightly higher percentage of high-mannose glycans than HCL 64–30 clones in the 6-well batch culture (). The co-transfection with GnT-III and Man-II did not significantly influence glycopattern of the two tested cell lines.
Table 1. A-fucosylation of antibody expression cell lines derived from stable transfection of glycoengineered host cell lines. The host cell lines were transfected with a standard plasmid used for development of expression cell lines for a human antibody (mAb2 or mAb3), or co-transfected with an antibody and a GnT-III/Man-II expression plasmid. The antibody plasmid also contained the DHFR gene, and methotrexate was added to the medium as selective agent. After about three weeks of clone selection, the plates were screened for antibody expression by ELISA. Antibody expressing clones were further expanded into shaken 24-well or 6-well plates to perform a batch culture with a defined cell count at start. The antibody was purified from the cell culture supernatant after 7 days by protein A HPLC. The proportion of a-fucosylated glycans was measured by RP-HPLC or MALDI-TOF MS.
Characterization of glycoengineered host cell lines
Next, we assessed the stability of GnT-III and Man-II expression in the two candidates for glycoengineered HCL, clone 17–494 and clone 64–30. The HCLs were therefore permanently cultivated for >160 days to reflect the time period for cell line development from transfection to fermentation. Over this period, viable cells were cryo-preserved periodically and, finally, thawed and recultivated in parallel to analyze expression stability of the respective enzymes. We performed intracellular flow cytometry to assess the expression level of both enzymes, and qPCR to determine the copy numbers of the GnT-III and Man-II transgenes in the genome over the respective period of time ().
Figure 5. Characterization of transgene stability. A: GnT-III expression for each HCL was determined by flow cytometry after intracellular staining. Each time point was measured in triplicates. Relative GEO Mean: Geometric mean fluorescence intensity normalized to 100% at day zero. B: Man-II expression assessment by flow cytometry as described for GnT-III (A). C: Copy number determination at day zero and after 180 days (HCL 17–494) or 134 days (HCL 60–30) by qPCR. Error bars represent standard deviation.
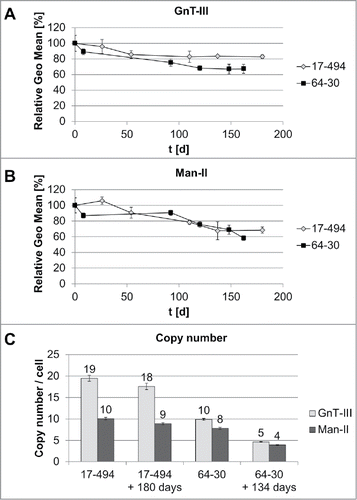
The flow cytometry analysis of the HCL 17–494 demonstrated a slight decrease of protein expression from 100% to 86% after 50 days of cultivation and remained at that level for 130 more days for GnT-III, whereas expression of Man-II dropped to 68%, compared to the initial level during similar period of time. The HCL 64–30 demonstrated less stable expression of both enzymes with a decrease in expression level to 67% for GnT-III and to 58% for Man-II after 162 days in culture.
For HCL 17–494, we observed nearly double the number of copies of GnT-III (19 per cell) compared to Man-II (10 per cell), which remained mostly unaffected after 180 days. The HCL 64–30 appeared to carry comparable numbers for both enzymes, with 10 and 8 copies per cell for GnT-III and Man-II, respectively. These numbers decreased by half after 134 days.
The obtained results, along with a fast and robust growth behavior and an easy transfection procedure, make HCL 17–494 a suitable candidate for a pre-glycoengineered HCL.
FcγRIII-HPLC for high throughput clone screening
FcγRIIIa affinity chromatography is a novel tool enabling separation of antibodies by their a-fucose content. MALDI-TOF MS analysis, applied here, quantifies the absolute percentage of isolated N-glycans that are not fucosylated, and does not discriminate between mono- and bi-a-fucosylated antibody samples. The application of FcγRIIIa affinity chromatography enables the determination of the relative biologic activity (RBA) of an antibody pool, as this method allows assessment of total percentage of the antibody species with improved effector functions.
Here, we compared analysis of the a-fucose levels of mAb2 samples from stable transfection in three pre-glycoengineered HCLs by the FcγRIIIa(V158) affinity column, to the percentage of a-fucosylation determined by MALDI TOF MS.
The comparative analysis of the a-fucose ratio of the 222 mAb2 samples revealed a high correlation of the results obtained by the two methods (). The percentage of a-fucosylated antibodies determined by FcγRIIIa chromatography, which reflects the RBA of the antibody pool, is higher than one measured by MS, as it comprises both mono- and bi-a-fucosylated immunoglobulins. On average, an a-fucosylation rate of greater than 60% measured by MS corresponds to a relative biological activity of greater than 90% (as achieved with HCL 17–494 for two different antibodies). These results demonstrated that FcγRIIIa affinity chromatography is a fast and efficient method for cell line development that enables the detection of clones with high a-fucose levels in a high throughput manner.
Figure 6. Comparison of MALDI TOF MS and FcγRIIIa chromatography as two different methods to analyze the a-fucosylation degree of antibodies. Batch culture samples from 69 mAb2 expressing clones derived from HCL 17–483, 73 samples from HCL 17–494 and 80 samples from HCL 51–201 were analyzed by MALDI-TOF MS to determine the percentage of a-fucosylated glycans (A-fuc). In parallel, the relative biological activity (RBA) was measured by FcγRIIIa affinity chromatography. Linear regression analysis was performed excluding two outliers (triangles).
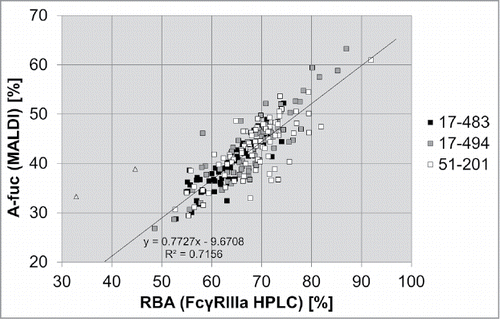
Media and process dictates the level of desired a-fucosylated glycan types
Media composition and process parameters have been shown to influence the structure of generic N-glycans expressed in mammalian cell cultures.Citation27 Particularly, the effect of trace elements, like manganese, on N-glycosylation site-occupancy and glycan structure of recombinant glycoproteins expressed in CHO cells has been previously described.Citation33,34 Copper is another important cofactor used in CHO cultivations used for modulation of amidation levels of the carboxyl terminal of immunoglobulins.Citation35 Therefore, we employed FcγRIIIa affinity chromatography to address the influence of essential medium trace elements manganese and copper on the level of mAb3 a-fucosylation for established glyK1M CHO cell lines. We used three different glycoengineered clones (clone 1, clone 2 and clone 3), all expressing mAb3 in a protein-free and chemically-defined cultivation medium A (CDM-A) background for a subsequent concentration screen of the metals in a production-like fed-batch experiment (). Interestingly, high levels of manganese showed the largest, and a consistent and clone-independent, positive effect for the formation of a-fucosylated glycan species for all three clones (up to 1.5 to 1.7-fold increase of a-fucosylated species) (, Supplement Figure S3). No differences in the RBA analysis were detected for the tested copper concentrations. Since manganese has the highest impact on the a-fucose level for mAb3, we tested in a following batch cultivation experiment six recombinant glyK1M clones expressing the same mAb3, but originating from different HCLs 17–494 and 64–30 to analyze the general relevance (). By that, all tested clones showed a significant increase in IgG a-fucosylation measured by FcγRIIIa affinity chromatography (10–51% increase). In-depth MS analytics of the respective glycan species generated with manganese supplementation revealed a specific increase of primarily complex-type (3-fold increase), but also a-fucosylated hybrid N-glycans (2-fold increase) and no impact on high mannose species ().
Figure 7. Influence of medium trace elements on a-fucosylation degree of antibodies. Different concentrations for manganese, copper, and iron in a chemically-defined medium A (CDM-A) were used for cultivation experiments with preglycoengineerd CHO cells all expressing the same mAb3 (A). The relative biological activity (RBA, measured by FcγRIIIa chromatography) for mAb3 produced with clone 1 in a 14-day fed-batch cultivation with CDM-A variants A1 to A8 is shown in (B). Six mAb3 expressing clones, clone 1, clone 2, clone 3 - HCL 17–494 based, clone 4, clone 5, clone 6 - HCL 64–30 (as listed in ) based were cultivated in a seven day batch process with low (A1) and high (A2) manganese levels. The RBA is shown in (C). The different glycan species of mAb3 produced with clone 1 in a 14-day fed-batch cultivation with CDM-A variants A1 to A8 are shown in (D).
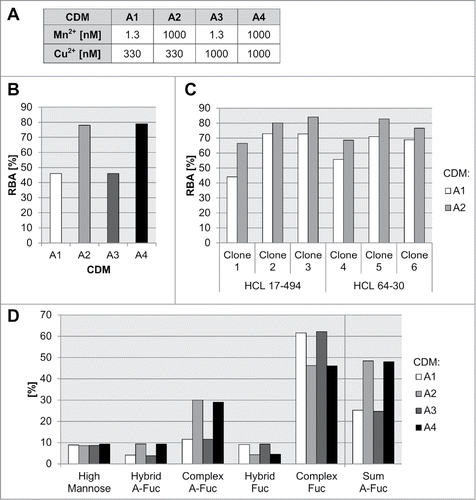
Next, we aimed to identify optimized process parameters for increased a-fucosylated IgG production by testing the influence of two different media backgrounds, medium osmolality, and cultivation temperature in combination with different levels of manganese (). For that, clones 1, 2 and 3, all expressing the same mAb3 used in the previous experiment, were cultivated as fed-batch cultures in shaker flasks in two chemically defined medium variants (CDM-A and CDM-B). For clone 1 and clone 2, CDM-B caused a 2- to 3-fold increase in IgG levels compared to CDM-A background (). In general, the a-fucosylation ratio did not correlate with the antibody titer. Supplementation with manganese had no influence on the final titer of the fed-batch assays, although the same high levels of manganese in CDM-A and CDM-B led to comparable abundance of cumulative a-fucosylated glycans (). As shown above, the addition of manganese leads to a strong increase of a-fucosylated complex- and hybrid-type glycans, and it has no influence on the amount of high mannose type glycans (). Slightly higher levels of a-fucosylated complex-type glycans were found for clone 1 and clone 3 produced with CDM-B. The effect seems to be specific for glycoengineered recombinant CHO cell lines since addition of manganese to the culture medium of recombinant wild-type CHO cell lines, clone 7 and clone 8, expressing mAb4 and mAb5, respectively, has no effect on the level of a-fucosylated glycan species (figure S4).
Figure 8. Influence of medium and process parameters on titer and different a-fucosylated glycan classes. A: Three mAb3 expressing clones (clone 1 to 3) were cultivated in a fed-batch process using different basal media, manganese concentrations, base media osmolality levels, and cultivation temperature. For selected tested cultivation and medium conditions the titer (B), the sum of a-fucosylated IgGs (C), sum of complex a-fucosylated IgGs (D), sum of hybrid a-fucosylated IgGs (E), and sum of high mannose IgGs (F) were analyzed. The sum of complex, hybrid and mannose species was quantified by an appropriate MS method.
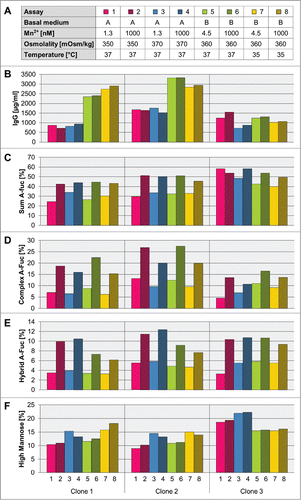
Both media variants differ in their osmolality of the basal medium: CDM-A has a lower osmolality than CDM-B. Therefore, we tested the relevance of osmolality on titer and glycan species formation using the CDM-A matrix. Except for clone 3 the medium osmolality has no effect on the titer level. Yet, all three clones showed an increased amount of high mannose and a tendency toward less a-fucosylated complex-type glycan species in manganese-supplemented cultures (, ).
An additional temperature shift to 35°C during cultivation in CDM-B background, had no clear effect on overall product titer in the tested set-up. All three clones showed a clear sensitivity for a temperature shift in glycan formation, which leads to higher mannose and less a-fucosylated complex- and hybrid-type glycan compared to a culture without changing temperature ().
Recently, it was shown that glycan species profile of an expressed generic mAb by CHO cells can change during fed-batch production processes.Citation36 The dynamic of glycan profile for pre-glycoengineered CHO cells has not been reported so far. Therefore, we used clone 5, originating from 2x glycoengineered HCL 64–30 (), with identified high RBA levels for mAb3 and a CDM-B-based 2 L production-like small-scale model to elucidate the glycan species dynamic throughout a 14-day fed-batch process. As reported previously by Reusch et al., the galactosylated glycan species of mAb3 decreased and was even extinct until day 14 of the production process ().Citation36 On the other hand, the sum of a-fucosylated glycans, as well as high mannose, complex- and hybrid-type species, remained equal throughout the process (). Also, the sum of glycan species with bisecting GlcNAc, catalyzed with heterologous expressed GnT-III, did not change over the process ().
Table 2. Origin of recombinant clones used for cultivation experiments. The recombinant clones expressing a human antibody (mAb3, mAb4 or mAb5) were generated using either the wild-type CHO-K1 host cell line or glycoengineered host cell lines 17–494 or 64–30.
Figure 9. Dynamic of glycan species profile during a fed-batch process. A mAb3 expressing clone 5 was cultivated in a 14-day fed-batch process using media CDM-B and respective feeds. A: The glycan species abundance at different process timepoints, measured by RP-HPLC, is shown for sum of a-fucosylated (A-fuc) IgGs, sum of high mannose IgGs, sum of a-fucosylated complex-type IgGs, and sum of a-fucosylated hybrid-type IgG. B: The abundance of galactosylated IgGs and sum of GlcNAc-bisecting glycan species is shown.
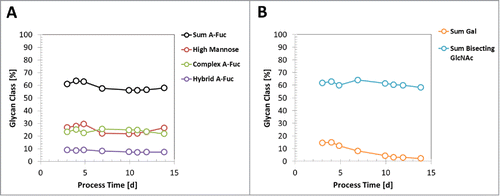
Discussion
The composition of the Fc-attached glycan is known to be important for therapeutic antibodies to elicit their effector functions.Citation9,10,12,37-39 The absence of core fucose plays the most important role in the design of antibodies engineered to have enhanced ADCC activity. Antibodies lacking core fucose residues have an up to 50-fold higher affinity to FcγRIIIa and FcγRIIIb, which leads to an increased ADCC.Citation3,8,13,14,16-18,40,41 Therefore, numerous techniques and methods have been developed to achieve production of antibody therapeutics with low fucosylation levels. All of the existing strategies are aimed to block one of the fucosylation relevant enzymes.Citation15,21,22,40,41,42 However, a FUT8-knockout cell line with moderate productivity is currently the only stable HCL for production of completely a-fucosylated antibodies.Citation19,23
In this work, we generated a pre-glycoengineered HCL, stably expressing GnT-III and Man-II, which delivers high titers of antibody products with an a-fucose content of more than 60%, as demonstrated for at least two different mAbs. To achieve this goal, it was necessary to stably transfect the CHO-K1 cells two times with both glycosylation enzymes. Pre-glycoengineered HCLs from the first round already showed a high percentage of a-fucosylation in transient transfection with an antibody plasmid. But this could not be attained with antibody-expressing clones derived from stable transfection of these HCLs. Thus, the HCLs had to be transfected a second time with GnT-III and Man-II to further increase their potential for a-fucosylated antibodies. A-fucose maxima of up to 67% were now observed with the best two HCLs, confirming the improving effect of the second transfection of the HCLs with GnT-III and Man-II. The a-fucosylation could not be further increased by overexpression of the two enzymes: A cotransfection of the (2-fold glycoengineered) HCLs with antibody plus additional GnT-III/ManII had no or only marginal effect on the a-fucose level. A possible limit might rather depend on the cultivation parameters of the cell lines, like medium composition or culture conditions, than on lack of the two enzymes.
The expression of large amounts of antibodies with high mannose glycans was reported to be critical for the Fc-mediated effector functions and the half-life of the given antibody.Citation8,43 In addition, efficient performance of GnT-III and Man-II is characterized by the absence of the high mannose sugar structure and by the shift to expression of bi-sected, a-fucosylated, complex sugar type antibodies.Citation13 To assess this, we performed a more detailed analysis of the glycopattern of antibody samples by mass spectrometry. The glyco-analysis revealed that the majority of the Fc-attached glycans of the obtained IgGs was of complex bisected type, as is characteristic for the efficient performance of the GnT-III and Man-II.Citation13
The subsequently performed copy number determination demonstrated nearly no loss of both transgenes in HCL 17–494 over 180 days. However, unstable expression can be caused by copy loss, but also by epigenetic transgene silencing.Citation44-47 In fact, we determined a slight decrease of GnT-III expression (from 100% to 83%) by flow cytometry, whereas expression of Man-II dropped to 68% over 180 days. Currently, we cannot rule out that this moderate decrease of GnT-III/Man-II expression can have a negative influence on a-fucosylation during the development process of an antibody production cell line. Thus, although a co-transfection of HCL 17–494 with antibody plus GnT-III/Man-II showed no additional effect on a-fucosylation in our experiments, it might be helpful to avoid glycan pattern changes and reduction of antibody effector function over the process time.
Taken together, the generated HCL 17–494, was characterized by robust delivery of high antibody yields with low fucose levels. Moreover, this glycoengineered HCL has morphological properties, growth behavior and expression yields similar to those of the parental CHO-K1 cell line. Hence, the expression of therapeutic proteins can be carried out on an already established platform to generate manufacturing cell lines for non-glycoengineered antibodies.
We also demonstrated application of FcγRIIIa affinity chromatography, which is a novel method for the assessment of a-fucosylation of an antibody pool. The FcγRIIIa affinity chromatography was employed here in a comparative to MS-analysis manner. Our results showed a high correlation of the two methods, and proved that this technique can be used in the development process as a fast and efficient tool for ranking of cell lines producing a-fucosylated antibodies. This enables high-throughput screening of hundreds of clones at an early timepoint during the cell line development process. Thus, in combination with productivity screening, it simplifies and accelerates the identification of manufacturing cell lines producing antibodies with high effector function.
Next, we employed the FcγRIIIa affinity chromatography to assess a-fucose ratio of the antibodies produced in the same HCL under various medium conditions. The results revealed a clear impact of the applied medium on the percentage of the a-fucosylated antibodies. This finding indicates that modulation of cultivation conditions, such as temperature, osmolality, composition of medium per se, and addition of different ions/additives, might further increase the a-fucose levels of the antibodies produced by glycoengineered HCL, although this requires further study. Such changes in culture conditions might not only affect the a-fucose ratio, but also the overall glycan composition. Thus, FcγRIIIa affinity chromatography cannot replace the detailed glycan pattern analysis by MS or RP-HPLC that allow the detection of all oligosaccharide structures and their classification into different glycan types.
The mammalian N-glycosylation pathway is a highly spatial and temporal regulated post-translational modification machinery, and even minor variations of, for example, cultivationconditions may have a significant impact on the final glycan structure.Citation27 Trace elements, like manganese, have been discussed as glycan modulators of recombinant glycoproteins expressed in CHO cells before.Citation33,34 In our cultivation experiments we have observed a positive effect of manganese supplementation to increase the level of a-fucosylated glycan species. Almost all N-glycosylation relevant enzymes are metalloenzymes and require manganese for full activity. The substantial impact of manganese supplementation for the production of bisecting a-fucosylated mAbs, may be due to the manganese-dependency of the GnT-III enzyme.Citation25 On the other hand, the analysis of glycan dynamics during a 14-day fed-batch process suggested that, compared to galactosylated glycan species, the process time has no effect on the a-fucosylated N-glycan profile. Yet, more studies are needed to identify the rate-limiting enzymatic step that utilizes manganese in the generation of a-fucosylated mAbs.
In general, high levels of high mannose should be avoided due to a greater risk for altering the antibody effector function and faster clearance in vivo.Citation48,49 A previous study has shown the influence of cell culture medium osmolality and the level of high mannose glycan species.Citation50 In line with the former study, we observed a clear trend to more high mannose and less a-fucosylated complex-type glycans by using a high osmolality version of medium CDM-A. This observation suggests cultivation media with low osmolality should be used for the production of antibodies with the favored a-fucosylated complex type glycans from pre-glycoengineered CHO HCLs.
In summary, the development of a pre-glycoengineered HCL as presented here is an efficient and reliable strategy for the production of antibodies with high ratios of a-fucosylated Fc-attached N-glycans. The innovative FcγRIIIa affinity chromatography method can be applied for the high content and throughput assessment of a-fucosylation levels of mAbs, especially clone screening and development. Finally, we have shown that manganese is a key media component vital for the production of a-fucosylated mAbs from our novel pre-glycoengineered CHO HCLs.
Material and methods
Glycoengineering of CHO-K1 cells
Construction of GnT-III/Man-II expression vectors
The rat GnT-III gene was amplified by polymerase chain reaction (PCR) from a rat kidney cDNA library (BD Biosciences). The gene coding for Man-II was amplified from human DNA using gene specific primers. Both genes were combined with chimeric MPSV promoter and subcloned into expression vector. The vector was derived from pUC18 (Thermo Fisher Scientific) by inserting either the puromycin resistance gene or hygromycin resistance gene and the scaffold-attachment region (SAR) for enhanced expression.
Stable transfection
CHO-K1 cells were transfected by nucleofection (Amaxa) with the GnT-III/Man-II expression vector containing puromycin resistance gene as a selection marker. Starting two days after transfection, the cells were cultivated in chemically defined medium with 9 µg/ml puromycin to generate puromycin-resistant, glycoengineered HCLs. Glycoengineered HCLs were transfected a second time with the GnT-III/Man-II expression vector containing hygromycin resistance gene. The cells were cultivated in chemically defined medium with 9 µg/ml puromycin and 600 µg/ml hygromycin B. Clones were expanded from 384-well plate to shaker flask format. Single cell cloning was performed by automated limiting dilution into 384-well plates. For cryopreservation, selected clones were frozen with 1 × 107 cell/ml in chemically defined medium containing 7.5% dimethyl sulfoxide. Monoclonal clones expressing mAb3 were generated using either HCL 17–494 for clone 1, clone 2, clone 3 or HCL 64–30 for clone 4, clone 5, and clone 6. Two non-glycoengineered recombinant CHO-K1 clones, clone 7 and clone 8, expressing mAb4 (IgG1) and mAb5 (IgG4), respectively, were used for testing the effect of manganese addition to proprietary medium CDM-A on a-fucosylated glycan levels.
Cell cultivation
Recombinant glycoengineered CHO-K1 clones stably expressing mAbs were grown in shake flasks and a 2 L small-scale bioreactor (Sartorius Stedim) system using standard eukaryotic cultivation conditions. Batch and fed-batch experiments were performed using proprietary media CDM-A or CDM-B and respective feeds. Cells can also be cultivated in CD-CHO medium (Thermo Fisher Scientific). Product titers were quantified by a PorosA HPLC method as described previously.Citation51
Flow cytometry
Relative rat GnT-III expression of cells was evaluated by flow cytometry using rabbit anti-GnT-III serum, derived from an in-house immunization campaign. For intracellular staining, cells were washed, fixed with 4% paraformaldehyde (Sigma-Aldrich), permeabilized using 1% saponin (Sigma-Aldrich) in phosphate-buffered saline (PBS), and then incubated with rabbit anti-GnT-III serum for 30 min at 4°C. Cells were rinsed with PBS and incubated for 30 min at 4°C with an FITC-conjugated goat-anti-rabbit antibody (Sigma-Aldrich, F6005), washed three times and resuspended in PBS for flow cytometry analysis.
For analyzing the expression stability of GnT-III and Man-II, living cells were fixed and permeabilized using BD Cytofix/Cytoperm kit (BD Bioscience) and then incubated for 30 min at 4°C with sheep anti-GnT-III, or sheep anti-Man-II serum (both derived from in-house immunization campaigns), respectively. Cells were washed and incubated for 30 min at 4°C with Alexa Fluor 488 Donkey Anti-Sheep IgG (H+L) (Molecular Probes, A11015), washed and resuspended in PBS with 2% Ultra Low IgG FCS (Gibco) for flow cytometer analysis.
Quantitative real-time RT-PCR
The relative amount of Man-II mRNA was determined by quantitative, real-time, one-step RT-PCR using FastLane Cell RT-PCR Kit (Qiagen) with a LightCycler 480 (Roche) and Man-II specific primers as follows: GAB5625 (5′-GTCGTTATGGGAATGAGGCACCACAAAG-3′) and GAB1084 (5′-CTCACAATTATCCCTCTCAGTTGACACTGC-3′). After initial incubations at 50°C for 20 min and 95°C for 10 min, 40 cycles of amplification were carried out for 15 s at 95°C, followed by 30 s at 56°C. The amplification product was normalized to that of human GAPDH.
Transgene copy number determination
The copy number of the transgenes GnT-III or Man-II were determined by quantitative real-time PCR as described previously,Citation46 using primers as follows: GnT-III forward primer 158 (5′- TGAACACATGTATGCTCCTAAG-3′), reverse primer 159 (5′-AGGTCCTCTTCAGAGATCAG-3′), probe 165 (5′-[FAM]-AAATCCCTACCGGGAGCCCAAGAG-[BHQ1]-3′); Man-II forward primer 162 (5′- GCCTTGAGCAAGGTATCCAG-3′), reverse primer 163 (5′-GCAGCTCAAGGGTAGGTGAG-3′), probe 167 (5′-[Cy5]-TCGGTCAGTTATCCTTCTCTCCTTAGCC-[BHQ3]-3′). Genomic DNA and standards were tested in quadruplicates.
Transient and stable transfection with antibody plasmid
Glycoengineered HCLs were transfected by nucleofection with a plasmid containing a human IgG heavy chain, a human IgG light chain and a murine dihydrofolate reductase (DHFR) expression cassette. For transient expression of the antibody, the transfected cells were cultivated in chemically defined medium in shaken 6-well plates. Supernatant was harvested seven days after transfection for further analysis.
For generating stably transfected antibody expressing cell lines, the HCLs were cultivated in chemically defined medium containing 5 µg/ml puromycin and 300 µg/ml hygromycin B for transfection. Two days after transfection, 350 to 600 nM methotrexate was added to the medium as selective agent. After three weeks, stably transfected clones were further expanded in medium with 5 µg/ml puromycin, 300 µg/ml hygromycin B and 250 nM methotrexate. Final recombinant candidate clones were further processed by limited dilution to ensure clonality. For antibody analysis, the clones were cultivated for one week in shaken 6-well or 24-well plates.
Glycan analysis
MALDI-TOF MS analysis of carbohydrate composition
Generation of Fc fragments from human IgG
To generate Fc-fragments, the antibodies were incubated for 72 hours at 25°C in 50 mM Tris pH 8.0, 150 mM NaCl with 0.42 U plasmin (Roche, Switzerland) per mg. Fc fragments were subsequently isolated by Protein A beads, GE Healthcare) washed with 50 mM Tris pH 8.0, 100 mM glycine, 150 mM NaCl). Elution was carried out with 50 mM Tris pH 3.0, 100 mM glycine, 150 mM NaCl. The eluate was neutralized by adding 1:40 v/v 2 M Tris pH 8.0 and loaded on a SEC column Superdex S200 10/300 GL (GE Healthcare). Samples were concentrated with the exchange of buffer to 20 mM Tris pH 8.0 (Amicon, Millipore).
Cleavage of N-linked oligosaccharides from human Fc
The N-linked oligosaccharides were cleaved from 1 mg of Fc-fragments by incubation with 0.005U of PNGase F (QA-Bio, E-PNG01). Additionally, carbohydrates were cleaved by Endo S (Genovis, IgGZERO), applied in a molar ratio 1:20, and combined with 0.1 U/mg Endo H (QA-Bio, E-EH02). All reactions were carried out in 20 mM Tris pH 8.0 at 37°C for 16 hours.
Cleavage of N-linked oligosaccharides from a full size human IgG
To cleave carbohydrates from full-length human IgG, the samples were transferred in 20 mM Tris pH 8.0 (Amicon 5.000 MWCO, Millipore) and incubated with Endo S in a molar ratio of 1:7 combined with 0.1 U/mg Endo H at 37°C for 16 hours.
Carboxypeptidase B treatment
To remove heterogeneity caused by C-terminal lysine, samples were further treated with Carboxypeptidase B (Roche, 03358682103; 1 mg/ml). Subsequently, 1 µl Carboxypeptidase B per 50 µg protein was added to the Endoglycosidase reaction and incubated again for 1 hour at 37°C. After digestion, the samples were purified by Protein A (POROS A, Applied Biosystems) and neutralized with 1:40 v/v 2M Tris pH 8.0.
MALDI-TOF analysis
Neutral oligosaccharide profiles for the human IgGs were analyzed by mass spectrometry (Autoflex, Bruker Daltonics GmbH) in positive ion mode as described previously.Citation52
Reversed phase chromatography
The content of a-fucosylated N-glycans was determined by glycan release with PNGaseF, followed by derivatization with 2-aminobenzamidine and separation by reversed-phase ultra-performance liquid chromatography (UPLC). 200 µg of protein sample were buffer-exchanged in 20 mM ammonium bicarbonate pH 7.8 at a final volume of 48 µL, before 2 µL of PNGase F (New England Biolabs, P0705L; 500000 U/mL) were added and the reaction mixture was incubated at 45°C for 1 hour. Released glycans were collected in the flow-through by ultracentrifugation in a NanoSep centrifugal device with 10 kD cutoff (Pall Life Sciences). 5 µL of 200 mM acetic acid were added and the mixture was incubated at 45°C for 15 minutes. 2-AB labeling of the released glycans was performed with the GlycoProfile 2-AB labeling kit (Sigma) according to the manufacturer's instructions. Post-labeling cleanup was performed on HyperSep-96 diol cartridges (Thermo Scientific) according to the manufacturer's instructions. Labeled glycans (approximately 130 pmol) were applied on an Acquity HSS T3 column (2.1 × 150 mm, 1.8 µm bead size) (Thermo Scientific), pre-equilibrated with buffer A (0.1% aqueous formic acid) at a flow rate of 0.3 mL/min at 80°C and separated by a linear gradient of 3% to 8.5% buffer B (0.1% formic acid in acetonitrile) over 8 minutes. Glycans were detected by 2-AB fluorescence with an excitation and emission wavelength of 362 and 422 nm, respectively. This method separates non-fucosylated glycans (retention time between 4.1 and 6.4 minutes) from fucosylated glycans (retention time between 6.4 and 7.1 minutes).
Quantification of relative biological activity by FcγRIIIa affinity chromatography
Biotinylated human FcγRIIIa_V158 was incubated with streptavidin sepharose for 2 hours at mild shaking. The receptor derivatized sepharose was packed in a 1 ml XK column, (GE Healthcare). Subsequently, the affinity column was equilibrated with 20 mM sodium citrate, 150 mM NaCl pH 6.0 at flow rate of 0.5 ml/min using a Dionex Summit system. The antibody samples containing 30 to 50 µg in equilibration buffer were loaded onto the column, washed with 2.5 column volumes (CV) of equilibration buffer and eluted with a linear pH gradient of 30 CV with 20 mM Citrate, 150 mM NaCl pH 3.0. The experiments were carried out at room temperature. The elution profile was obtained by continuous measurement of the absorbance at 280 nm. The under curve areas of both peaks were determined by integration and the percentage of the eluted peaks over the total area were recorded.
Abbreviations
2-AB | = | 2-aminobenzamide |
ADCC | = | antibody-dependent cell-mediated cytotoxicity |
CDC | = | complement-dependent cytotoxicity |
CDM | = | chemically defined cultivation medium |
CH2 | = | constant domain of antibody heavy chain 2 |
CHO | = | Chinese hamster ovary |
CV | = | column volumes |
DHFR | = | dihydrofolate reductase |
Endo H | = | Endoglycosidase H |
Endo S | = | Endoglycosidase S |
FACS | = | fluorescence-activated cell sorting |
Fc | = | constant region of an antibody |
FITC | = | Fluorescein isothiocyanate |
Fuc | = | fucose |
FUT8 | = | 1,6-fucosyltransferase |
GlcNAc | = | N-Acetyl-Glucosamine |
GMD | = | GDP-mannose 4,6-dehydratase |
GnT-III | = | β1,4-N-Acetylglucoseaminyltransferase III |
HCL | = | host cell line |
IgGs | = | immunoglobulin G |
mAbs | = | monoclonal antibodies |
MALDI-TOF MS | = | matrix-assisted laser desorption ionization-time of flight mass spectrometry |
Man | = | mannose |
Man-I | = | α-mannosidase I |
Man-II | = | α-mannosidase II |
NANA | = | N-acetylneuraminic acid |
NGNA | = | N-glycolylneuraminic acid |
PNGase F | = | peptide-N4-(N-acetyl-beta-glucosaminyl) asparagine amidase |
qPCR | = | quantitative polymerase chain reaction |
RBA | = | relative biologic activity |
RP-HPLC | = | reverse phase-high performance liquid chromatography |
Sia | = | sialic acid |
UPLC | = | ultra-performance liquid chromatography |
WT | = | wild-type |
Disclosure of potential conflicts of interest
No potential conflicts of interest were disclosed.
suppl_mat.zip
Download Zip (7.5 MB)Acknowledgments
The authors thank Annett Kaeske, Inga Kranz, Carina Post, Beatrix Rauch, Christina Reichle, Heidi Reindl, Tea Rodriguez Diaz and Sabrina Vetter for brilliant contributions to the development of the cell lines. We also thank Petra Hausknecht and Anna Mayr for excellent conduction of cell culture experiments, and Eva Greiter and Markus Haberger for valuable glycan analysis. And we thank Tetyana Dashivets for critical review of the manuscript.
References
- Jefferis R. Isotype and glycoform selection for antibody therapeutics. Arch Biochem Biophys. 2011;526:159–66. doi:10.1016/j.abb.2012.03.021.
- Jiang XR, Song A, Bergelson S, Arroll T, Parekh B, May K, Chung S, Strouse R, Mire-Sluis A, Schenerman M. Advances in the assessment and control of the effector functions of therapeutic antibodies. Nat Rev Drug Discov. 2011;10:101–11. doi:10.1038/nrd3365. PMID:21283105.
- Niwa R, Satoh M. The current status and prospects of antibody engineering for therapeutic use: focus on glycoengineering technology. J Pharm Sci. 2015;104:930–41. doi:10.1002/jps.24316. PMID:25583555.
- Reichert JM. Marketed therapeutic antibodies compendium. mAbs. 2012;4:413–5. doi:10.4161/mabs.19931. PMID:22531442.
- Reichert JM. Antibodies to watch in 2014. mAbs. 2014;6:5–14. doi:10.4161/mabs.27333. PMID:24284914.
- Reichert JM, Dhimolea E. The future of antibodies as cancer drugs. Drug Discov Today. 2012;17:954–63. doi:10.1016/j.drudis.2012.04.006. PMID:22561895.
- Scott AM, Allison JP, Wolchok JD. Monoclonal antibodies in cancer therapy. Cancer Immun. 2012;12:14. PMID:22896759.
- Kanda Y, Yamada T, Mori K, Okazaki A, Inoue M, Kitajima-Miyama K, Kuni-Kamochi R, Nakano R, Yano K, Kakita S, et al. Comparison of biological activity among nonfucosylated therapeutic IgG1 antibodies with three different N-linked Fc oligosaccharides: the high-mannose, hybrid, and complex types. Glycobiology. 2007;17:104–18. doi:10.1093/glycob/cwl057. PMID:17012310.
- Anthony RM, Nimmerjahn F. The role of differential IgG glycosylation in the interaction of antibodies with FcgammaRs in vivo. Curr Opin Organ Transplant. 2011;16:7–14. doi:10.1097/MOT.0b013e328342538f. PMID:21150612.
- Arnold JN, Wormald MR, Sim RB, Rudd PM, Dwek RA. The impact of glycosylation on the biological function and structure of human immunoglobulins. Annu Rev Immunol. 2007;25:21–50. doi:10.1146/annurev.immunol.25.022106.141702. PMID:17029568.
- Jefferis R. Glycosylation of recombinant antibody therapeutics. Biotechnol Prog. 2005;21:11–6. doi:10.1021/bp040016j. PMID:15903235.
- Raju TS. Terminal sugars of Fc glycans influence antibody effector functions of IgGs. Curr Opin Immunol. 2008;20:471–8. doi:10.1016/j.coi.2008.06.007. PMID:18606225.
- Ferrara C, Brunker P, Suter T, Moser S, Puntener U, Umana P. Modulation of therapeutic antibody effector functions by glycosylation engineering: influence of Golgi enzyme localization domain and co-expression of heterologous beta1, 4-N-acetylglucosaminyltransferase III and Golgi alpha-mannosidase II. Biotechnol Bioeng. 2009;93:851–61. doi:10.1002/bit.20777.
- Schuster M, Umana P, Ferrara C, Brunker P, Gerdes C, Waxenecker G, Wiederkum S, Schwager C, Loibner H, Himmler G, et al. Improved effector functions of a therapeutic monoclonal Lewis Y-specific antibody by glycoform engineering. Cancer Res. 2005;65:7934–41. doi:10.1158/0008-5472.CAN-04-4212. PMID:16140965.
- Yamane-Ohnuki N, Satoh M. Production of therapeutic antibodies with controlled fucosylation. mAbs. 2009;1:230–6. doi:10.4161/mabs.1.3.8328. PMID:20065644.
- Mori K, Iida S, Yamane-Ohnuki N, Kanda Y, Kuni-Kamochi R, Nakano R, Imai-Nishiya H, Okazaki A, Shinkawa T, Natsume A, et al. Non-fucosylated therapeutic antibodies: the next generation of therapeutic antibodies. Cytotechnology. 2007;55:109–14. doi:10.1007/s10616-007-9103-2. PMID:19003000.
- Shinkawa T, Nakamura K, Yamane N, Shoji-Hosaka E, Kanda Y, Sakurada M, Uchida K, Anazawa H, Satoh M, Yamasaki M, et al. The absence of fucose but not the presence of galactose or bisecting N-acetylglucosamine of human IgG1 complex-type oligosaccharides shows the critical role of enhancing antibody-dependent cellular cytotoxicity. J Biol Chem. 2003;278:3466–73. doi:10.1074/jbc.M210665200. PMID:12427744.
- Shields RL, Lai J, Keck R, O'Connell LY, Hong K, Meng YG, Weikert SH, Presta LG. Lack of fucose on human IgG1 N-linked oligosaccharide improves binding to human Fcgamma RIII and antibody-dependent cellular toxicity. J Biol Chem. 2002;277:26733–40. doi:10.1074/jbc.M202069200. PMID:11986321.
- Kanda Y, Yamane-Ohnuki N, Sakai N, Yamano K, Nakano R, Inoue M, Misaka H, Iida S, Wakitani M, Konno Y, et al. Comparison of cell lines for stable production of fucose-negative antibodies with enhanced ADCC. Biotechnol Bioeng. 2006;94:680–8. doi:10.1002/bit.20880. PMID:16609957.
- Uozumi N, Teshima T, Yamamoto T, Nishikawa A, Gao YE, Miyoshi E, Gao CX, Noda K, Islam KN, Ihara Y, et al. A fluorescent assay method for GDP-L-Fuc:N-acetyl-beta-D-glucosaminide alpha 1-6fucosyltransferase activity, involving high performance liquid chromatography. J Biochem. 1996;120:385–92. doi:10.1093/oxfordjournals.jbchem.a021424. PMID:8889825.
- Mori K, Kuni-Kamochi R, Yamane-Ohnuki N, Wakitani M, Yamano K, Imai H, Kanda Y, Niwa R, Iida S, Uchida K, et al. Engineering Chinese hamster ovary cells to maximize effector function of produced antibodies using FUT8 siRNA. Biotechnol Bioeng. 2004;88:901–8. doi:10.1002/bit.20326. PMID:15515168.
- Zhou Q, Shankara S, Roy A, Qiu H, Estes S, Vie-Wylie A, Culm-Merdek K, Park A, Pan C, Edmunds T. Development of a simple and rapid method for producing non-fucosylated oligomannose containing antibodies with increased effector function. Biotechnol Bioeng. 2008;99:652–65. doi:10.1002/bit.21598. PMID:17680659.
- Yamane-Ohnuki N, Kinoshita S, Inoue-Urakubo M, Kusunoki M, Iida S, Nakano R, Wakitani M, Niwa R, Sakurada M, Uchida K, et al. Establishment of FUT8 knockout Chinese hamster ovary cells: an ideal host cell line for producing completely defucosylated antibodies with enhanced antibody-dependent cellular cytotoxicity. Biotechnol Bioeng. 2004;87:614–22. doi:10.1002/bit.20151. PMID:15352059.
- Umana P, Jean-Mairet J, Moudry R, Amstutz H, Bailey JE. Engineered glycoforms of an antineuroblastoma IgG1 with optimized antibody-dependent cellular cytotoxic activity. Nat Biotechnol. 1999;17:176–80. doi:10.1038/6179. PMID:10052355.
- Schachter H. Biosynthetic controls that determine the branching and microheterogeneity of protein-bound oligosaccharides. Biochem Cell Biol. 1986;64:163–81. doi:10.1139/o86-026. PMID:3521675.
- Kim TK, Eberwine JH. Mammalian cell transfection: the present and the future. Anal Bioanal Chem. 2010;397:3173–8. doi:10.1007/s00216-010-3821-6. PMID:20549496.
- Hossler P, Khattak SF, Li ZJ. Optimal and consistent protein glycosylation in mammalian cell culture. Glycobiology. 2009;19:936–49. doi:10.1093/glycob/cwp079. PMID:19494347.
- Ahn WS, Jeon JJ, Jeong YR, Lee SJ, Yoon SK. Effect of culture temperature on erythropoietin production and glycosylation in a perfusion culture of recombinant CHO cells. Biotechnol Bioeng. 2008;101:1234–444. doi:10.1002/bit.22006. PMID:18980186.
- Borys MC, Linzer DI, Papoutsakis ET. Culture pH affects expression rates and glycosylation of recombinant mouse placental lactogen proteins by Chinese hamster ovary (CHO) cells. Biotechnology (N Y). 1993;11(6):720–4. PMID:7763675.
- Ryll T. Mammalian cell culture process for producing glycoproteins. 2003; US 6528286 B1. [release date March 4, 2003].
- Gramer MJ, Eckblad JJ, Donahue R, Brown J, Shultz C, Vickerman K, Priem P, van den Bremer ET, Gerritsen J, van Berkel PH. Modulation of antibody galactosylation through feeding of uridine, manganese chloride, and galactose. Biotechnol Bioeng. 2011;108:1591–602. doi:10.1002/bit.23075. PMID:21328321.
- Campbell C, Stanley P. The Chinese hamster ovary glycosylation mutants LEC11 and LEC12 express two novel GDP-fucose:N-acetylglucosaminide 3-alpha-L-fucosyltransferase enzymes. J Biol Chem. 1984;259:11208–14. PMID:6540777.
- Crowell CK, Grampp GE, Rogers GN, Miller J, Scheinman RI. Amino acid and manganese supplementation modulates the glycosylation state of erythropoietin in a CHO culture system. Biotechnol Bioeng. 2007;96:538–49. doi:10.1002/bit.21141. PMID:16937399.
- Gawlitzek M, Estacio M, Fürch T, Kiss R. Identification of cell culture conditions to control N-glycosylation site-occupancy of recombinant glycoproteins expressed in CHO cells. Biotechnol Bioeng. 2009;103:1164–75. doi:10.1002/bit.22348. PMID:19418565.
- Carlson K, Pomerantz SC, Vafa O, Naso M, Strohl W, Mains RE, Eipper BA. Optimizing production of Fc-amidated peptides by Chinese hamster ovary cells. BMC Biotechnol. 2015;15:95. doi:10.1186/s12896-015-0210-4. PMID:26475607.
- Reusch D, Haberger M, Kailich T, Heidenreich AK, Kampe M, Bulau P, Wuhrer M. High-throughput glycosylation analysis of therapeutic immunoglobulin G by capillary gel electrophoresis using a DNA analyzer. mAbs. 2014;6:185–96. doi:10.4161/mabs.26712. PMID:24135630.
- Anthony RM, Ravetch JV. A novel role for the IgG Fc glycan: the anti-inflammatory activity of sialylated IgG Fcs. J Clin Immunol. 2010;30 Suppl 1:S9–14. doi:10.1007/s10875-010-9405-6. PMID:20480216.
- Jefferis R. The glycosylation of antibody molecules: functional significance. Glycoconj J. 1993;10:358–61. PMID:8298303.
- Xue J, Zhu LP, Wei Q. IgG-Fc N-glycosylation at Asn297 and IgA O-glycosylation in the hinge region in health and disease. Glycoconj J. 2013;30:735–45. doi:10.1007/s10719-013-9481-y. PMID:23783413.
- Golay J, Da RF, Bologna L, Ferrara C, Leusen JH, Rambaldi A, Klein C, Introna M. Glycoengineered CD20 antibody obinutuzumab activates neutrophils and mediates phagocytosis through CD16B more efficiently than rituximab. Blood. 2013;122:3482–91. doi:10.1182/blood-2013-05-504043. PMID:24106207.
- Mossner E, Brunker P, Moser S, Puntener U, Schmidt C, Herter S, Grau R, Gerdes C, Nopora A, van Puijenbroek E, et al. Increasing the efficacy of CD20 antibody therapy through the engineering of a new type II anti-CD20 antibody with enhanced direct and immune effector cell-mediated B-cell cytotoxicity. Blood. 2010;115:4393–402. doi:10.1182/blood-2009-06-225979. PMID:20194898.
- Natsume A, Wakitani M, Yamane-Ohnuki N, Shoji-Hosaka E, Niwa R, Uchida K, Satoh M, Shitara K. Fucose removal from complex-type oligosaccharide enhances the antibody-dependent cellular cytotoxicity of single-gene-encoded antibody comprising a single-chain antibody linked the antibody constant region. J Immunol Methods. 2005;306:93–103. doi:10.1016/j.jim.2005.07.025. PMID:16236307.
- Wright A, Morrison SL. Effect of altered CH2-associated carbohydrate structure on the functional properties and in vivo fate of chimeric mouse-human immunoglobulin G1. J Exp Med. 1994;180:1087–96. doi:10.1084/jem.180.3.1087. PMID:8064227.
- Chusainow J, Yang YS, Yeo JH, Toh PC, Asvadi P, Wong NS, Yap MG. A study of monoclonal antibody-producing CHO cell lines: what makes a stable high producer? Biotechnol Bioeng. 2009;102:1182–96. doi:10.1002/bit.22158. PMID:18979540.
- Kim M, O'Callaghan PM, Droms KA, James DC. A mechanistic understanding of production instability in CHO cell lines expressing recombinant monoclonal antibodies. Biotechnol Bioeng. 2011;108:2434–46. doi:10.1002/bit.23189. PMID:21538334.
- Osterlehner A, Simmeth S, Göpfert U. Promoter methylation and transgene copy numbers predict unstable protein production in recombinant Chinese hamster ovary cell lines. Biotechnol Bioeng. 2011;108:2670–81. doi:10.1002/bit.23216. PMID:21618470.
- Veith N, Ziehr H, MacLeod RA, Reamon-Buettner SM. Mechanisms underlying epigenetic and transcriptional heterogeneity in Chinese hamster ovary (CHO) cell lines. BMC Biotechnol. 2016;16:6. doi:10.1186/s12896-016-0238-0. PMID:26800878.
- Yu M, Brown D, Reed C, Chung S, Lutman J, Stefanich E, Wong A, Stephan JP, Bayer R. Production, characterization, and pharmacokinetic properties of antibodies with N-linked mannose-5 glycans. MAbs. 2012;4:475–87. doi:10.4161/mabs.20737. PMID:22699308.
- Goetze AM, Liu YD, Zhang Z, Shah B, Lee E, Bondarenko PV, Flynn GC. High-mannose glycans on the Fc region of therapeutic IgG antibodies increase serum clearance in humans. Glycobiology. 2011;21:949–59. doi:10.1093/glycob/cwr027. PMID:21421994.
- Pacis E, Yu M, Autsen J, Bayer R, Li F. Effects of cell culture conditions on antibody N-linked glycosylation-what affects high mannose 5 glycoform. Biotechnol Bioeng. 2011;108(10):2348–58. doi:10.1002/bit.23200. PMID:21557201.
- Zeck A, Regula JT, Larraillet V, Mautz B, Popp O, Göpfert U, Wiegeshoff F, Vollertsen UE, Gorr IH, Koll H, et al. Low level sequence variant analysis of recombinant proteins: an optimized approach. PLoS One. 2012;7(7):e40328. doi:10.1371/journal.pone.0040328. PMID:22792284.
- Papac DI, Briggs JB, Chin ET, Jones AJ. Ahigh-throughput microscale method to release N-linked oligosaccharides from glycoproteins for matrix-assisted laser desorption/ionization time-of-flight mass spectrometric analysis. Glycobiology. 1998;8:445–54. doi:10.1093/glycob/8.5.445. PMID:9597542.
- Kabat EA, Wu TT, Foeller C, Perry HM, Gottesman KS. Sequences of proteins of immunological interest. 5th Ed. US Department of Health and Human Services, NIH publication n 91-3242, 1991; pp. 662,680,689.