ABSTRACT
The use of nanomaterials in food-contact applications has created enormous interest in recent years. The potential migration of engineered nano-objects (ENOs) from food-contact materials (FCMs) is one of the most important concerns regarding potential human exposure to ENOs and health risks. Current research focusing on FCMs has often reached inconsistency regarding migration of ENOs. The scope of this critical review is to give a concise overview of the most relevant aspects of the subject, and to identify and discuss the major open questions in relation to migration of ENOs from FCMs. This includes the very fundamental questions whether ENOs can migrate from FCMs at all and what the potential release mechanisms of ENOs could be. The inconsistency of findings from experimental studies is highlighted based on the example of silver nanoparticle migration from polymer-based FCMs. Challenges in the detection and characterisation of ENOs in migration studies and the suitability of the most frequently used analytical techniques are discussed. Further, this review questions the suitability of standard food simulants and migration test conditions for FCMs as well as of conventional mathematical migration models. Considerations regarding the risk for consumers associated with migrating ENOs from FCMs are discussed.
Graphical Abstract
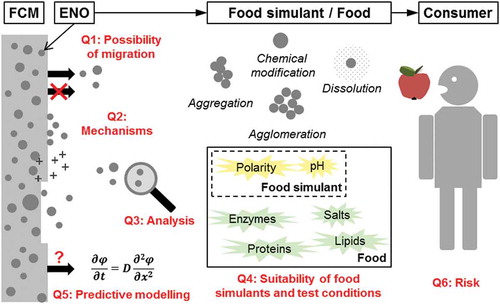
Introduction
The development of new materials based on nanotechnology has created great interest in the field of food-contact materials (FCMs). FCMs are materials and articles intended to come into contact with food during food production, processing, storage, preparation and serving (European Commission Citation2016). Introduction of nanomaterials into the food packaging industry has led to an improvement of flexibility, barrier properties, mechanical strength, thermal resistance and antimicrobial properties of packaging materials (Chaudhry et al. Citation2008; Bumbudsanpharoke & Ko Citation2015). Emerging food packaging materials, which could possess functional properties as an active food packaging, have the potential to increase the shelf life of food products, improve food safety, reduce food waste and subsequently alleviate global food supply issues (Hannon et al. Citation2015a). The global use of nanomaterials in the food packaging market was estimated to be US$6.5 billion in 2013 and was predicted to reach about US$15.0 billion in 2020 at a compound annual growth rate (CAGR) of 12.7% (Persistence Market Research Citation2014; Bumbudsanpharoke & Ko Citation2015). The European Institute for Health and Consumer Protection estimated the global nano-enabled food packaging market to be US$20 billion by 2020 (Belli Citation2012; Bumbudsanpharoke & Ko Citation2015). For comparison, the global food packaging market is projected to reach US$306 billion by 2019 (Research and Markets Citation2015).
One major application of nanotechnology for FCMs is the addition of engineered nano-objects (ENOs) to polymeric matrices. ENOs are discrete pieces of material with one, two or three external dimensions in the nanoscale and which are designed for specific purpose or function. The nanoscale has been defined to refer to the length interval of approximately 1–100 nm (ISO Citation2015). ENOs can be incorporated into polymers in embedded, fixed or bonded forms (Wyser et al. Citation2016). They can be nanoparticles, nanofibres or nanoplates, as well as sother structures. According to ISO (Citation2015), nanoparticles are nano-objects with all external dimensions in the nanoscale and where the lengths of the longest and shortest axes do not differ significantly. For nanofibres the third dimension is significantly larger (typically by more than three times) and not necessarily in the nanoscale, whereas for nanoplates two external dimensions are significantly larger. According to the European Commission’s recommendation on the definition of a nanomaterial from 2011, a nanomaterial is defined as ‘a natural, incidental or manufactured material containing particles, in an unbound state or as an aggregate or as an agglomerate and where, for 50% or more of the particles in the number size distribution, one or more external dimensions is in the size range 1–100 nm’. A particle is defined as ‘a minute piece of matter with defined physical boundaries’ (European Commission Citation2011a).
Examples of FCMs containing ENOs which are currently on the market (the actual elemental nanomaterial in parentheses) include refrigerators (Ag, Fe), cutting boards (Ag), frying pans (Ag), food storage containers (Ag), storage bags (Ag) and wok pans (Ti) (BUND – Friends of the Earth Germany Citation2016; Center for Food Safety (USA) Citation2016; DTU Environment and The Danish Ecological Council and Danish Consumer Council Citation2016).
Due to a potential human exposure, the importance of estimating migration of ENOs from FCMs to food has attracted the interest of scientific and legislative communities (Arvanitoyannis & Bosnea Citation2004). Migration is defined as the mass transfer of a substance or component from a FCM to foodstuff by sub-microscopic processes (Katan Citation1996). Convective or bulk processes (e.g., contamination by macroscopic fragments) are excluded, but otherwise there is little restriction on the scientific processes involved (Katan Citation1996). Migration is considered as a main critical factor in risk assessment of FCMs (Meulenaer Citation2009). The potential health risk of migrating ENOs is related to their small size and thereby potentially to exhibit different physico-chemical properties in comparison with the bulk form of the material as well as potentially higher bioavailability due to increased and faster passage thorough natural biological barriers (Noonan et al. Citation2014).
According to EFSA’s scientific opinion (EFSA Citation2009), the potential risk arising from nanoscience and nanotechnology in food and FCMs has to be clarified, and approval for the application of new FCMs depends on migration assessment. Migration studies including studies on migration mechanisms will lead to enhanced knowledge about the safety of FCMs (Huang et al. Citation2015). According to EU Regulation No. 10/2011 (European Commission Citation2011b), only nanoparticles of titanium nitride (TiN) (FCM 807) are authorised to be used in FCMs with the following restrictions: no migration of TiN occurs; only to be used in polyethylene terephthalate (PET) up to 20 mg kg–1; and the agglomerates in PET should have a diameter of 100–500 nm consisting of primary TiN nanoparticles with a diameter of approximately 20 nm. Two other substances, carbon black (FCM 411) and silicon dioxide (FCM 504), are authorised but not listed as nanoparticles in EU Regulation No. 10/2011. Carbon black should consist of aggregated particles of size 100–1200 nm originating from primary particles of 10–300 nm (which may form agglomerates within the size distribution of 300 nm to mm size). Similarly, silicon dioxide should consist of aggregates particles of 100–1000 nm originating from primary particles of 1–100 nm (which may form agglomerates within the size distribution of 300 nm to mm size) (European Commission Citation2011b).
A number of review papers on the potential migration of ENOs from FCMs already exist. However, they focus on specific aspects, like test methods (Calzolai et al. Citation2012; Noonan et al. Citation2014), safety assessment (Huang et al. Citation2015), market situation and safety regulations (Bumbudsanpharoke & Ko Citation2015; Hannon et al. Citation2015a), and legal framework (Wyser et al. Citation2016). The scope of this critical review is to give a concise overview of the most relevant aspects of the subject and to identify the major open questions in relation to migration of ENOs from FCMs. The inconsistency of findings from experimental studies is highlighted based on the example of silver (Ag) nanoparticle migration from FCMs. The review focuses on polymer-based FCMs as most applications and research has been concentrating on these types of materials. However, similar considerations will apply for migration of ENOs from non-polymeric FCMs, like paper and board, rubber, adhesives and printing inks.
Open question 1: Can ENOs migrate from FCMs at all?
The most important inconsistency in the literature within the area of FCMs is whether or not migration of ENOs from FCMs is possible. The most prominent example of this inconsistency is migration of Ag nanoparticles (AgNPs) from polymer-based FCMs, which has been studied extensively in recent years (). According to a predictive migration model presented by Simon et al. (Citation2008), migration of AgNPs from packaging into food is only possible in the case of low dynamic viscosity polymers as polyethylene (PE) and polypropylene (PP) and when the nanoparticle radius is in the range of 1 nm. However, a relatively large number of experimental studies showed migration of AgNPs either by electron microscopy techniques and/or inductively coupled plasma-mass spectrometry in single-particle mode (sp-ICP-MS) (e.g., Huang et al. Citation2011; Echegoyen & Nerín Citation2013; Artiaga et al. Citation2015; Hannon et al. Citation2015b). The migrated AgNPs or AgNP agglomerates were reported to be in the size range of 10–950 nm in seven studies (), which is significantly larger than the possible size migration limit of around 1 nm (radius) estimated by Simon et al. (Citation2008). The fraction of nanoparticulate Ag in relation to the total migrated Ag ranged from below 1% to 69% (Echegoyen & Nerín Citation2013; von Goetz et al. Citation2013; Ramos et al. Citation2016). Several studies on AgNP-containing polymers did not examine if the observed migration of Ag was due to migration of AgNPs (e.g., Busolo et al. Citation2010; Emamifar et al. Citation2010; Song et al. Citation2011; Bott et al. Citation2014b; Jokar & Abdul Rahman Citation2014). Only one experimental study that applied a method to detect ENOs (transmission electron microscopy – TEM) described the absence of AgNPs in migrates from AgNP-containing polymer (Ntim et al. Citation2015).
Table 1. Silver migration studies from silver-based food-contact materials.
Table 2. Comparison of the analytical techniques used for studying migration of ENOs from FCMs. The presented detection limits are indicative values, as they strongly depend on the chemical composition of the ENOs and the used instrumentation.
The experimental studies about migration of Ag from FCMs are summarised in . The FCMs were either commercially available products or produced in the laboratory. The initial Ag concentrations in FCMs were in the range of 1–3000 and 1–50,000 µg g–1 for commercial and laboratory-produced FCMs, respectively. The maximum Ag migration was in the range of 0.01–4.2 and 1–670 µg dm–2 for commercial and laboratory-produced FCMs, respectively. Most of the published migration studies focus on Ag, which can be, on the one hand, explained by the relatively frequent use of Ag (as antimicrobial agent) in FCMs and, on the other, with the fact that Ag can be relatively easily analysed by sp-ICP-MS and electron microscopy. However, the solubility of Ag (particular in an acidic medium) and its tendency to react with chlorine and sulphur to form Ag salts make it a difficult case for studying migration and answering the generic question whether or not ENOs can migrate from FCMs.
Nevertheless, evidence for the migration of ENOs significantly larger than a few nanometres was not limited to AgNPs but, for example, also observed for layered double hydroxide (LDH-C12) from polylactic acid (PLA) with platelet sizes of up to 50 nm in the migrates (Schmidt et al. Citation2011), titanium dioxide nanoparticles from PE with a size range of 50–100 nm (Mackevica et al. Citation2016), and nanoclay platelets from low-density polyethylene (LDPE) with a size range of 500 nm–1 µm (larger external dimensions of the platelet) (Echegoyen et al. Citation2016). In the same way, studies exist where no migration was observed for other ENOs than AgNPs, e.g., nanoclay platelets (Cloisite® 30B) from PLA (Schmidt et al. Citation2009) and TiN nanoparticles in LDPE (Bott et al. Citation2014a). The latter study comes to the general conclusion that ‘due to the usual size, shape and aggregation of nanoparticles’ in polymeric-FCMs, ENOs are immobilised and exposure of the consumer to ENOs via migration from FCMs cannot be expected (Bott et al. Citation2014a).
Open question 2: If migration of ENOs occurs, what is the migration mechanism?
The migration of low molecular weight compounds (< 1000 g mol–1) from polymeric FCMs to food is well understood and determined by diffusion and sorption processes (Miltz Citation1987). Three sub-processes can be distinguished: (1) diffusion of the molecule in the polymer in the direction of food because of a concentration gradient; (2) desorption of the molecule from the polymer and subsequent adsorption by the food at the food-packaging interface; and, finally, (3) diffusion of the molecule in the food due to a concentration gradient (Meulenaer Citation2009). Diffusion depends on physico-chemical properties of the migrant (such as size, polarity and molecular weight), properties of the polymer material (such as thickness, surface, density and polarity), contact media properties (viscosity, acidity and composition), and external conditions (temperature, time of contact and pressure) (Noonan et al. Citation2014). A more detailed description of the topic is given, for example, by Piringer (Citation2007, Citation2008) and Mercea (Citation2008).
The fundamental difference between the low molecular weight compounds and ENOs is their size. Nanoparticles with sizes in the range of 1–100 nm are significantly larger than low molecular weight compounds. To underline the difference, we will try to estimate the hydrodynamic diameter (a parameter used to describe the size of nanoparticles) of low molecular weight compounds and the ‘molecular weight’ (a parameter used to describe the size of molecules) of a nanoparticle.
The conventional chemical substances in polymer-based FCMs listed in Commission Regulation (EU) No. 10/2011 (European Commission Citation2011b), including monomers, starting substances, additives and polymer production aids, have molecular weights in the range of 40–1000 g mol–1. To allow an approximate comparison of molecular weight and hydrodynamic diameter, the example of bovine serum albumin (BSA) can be used. BSA has a molecular weight of 66,000 g mol–1, which results in a hydrodynamic diameter of approximately 7 nm as determined by asymmetrical flow field-flow fractionation (AF4) (Yohannes et al. Citation2010). That means that a 1000 g mol–1 molecule is around times = four times smaller, i.e., around 2 nm. The other way around, one can calculate the quasi-molecular weight of a nanoparticle, as Bott et al. did for the case of spherical carbon black nanoparticles based on the particle volume (diameter) and density (Bott et al. Citation2014a). Nanoparticle diameters of 1 and 10 nm corresponded to molecular weights of 314 and 314,000 g mol–1.
The diffusion coefficient exponentially decreases with size, which results in very small diffusions coefficients for nanoparticles with sizes larger than a few nanometres (Simon et al. Citation2008; Bott et al. Citation2014a). Consequently, diffusion may not be the major responsible mechanisms for ENO migration. Noonan et al. discussed, besides diffusion, three other possible routes for ENO migration, namely: (1) desorption of the ENO from the FCM surface, (2) dissolution of the ENO, and (3) degradation of the polymer matrix surrounding the ENO (Noonan et al. Citation2014).
Desorption (1) would be most likely for FCMs in which the ENOs are located near or at the FCM surface, i.e., in the interfacial region between FCM and food. ENO attachment is controlled by electrostatic interactions between the surface of ENO and FCM. The bonding at the interface between ENO surface and FCM is likely affected by liquid characteristics of the contact medium (pH, ionic strength) as well as temperature, vibration and physical abrasion (Noonan et al. Citation2014). Unlike in the case of diffusion, size and mobility of the migrant are not limiting factors for the release of ENOs from the FCM surface (Noonan et al. Citation2014). Surface detachment of AgNPs was proposed by Echegoyan and Nerín as an explanation of their observed AgNP migration from PE food containers (Echegoyen & Nerín Citation2013). The authors explained that the migration process was based on surface detachment of encapsulated AgNPs followed by oxidative dissolution of Ag from the remaining nanoparticles over time. Desorption could also be responsible for a release of ENOs from the cut edges of the samples during migration testing. The so-called ‘cutting edge effect’ should be considered as more severe testing, and was discussed to generate unrealistic contact conditions compared with conventional use (Bott et al. Citation2014b).
Dissolution (2) involves the transformation of the ENO into ionic form. The large body of literature evidenced dissolution of metal-containing nanoparticles, like Ag () and zinc oxide nanoparticles, into ions from FCMs into food/food simulants (Kumar et al. Citation2005; Song et al. Citation2011; Jokar & Abdul Rahman Citation2014; Ntim et al. Citation2015). Dissolution rate depends on redox potential and surface functionalisation of the ENO as well as ionic strength, temperature and dissolved oxygen level of the surrounding medium. So far, it is not known whether ENOs migrate from the surface of the FCM into the food/food simulant and then dissolve into ions, or whether the ions desorb from the surface of ENOs while the ENOs are still dispersed in the FCM (Noonan et al. Citation2014). Von Goetz et al. observed a significant decrease of Ag migration in repeated migrations tests already after the first use (von Goetz et al. Citation2013). This finding indicated that Ag release mainly occurred from the uppermost layer of the polymer where the food simulant could enter the porous FCM surface. The oxidative dissolution of migrated AgNPs to Ag ions increases in acidic pH (Elzey & Grassian Citation2010). In agreement with that, a lower AgNP fraction versus total Ag was detected in acidic food simulants (acetic acid) in comparison with alcoholic food simulants (Echegoyen & Nerín Citation2013). A secondary formation of ENOs after migration of ions, as in the case of AgNPs, by chemical reduction of migrating ions in the food simulant could not be excluded in the published studies (Mackevica et al. Citation2016), but was seen as less likely than the actual migration of nanoparticles (von Goetz et al. Citation2013).
Degradation of the polymeric matrix of the FCM (3) may take place by chemical or mechanical decomposition of the matrix and may lead to a release of the embedded ENOs. Degradation could be caused by external factors such as physical abrasion, heating and UV exposure, or by internal factors such as impurities in the polymer causing hydrolysis (Bhunia et al. Citation2013). Hydrolysis could change the gross properties of the polymer and subsequently increase the risk of potential release of ENOs (Noonan et al. Citation2014). Migration of LDH from PLA films was attributed to hydrolysis of PLA in the food simulant (95% ethanol, 40°C, 10 days) (Schmidt et al. Citation2011). The corresponding molecular weight reduction of the polymer was proven by gel permeation chromatography. A greater decrease in polymer molecular weight in PLA containing nano-dispersed LDH platelets in comparison with the pure PLA was attributed to a catalytic effect of the LDH platelets. Micro- and nano-sized polymer particles (PE and PP) of different shapes were detected by SEM in the migration solutions from both AgNP or nanoclay-containing food storage containers (Echegoyen & Nerín Citation2013). Further, AgNPs still embedded in or attached to the pieces of the polymer (PE) matrix were found in migration solutions by Mackevica et al. (Citation2016). The finding of polymeric particles in migration solutions could support the hypothesis that the decomposition of the polymer plays a role in ENO migration (Echegoyen & Nerín Citation2013; Echegoyen et al. Citation2016).
To the best of our knowledge, no studies on migration mechanisms of ENOs from FCMs exist. In published migration studies, discussions of underlying migration mechanism are most often hypotheses, but no proof is given. It is possible that migration of ENOs from FCMs can occur via a combination of the above-described mechanisms. The migration mechanisms will most likely depend on the properties of the ENO (e.g., size, shape, chemical composition), the properties of the given polymer (e.g., density, viscosity, solubility, (bio)degradability, swelling behaviour) and the distribution of the ENOs within the FCM (e.g., homogenous, surface-bound, only in the inner layer). Desorption, dissolution, and degradation processes and the influence of external factors such as UV radiation and abrasion are also considered in studies that focus on the environmental release of ENO, like titanium dioxide nanoparticles from painted surfaces or carbon nanotube from composites (Nowack et al. Citation2012; Froggett et al. Citation2014). More emphasis should be placed on the exchange of knowledge within food and environmental research on the potential mechanism and factors affecting the release of ENO from polymers.
Open question 3: What are suitable analytical techniques for studying the migration of ENOs from FCMs?
In conventional (specific) migration testing of molecular substances, analytical methods normally need to answer two questions: (1) whether a certain substance is in the migrate (identity), and (2) how much of the substance is migrating (mass concentration). A molecule’s identity can be defined by its chemical formula. For ENO, ‘identity’ is defined by several parameters, like size, shape, porosity, surface coating or chemical composition (Linsinger et al. Citation2013). To analyse and determine ENOs, the applied analytical techniques should be able to (1) detect ENOs with high selectivity and high sensitivity, (2) quantify the concentration of the ENOs in the food or food simulant accurately and precisely, and (3) characterise the properties like size, shape, agglomeration state and chemical composition of released ENOs. Currently, no single analytical technique can fulfil all these requirements.
The most cited techniques for general detection and characterisation of ENOs are scanning and transmission electron microscopy (SEM/TEM), laser light scattering, AF4, centrifugation techniques, and the emerging technique of sp-ICP-MS. A more detailed description of the methods including their advantages and disadvantages can be found, for example, in Hassellöv et al. (Citation2008), Tiede et al. (Citation2008) and Calzolai et al. (Citation2012). The major analytical challenges in the case of migrations studies of FCMs are the typically low concentrations of migrating ENOs and the fact that mainly very small ENOs are expected to migrate. Similar challenges are reported for the detection and characterisation of ENOs in the environment (Nowack et al. Citation2012; Froggett et al. Citation2014). An overview of the techniques used for studying the migration of ENOs from FCMs, their corresponding LODs, and the information that can be obtained is given in .
Most of the migration studies of AgNPs () rely on ICP-MS because of its superior sensitivity (). The main limitation of ICP-MS is that it can only determine the total elemental concentration in the migration solution. No information about the form of the migrated element – ion, ENO or polymeric particle containing ENOs – can be given. The sp-ICP-MS technique can address these limitations to some extent as it can distinguish between ionic and nanoparticle forms of an element and provide (number-based) particle size distributions (Degueldre & Favarger Citation2003; Laborda et al. Citation2011; Mitrano et al. Citation2012). A big advantage of sp-ICP-MS is its high sensitivity. Typical particle concentrations for obtaining particle size distributions are in the range of 106–108 particles l–1 (Pace et al. Citation2012). This corresponds to particle mass concentrations in the pg l–1–ng l–1 range depending on the density and diameter of the nanoparticle. Currently, sp-ICP-MS is the only analytical technique that can directly quantify the ratio between ions and nanoform of a certain element in migration solutions. Sample preparation for sp-ICP-MS involves, in the case of migration solutions, direct analysis without the need for sample preparation (von Goetz et al. Citation2013; Mackevica et al. Citation2016), or simple dilution with ultrapure water (Ramos et al. Citation2016) or the food simulant itself (Linsinger et al. Citation2014). The possibility of direct analysis reduces the likelihood of sample preparation artefacts. Some authors chose to apply ultrasound, e.g., via a probe sonicator, to the migration solutions before analysis (Ramos et al. Citation2016). It needs to be critically evaluated whether such a high-intensity dispersion step is suitable or whether it could falsify the information about the size and agglomeration state of the migrating ENOs. No studies could be found that use sp-ICP-MS for studying the migration of ENOs from FCMs into real foods. Semi-solid or solid food matrices would require a suitable sample preparation method for converting the sample into an analysable suspension without altering the ENO. This can, for example, be achieved by degradation of the matrix with enzymes as used for the sp-ICP-MS analysis of AgNPs in chicken meat (Loeschner et al. Citation2013a; Peters et al. Citation2014). The main constraint of sp-ICP-MS is currently its size LOD which is around 10–20 nm in the case of gold and AgNPs but significantly higher for other elements that are contained in ENOs used in FCMs, like silicon and titanium (Laborda et al. Citation2014; Lee et al. Citation2014). Furthermore, sp-ICP-MS requires knowledge about the composition, density and shape of the ENO to be able to determine its size. Typically, a spherical nanoparticle is assumed, and potential agglomerates appear as larger nanoparticles. Several ongoing instrumental developments are addressing the current limitations of sp-ICP-MS with a focus on improving the size LOD and on allowing the detection of more than one element in one particle (Laborda et al. Citation2014).
Another often applied technique for detection and characterisation of ENOs in migration studies is electron microscopy. An overview of electron microscopic techniques that can be used for the analysis of ENOs in food is given in by Dudkiewicz et al. (Citation2011). Electron microscopy techniques not only allow visualisation of the ENOs, but also give information on their size and shape (Tiede et al. Citation2008). The high lateral resolution (< 0.1 nm for TEM and < 1 nm for SEM) allows the detection of much smaller particles than by sp-ICP-MS. The combination of SEM or TEM with energy-dispersive X-ray spectrometry (EDX) allows the determination of the elemental composition of the ENO. The required particle concentrations for detecting a sufficient particle number on the substrate (e.g., grids for TEM) are higher than in the case of sp-ICP-MS and typically in the µg l–1–mg l–1 range. Pre-concentration techniques like (ultra)centrifugation and evaporation can be used to overcome this problem. However, these sample preparations might lead to artefacts, like induced agglomeration and precipitation of ions. Another possibility for increasing the ENO concentration in the migration solution is the use of high-polymer/food-simulant ratios in the migration test (von Goetz et al. Citation2013). Classical electron microscopy requires dry samples, which means that migration solutions need to be dried on a suitable substrate before analysis by electron microscopy. This step can lead to artefacts, like induced agglomeration and precipitation of ions. Described sample preparation techniques of migration solutions for SEM include: pre-concentration by evaporation followed by air-drying on the substrate (Ramos et al. Citation2016), drop casting of migration solutions onto the substrate followed by drying in air (Echegoyen & Nerín Citation2013; Hannon et al. Citation2015b), and spin casting of droplets on the substrate (von Goetz et al. Citation2013). TEM sample preparation involved direct drop deposition on a TEM grid (Ntim et al. Citation2015), centrifugation on a TEM grid (von Goetz et al. Citation2013), and pre-concentration by centrifugation and evaporation for 2 days followed by drop deposition on TEM grid (Mackevica et al. Citation2016). Theoretically, electron microscopy methods would be suitable for detecting migrated ENOs in real food after appropriate sample preparation. However, the major challenge would be the analysis in the case of low ENO concentrations (µg l–1 range and lower) as discussed by Dudkiewicz et al. (Citation2011).
AF4 coupled to ICP-MS was used by Artiaga et al. (Citation2015) for studying AgNPs in migration solutions from commercial food containers. AgNP diameters from 40 to 60 nm were determined based on external size calibration with citrate-stabilised AgNPs, assuming the same particle–membrane interaction (e.g., extent of repulsion) between citrate-stabilised AgNPs and the migrating AgNPs. Before injection into the AF4 (injection volume 200 µl), the migration solution was dried by evaporation, resuspended in 0.5 ml of 0.01% sodium dodecyl sulphate at pH 8 (the carrier liquid used for AF4), sonicated in the ultrasonic bath or with a probe sonicator, and filtered through a 0.22-µm nylon filter. An overview on flow field-flow fractionation as analytical separation technique for studying ENO in food and environmental samples is given in von der Kammer et al. (Citation2011). Injected masses of particles are typically in the ng–µg range. For common injection volumes of 10–100 µl, the corresponding sample mass concentrations need to be in the range of µg l–1–mg l–1. Particles in the size range of approximately 1–1000 nm can be separated by AF4. In general, obtaining quantitative size information by AF4 is challenging and obtained size information should be confirmed with a second method (Loeschner et al. Citation2013b).
Note that ICP-MS, sp-ICP-MS and AF4-ICP-MS are only suitable for analysis of inorganic ENOs. Electron microscopy is also able to visualise organic and carbon-based ENOs, like organic pigments, carbon nanotubes and fullerenes, but the low density of these ENOs hampers detection. Staining with heavy metals may be used to create or increase contrast in the electron microscopic image (Dudkiewicz et al. Citation2011). No migration of carbon black from LDPE or PS could be detected by AF4 coupled to multi-angle light scattering (MALS) (Bott et al. Citation2014c). The LOD was estimated as 10 µg l–1 for an injection volume of 1 ml. To the best of our knowledge, no study exists that detected organic or carbon-based ENOs in migration solutions. It remains an open question whether no migration occurred in the reported studies or whether the applied analytical techniques were not suitable, e.g., in terms of LODs. In the cases of organic and carbon-based ENOs, where no elemental-specific detection like in the case of inorganic ENOs is possible, the analysis might also be challenged by particle contaminations from the used equipment and chemicals. Schmidt et al. detected structures with diameters from 100 to 1600 nm in blank food simulants by AF4 coupled to MALS (Schmidt et al. Citation2009), suggesting particle contamination of the migration cells and/or the used solvents.
A general challenge when analysing ENOs in migration studies is the lack of validated methods and of suitable reference materials to assure accuracy of the results with respect to determined sizes and concentrations.
Open question 4: Are standard food simulants and migration test conditions for plastic FCMs appropriate for studying the migration of ENOs?
According to the EU Regulation No. 10/2011 (European Commission Citation2011b), six food simulants can be used for migration testing of plastic FCMs: (1) ethanol 10% (v/v), (2) acetic acid 3% (v/v), (3) ethanol 20% (v/v), (4) ethanol 50% (v/v), (5) vegetable oil and (6) poly(2,6-diphenyl-ρ-phenylene oxide). These food simulants represent (1) foods with a hydrophilic character, (2) foods with a hydrophilic character and with pH lower than 4.5, (3) alcoholic foods with an alcohol content up to 20%, (4) alcoholic foods with an alcohol content of above 20% and foods with a lipophilic character/oil in water emulsions, (5) lipophilic foods with free fats at the surface, and (6) dry foods, respectively. Food simulants are used to simplify food matrices, and the test conditions should be equivalent to the worst foreseeable conditions of use. The question is whether or not standard migration test conditions and food simulants are suitable for studying migration of ENOs from FCMs.
In the majority of the migration studies with Ag (), the maximum migration of Ag (total Ag release) was observed for acetic acid, which is mimicking food with pH < 4.5. The dependence of migration rate on the food/food simulant is observed for molecular compounds as well. The migration behaviour at the FCM surface is typically described by the partition coefficient, which depends on the polarity of the molecule, the polymer and the food/food simulant (Franz & Störmer Citation2008). The ‘novel’ effect in the case of ENOs is that also the identity of the ENO, i.e., its size, shape or chemical composition, depends on the food/food simulant. It was, for example, shown by Mackevica et al. (Citation2016) for various commercially available food-storage containers that size distributions of migrated AgNPs were similar in deionised water and ethanol, but much larger particle diameters were observed in acetic acid. For one food storage container, dissolution of the AgNPs in acetic acid was observed. It was concluded that acetic acid could facilitate both dissolution and agglomeration/aggregation of AgNPs. In a recent study, the stability of AgNPs in three food simulants (water, ethanol 10% and acetic acid 3%) was investigated using AF4-ICP-MS and sp-ICP-MS (Ntim et al. Citation2016). The study showed that AgNPs were preserved in the presence of water and ethanol 10%, while acetic acid 3% caused significant dissolution. It remains open if the same behaviour can be expected for AgNPs in acidic food. There are a limited number of studies that investigate the stability of ENOs in real food. AgNPs were shown to be stable in apple juice for 4 and 21 days in orange juice (storage temperature of 2–8°C) based on sp-ICP-MS analysis (Witzler et al. Citation2016). For AgNPs spiked to chicken meat, a decrease of AgNP size already after 24 h of storage at 4°C indicated dissolution (Peters et al. Citation2014). A considerable reduction of the detected particle mass concentration after 48 h was explained by the formation and precipitation of insoluble Ag salts (Peters et al. Citation2014). To the best of our knowledge, no studies exist that detected and characterised ENOs after migration from a FCM into food.
The use of food simulants was established for molecular substances, where the chemical and physical structure of the migrant remains stable during migration testing and is not influenced by the food simulant itself. In contrast to molecules, ENOs can undergo transformation processes, like dissolution, chemical (surface) modification, agglomeration and aggregation which lead to changes of their chemical composition, shape and size. These processes are, besides the properties of the ENO, influenced by temperature, time and properties of the surrounding medium, like pH, ionic strength and chemical composition. Food simulants do not have the same chemical composition as the food they are mimicking. Consequently, agglomeration, aggregation and dissolution processes might occur in the food but not in the food simulant or the other way around. For example, can the formation of a ‘protein corona’ (adsorption of proteins onto nanoparticles) lead to steric stabilisation of ENOs and prevent agglomeration/aggregation in media with high electronic strength (Gebauer et al. Citation2012)? This phenomenon is so far mainly studied for ENOs interacting with biological fluids, but it was shown that ENOs interact with proteins and eventually other macromolecules in food in a similar way (Burcza et al. Citation2015). Proteins and other macromolecules are absent in food simulants. Another example is the chemical reaction of AgNPs with chlorine and sulphur which leads to the formation of insoluble Ag salts, like AgCl and Ag2S (Peters et al. Citation2014; Loeschner et al. Citation2015). Chlorine and sulphur as well as other sources of sulphur, like thiol groups of proteins (Liu et al. Citation2010) or hydrogen sulphide released by microorganisms (McMeekin et al. Citation1978), are absent in food simulants.
Besides the suitability of the standard food simulants, the suitability of the time and temperature of standard migration test conditions for FCM needs to be questioned. The test conditions are supposed to reflect worst-case conditions which lead to a maximum migration of the molecular compound. It is an open question if these are automatically worst-case conditions for the migration of ENOs, because ENOs are eventually released by different mechanisms (see ‘Open question 2’). To describe an example: higher temperature in migration testing of polymers typically increases the migration of molecules, as diffusion increases with temperature. In the case of soluble ENOs, increased temperature can result in enhanced dissolution, as solubility increases with temperature. As a consequence, the concentration of migrated ENOs will decrease. Data are necessary to understand how the properties of migrated ENOs change as function of time and temperature (Noonan et al. Citation2014).
The classical migration study where the FCM is immersed in the food simulant is based on the idea that migration mainly occurs via diffusion. If diffusion were negligible for ENOs in FCMs, as discussed above, the concept of migration testing by immersion of the FCM would not necessarily be obsolete. It could be equally used to study the release by desorption of the ENO, dissolution of the ENO and polymer degradation. However, additional factors, like mechanical forces (abrasion, vibration), microwave treatment, heating and UV exposure, will have to be considered as they can have a large influence on the release and occur in the ‘real-life’ use of FCMs. As described above, the ‘cutting-edge effect’ was discussed to generate unrealistic contact conditions (Bott et al. Citation2014b). This will be especially true if desorption of ENOs from the surface instead of diffusion were the dominating mechanism. The use of migration cells where the FCM is fixed in a frame or the ‘sealing’ of the cut edges would avoid or reduce this effect.
Finally, the potentially inhomogeneous distribution of the ENOs in the FCM needs to be considered for the design of the migration test. In a polymer film or sheet, ENOs could be located on one surface, on both surfaces, in the core of the material, or distributed with a concentration gradient across the thickness of the film/sheet. This distribution will impact the migration. For example, if ENOs only adsorb to the surface of the FCM, a higher migration of ENOs will be observed during the first uses but then decrease (significantly) during further uses of the FCM. A conclusion based on a single migration test would lead to an overestimation of the ENO release.
Open question 5: Can mathematical modelling be applied for predicting the migration of ENOs from FCMs?
Fundamentally, the best way to assess the exposure to a substance contained in an FCM is to conduct a migration test in which the concentration of the migrated substances in a food is measured under prescribed conditions. However, it is not always practical to perform migration experiments because they can be challenging, expensive, time consuming and difficult to generalise. Mathematical modelling can be used to predict the migration rate for different time/temperature conditions, different polymer properties and foods. Mathematical models to estimate migration rates are based on the physico-chemical properties of the host material (polymer) and basic diffusion physics. Classically, Fick's Laws of Diffusion is applied to predict the diffusion of small molecules through the polymer, which depends on the concentration gradient, solubility of the migrant in the polymer, and the contact liquid, temperature, shape and polarity of the migrant, as well as other additives that may be present in the polymer matrix (Pillai et al. Citation2014). Furthermore, a number of semi-empirical models have been developed to estimate migration from FCMs to food (Begley et al. Citation2005; Poças et al. Citation2008).
Predictive mathematical models were not developed for ENOs, so the feasibility of the conventional diffusion-based mathematical models to estimate the migration rate of ENOs needs to be evaluated. Simon et al. predicted the migration of 5-nm nanoparticles from FCMs to food for various polymers, temperatures and times based on Fick’s Second Law of Diffusion (Simon et al. Citation2008). The diffusion coefficient was calculated from the nanoparticle radius by the Stokes–Einstein relation. The calculations demonstrated that migration of ENOs (based on diffusion) could only occur in the case of small nanoparticles with a radius in the order of 1 nm from polymers with low dynamic viscosity, like polyolefins (LDPE, high-density polyethylene (HDPE), PP). It was predicted that migration will neither be detectable for larger ENOs which are bound to polymer matrix, nor for polymers with high dynamic viscosity such as PET and polystyrene (PS) (Simon et al. Citation2008). Bott et al. applied an existing migration model for conventional polymer additives (based on Fick’s Second Law of Diffusion) for nanoparticles (Bott et al. Citation2014a). Spherical nanoparticles consisting of carbon were assumed to be quasi-molecules for which quasi-molecular weights could be calculated based on the particle volume (diameter) and density. Carbon black was used as ‘worst-case nanoparticle’ as it has the lowest possible quasi-molecular weight for nanoparticles in general and consequently the highest possible diffusion coefficient. Diameters of 1 and 10 nm corresponded to molecular weights of 314 and 314,000 g mol–1. The diffusion coefficient decreased exponentially with increasing diameter and reached 1.1.E–35 cm2 s–1 for 10 nm particles. The model calculations showed that the migration of carbon black with a diameter of 1 and 10 nm from LDPE would be 770 and 3.5E–17 mg kg–1, respectively, after 10 days at 40°C for an initial concentration of carbon black in LDPE of 25,000 mg kg–1 (Bott et al. Citation2014b). In another study by the same authors, the migration of TiN nanoparticles from LDPE (initial concentration 1000 mg kg–1) was predicted to be 30.7–1.38 × 10–18 mg kg–1 after 10 days at 40°C for diameters of 1–10 nm, respectively. The authors concluded that only TiN nanoparticles up to 3.5 nm size would have the potential to migrate in detectable concentration (Bott et al. Citation2014a). For the calculations the diffusion coefficients calculated for carbon black were used and consequently diffusion and migration of TiN overestimated.
So far, all mathematical models applied for ENOs in FCMs assume diffusion as the main mechanism for migration, and they predict that migration of ENOs is negligible except for very small ENOs of a few nanometres in size. This is in contrast to the findings of much larger ENOs in experimental studies (), as described above, which supports the hypothesis that other physical mechanism than diffusion play a role in the migration of ENOs from FCMs. Consequently, other mathematical models are required that can account for dissolution of the ENO, desorption processes and degradation of the polymer. The classical diffusion-based models are mainly relevant for predicting the migration of very small ENOs (diameter less than 1 nm).
Open question 6: What are the risks of human exposure associated with migrating ENOs?
The last and probably most complex question is whether the eventually released ENOs pose a risk for human health. The toxicity of ENOs is known to depend on a variety of physico-chemical properties of the ENO. Three principles have been identified regarding nanoparticle toxicology that involve the unique characteristics of ENOs (Krug & Wick Citation2011). The ‘Transport principle’ explains that materials of a certain inherent toxicity may be particularly critical when they are in the nanoform. Uptake of ions and molecules in the body cells is usually very precisely regulated. However, if ENOs do not dissolve but remain stable for a long time or accumulate in cells, they may become ‘active’ in another way. The ‘Surface principle’ explains that the small size of ENOs may cause enhanced chemical reactivity by the large number of surface atoms and by surface effects, such as crystal lattice defects. The toxicity is, however, also strongly dependent on the nanomaterial itself including material properties, chemical composition, surface properties and potential impurities, the ‘Material principle’.
If ENOs migrate from FCMs and are persistent in food, the consumer will be exposed via the gastrointestinal (GI) tract. The mechanism of absorption of ENOs over the GI wall is complex, and little is known about the behaviour and fate of ENOs in the GI tract (EFSA Citation2009; Binderup et al. Citation2013). More detailed studies on the influence of physico-chemical characteristics of ENOs on GI absorption are needed. Data on rodents have shown that ENO can enter the body via intestinal absorption (Chen et al. Citation2006; SCENIHR Citation2009), but absorption was restricted to relatively small amounts of less than 1% of the dose expressed in mass units. GI tract absorption can be affected by different coatings of the ENOs (EFSA Citation2009; SCENIHR Citation2009). Proteins in food may lead to coating of the nanoparticle surface and this may significantly influence GI absorption and the potential to cross cellular barriers. To study the transformation of ENOs in the GI tract, testing of the ENO stability in GI fluids, e.g., by in vitro digestion testing, is recommended (EFSA Citation2011). To what extent different in vitro digestion models can lead to deviating conclusions regarding dissolution and degradation of nanomaterials has not yet been studied. Recently it was shown that in vitro digestion protocols for nanoparticles without food components may lead to misleading and inconclusive results of ENO uptake (Lichtenstein et al. Citation2015).
If ENOs are absorbed in the GI tract they can enter the blood stream and further into organs (SCENIHR Citation2009; Wyser et al. Citation2016). The liver and spleen seem in many cases to be the major target organs for accumulation of ENOs (De Jong et al. Citation2008; SCENIHR Citation2009). Distribution of gold nanoparticles in rats was found to be size dependent. The smallest particles showed the most widespread distribution in different organs, including blood, lung, liver, spleen, kidney, thymus, brain and testis (De Jong et al. Citation2008). Larger nanoparticles were mainly found in the liver and spleen. Nanoparticle–protein interactions in the body may change over time and enhance the membrane-crossing and cellular-penetration properties of the nanoparticles (Panté & Kann Citation2002; John et al. Citation2003; Dutta et al. Citation2007) and thereby affect their biological effect.
The current risk assessment paradigm for non-nanomaterials is considered applicable also for ENOs. However, it should include considerations regarding the specific properties of nanomaterials such as their chemical composition, physico-chemical properties and interaction with tissues (EFSA Citation2009, Citation2011). One of the challenges in evaluating the toxicity of ENOs is that their physico-chemical properties can change in different environments. Adequate characterisation of ENOs is essential to identify their physico-chemical form in a given environment (e.g., in food and under the given test conditions) and to identify if the ENO properties are affected by the different environments (EFSA Citation2011). In support of assessing the potential risk of ENOs in FCMs, EFSA has developed a guidance document (EFSA Citation2011) on the risk assessment of the application of nanoscience and nanotechnologies in the food and feed chain, which is aimed for use by applicants and risk assessors. As part of this document, a toxicity-testing strategy of engineered nanomaterials is outlined for six different cases, which are dependent on the persistence/degradation of ENOs (cases 1–4) and the availability of toxicity data for the non-nanoform (cases 5–6). The six cases are: (1) persistence of ENOs in the FCM, (2) migration of ENOs from the FCM, (3) transformation of ENOs into the non-nanoform before ingestion, (4) degradation of ENOs during digestion, and (5) availability of hazard information for the non-nanoform or (6) no hazard information available for the non-nanoform. illustrates how the risk of human exposure to ENOs increases for the different scenarios of cases 1–4. If ENOs migrate into food and persist in food and in GI fluids, the toxicity testing performed for hazard identification and characterisation should include specific nano-properties including comparison with data on the non-nanoform (if these data are available) as given by EFSA guidance (EFSA Citation2011).
The current EFSA guidance for FCMs in a non-nanoform (EFSA 2008) and a recent new opinion from EFSA (Citation2016) requires a different toxicological dataset to be provided by applicants depending on the amount of migration/the expected human exposure level for of a given substance. However, due to the limited knowledge of ENOs’ toxicity, such a paradigm is not considered appropriate by EFSA for FCM risk assessment at the moment. ENOs must be considered case by case (EFSA Citation2011, Citation2016). Whenever migration may occur, toxicological testing of the ENOs should be done in accordance with EFSA guidance, starting with the assessment of genotoxic potential (EFSA Citation2011, Citation2016).
A main limitation in the risk assessment of nanomaterials is the general lack of (high-quality) exposure data due to the difficulties in detection and characterisation of ENOs. As discussed for ‘ Open question 3’, appropriate analytical methods able to detect ENOs at a low level and within the full size range of 1–100 nm are essential to provide evidence for migration of ENOs. As dissolution, dissolution rate and physico-chemical properties of ENOs vary in different matrices, standard test methods to measure these parameters in the current environment are crucial as part of the risk assessment of nanomaterials (Howlett Citation2012). Most available data come from airborne measurements and uptake of ENOs by inhalation, whereas exposure estimates from food and consumer products are scarce (SCENIHR Citation2009; EFSA Citation2011; Binderup et al. Citation2013). Moreover, there is an urgent need for long-term exposure studies with ENOs as potential health effects are most likely to occur after long time exposure (SCENIHR Citation2009). A further issue, which should be considered if migration of ENOs into food occurs, is possible changes of the food matrix itself by interaction with the migrated ENOs. ENOs have the potential to interact with functional groups of organic molecules, such as carboxyl, hydroxyl, amino or carbonyl groups, which may lead to changes of proteins, lipids and polysaccharides in food (Kwak Citation2014).
Summary and conclusions
An evaluation of the current literature on the migration of ENOs from polymer-based FCMs showed that despite the increasing number of experimental studies, still many open questions remain. Six main open questions were identified (). They include the very fundamental question whether ENOs can migrate from FCMs at all (‘Open question 1’). Experimental studies are not giving a conclusive answer, as some observe migration of ENOs from FCMs and others do not. This can be partially attributed to lack of suitable analytical methods for the detection of low ENO quantities and small ENO sizes. We strongly suggest that studies that conclude that no migration occurred should add information about the LOD of the applied method not only for particle mass or number concentration but also for particle size. Predictive models only consider migration based on diffusion and therefore conclude that migration of ENOs (larger than a few nm) is not possible. Migration can, however, be caused by a number of other chemical and physical processes (Katan Citation1996). A clear legal definition of the term ‘migration’ would help to avoid these misunderstandings.
Potential release mechanisms of ENOs (‘Open question 2’) were discussed and a clear lack of data on the issue identified. The use of fluorescently (Meder et al. Citation2016) or isotopically labelled ENOs (Merrifield & Lead Citation2016), as in the fields of bionanotechnology and nanotoxicology, could help to study and identify dissolution and agglomeration processes. The behaviour of the relevant ions (e.g., Ag, Zn or Cu) in food and food simulants needs to be studied to evaluate whether or not secondary formation of ENOs after migration of ions is possible. After contact of the FCM with food/food simulants, the surface morphology of the materials (e.g., by scanning or atomic force microscopy) and the polymer structure (e.g., by infrared spectroscopy) should be studied to identify potential degradation of the polymer matrix. Characterisation of the ENOs within the FCM (before and after exposure to food/food simulant) in terms of size, shape, composition and localisation would significantly contribute to a better understanding of the results of migration studies and the potential release mechanisms.
The challenges in detection and characterising ENOs in migration studies and the suitability of the most frequently used analytical techniques were discussed (‘Open question 3’). The importance of suitable sample preparation was highlighted and the risk of sample preparation artefacts described. Due to different limitations (e.g., regarding size and concentration range) in every single applied technique, a combination of analytical techniques should preferable be used to improve the detection of ENOs. We suggest a combination of sp-ICP-MS and TEM-EDX for studying the migration of inorganic ENOs. The high sensitivity of sp-ICP-MS allows the detection of very low particle concentrations in food simulants without additional sample preparation. TEM-EDX gives information on particle shape and elemental composition, and can detect smaller ENOs than sp-ICP-MS. Lower size LODs of sp-ICP-MS would be highly beneficial for the research area. More studies should focus on studying the migration of organic or carbon-based ENOs, which is requiring the development of suitable methods for detection and characterisation of these types of ENOs. Most likely, labelling of the ENOs will be required to allow detection. Moreover, information about the surface properties of migrating ENOs should be part of the characterisation. For this, analytical methods need to be developed that can determine surface potential and surface chemistry of ENOs at relatively low particle concentrations.
Further, this review questions the suitability of standard food simulants and migration test conditions for ENOs in FCMs (‘Open question 4’). As food simulants do not have the same chemical composition as a real food, agglomeration, aggregation, and dissolution of ENOs, might not occur in the food simulant but in the food (or the other way around). Consequently, studies are required that compare the migration of ENOs into food simulants and into real food. These studies should not only determine the ENO concentrations but also the ENO properties (size, shape, agglomeration state, and chemical composition) in food simulants and food. The review suggests that additional other factors, like mechanical forces (abrasion, vibration), microwave treatment, heating and UV exposure, are considered in migration studies, as they potentially can have a large influence on the release of ENOs. The selected test conditions should reflect the potential use of the FCM.
Mathematical models (‘Open question 5’) that are able to account for other release mechanisms than diffusions are required. Inspiration can be gained from other research areas, and only a few examples will be given here. Models for mechanical degradation (abrasive wear) of polymers are developed in the field of mechanical engineering and material sciences, e.g., (Sinha et al. Citation2007; Abdelbary Citation2014). Models for decomposition of biodegradable polymers are studied in the context of medical applications, like drug delivery or implantable devices, e.g., (Vieira et al. Citation2014). Mathematical models for prediction of drug release from biodegradable polymers are able to model drug release from surface-eroding systems where the drug is released concurrently with the layer-by-layer erosion from the outermost surface of the matrix (Lao et al. Citation2008). Similar mechanisms are possible in the case of ENOs in FCMs based on biodegradable polymers.
At last, considerations regarding the risk for the consumer associated with migrating ENOs from FCM were discussed (‘Open question 6’). This question is probably the most complex question of all. Data are lacking in relation to all aspect of risk assessment including the fate of migrated ENOs in food and GI tract exposure to ENOs. Interactions of ENOs with food should be further studied, and ENOs characterised in different food matrices. Possible changes of the food matrix by interaction with (migrated) ENOs should be considered. More detailed studies on the influence of physico-chemical characteristics of ENOs on GI absorption are needed. The use of in vitro digestion models for predicting the fate of ENOs in the GI tract is recommended. However, a better understanding of the suitability of these models is required.
We suggest that further research should focus on answering these six questions to ensure safety of FCMs and to support the development of innovative and safe FCMs in future. A stronger collaboration with the research area that focuses on the environmental release of ENOs from solid materials is encouraged as it encounters similar challenges. This review focuses on polymer-based FCMs as most applications and research has been focusing on these types of materials. However, similar considerations will apply migration of ENOs from non-polymeric FCMs, like paper and board, rubber, adhesives and printing inks.
Disclosure statement
No potential conflict of interest was reported by the authors.
Additional information
Funding
References
- Abdelbary A. 2014. 8 – Prediction of wear in polymers and their composites. In: Wear of polymers and composites. Oxford: Woodhead; p. 185–217.
- Artiaga G, Ramos K, Ramos L, Cámara C, Gómez-Gómez M. 2015. Migration and characterisation of nanosilver from food containers by AF4-ICP-MS. Food Chem. 166:76–85.
- Arvanitoyannis IS, Bosnea L. 2004. Migration of substances from food packaging materials to foods. Crit Rev Food Sci Nutr. 44:63–76.
- Begley T, Castle L, Feigenbaum A, Franz R, Hinrichs K, Lickly T, Mercea P, Milana M, O’Brien A, Rebre S, et al. 2005. Evaluation of migration models that might be used in support of regulations for food-contact plastics. Food Addit Contam. 22:73–90.
- Belli B. 2012. Eating nano: processed foods and food packaging already contain nanoparticles—some of which could be harmful to our health. E – Environ Mag. [Internet]. [ cited 2016 May 26]. Available from: http://www.emagazine.com/magazine/eating-nano
- Bhunia K, Sablani SS, Tang J, Rasco B. 2013. Migration of chemical compounds from packaging polymers during microwave, conventional heat treatment, and storage. Compr Rev Food Sci Food Saf. 12:523–545.
- Binderup M-L, Bredsdorff L, Beltoft VM, Mortensen A, Loeschner K, Larsen EH, Eriksen FD. 2013. Systemic absorption of nanomaterials by oral exposure (Report to the Danish Environmental Protection Agency, Environmental Project No. 1505). Copenhagen: The Danish Environmental Protection Agency.
- Bott J, Störmer A, Franz R. 2014a. A model study into the migration potential of nanoparticles from plastics nanocomposites for food contact. Food Packag Shelf Life. 2:73–80.
- Bott J, Störmer A, Franz R. 2014b. A comprehensive study into the migration potential of nano silver particles from food contact polyolefins. In: Benvenuto M, editor. Chemistry of food, food supplements, and food contact materials: from production to plate. Vol. 1159. Washington, DC: American Chemical Society; p. 5–51.
- Bott J, Störmer A, Franz R. 2014c. Migration of nanoparticles from plastic packaging materials containing carbon black into foodstuffs. Food Addit Contam Part A. 31:1769–1782.
- Bumbudsanpharoke N, Ko S. 2015. Nano-food packaging: an overview of market, migration research, and safety regulations. J Food Sci. 80:R910–R923.
- BUND – Friends of the Earth Germany. 2016. Nanoproduktdatenbank (in German). [Internet]. [ cited 2016 Jun 3]. Available from: http://www.bund.net/nanodatenbank/
- Burcza A, Gräf V, Walz E, Greiner R. 2015. Impact of surface coating and food-mimicking media on nanosilver-protein interaction. J Nanoparticle Res. 17:1–15.
- Busolo MA, Fernandez P, Ocio MJ, Lagaron JM. 2010. Novel silver-based nanoclay as an antimicrobial in polylactic acid food packaging coatings. Food Addit Contam Part A. 27:1617–1626.
- Calzolai L, Gilliland D, Rossi F. 2012. Measuring nanoparticles size distribution in food and consumer products: a review. Food Addit Contam Part A Chem Anal Control Expo Risk Assess. 29:1183–1193.
- Center for Food Safety (USA). 2016. Nanotechnology in our food – an interactive database of consumer food products containing nanomaterials. [Internet]. [ cited 2016 Jun 3]. Available from: http://salsa3.salsalabs.com/o/1881/p/salsa/web/common/public/content?content_item_KEY=14112
- Chaudhry Q, Scotter M, Blackburn J, Ross B, Boxall A, Castle L, Aitken R, Watkins R. 2008. Applications and implications of nanotechnologies for the food sector. Food Addit Contam Part A. 25:241–258.
- Chen Z, Meng H, Xing G, Chen C, Zhao Y, Jia G, Wang T, Yuan H, Ye C, Zhao F, et al. 2006. Acute toxicological effects of copper nanoparticles in vivo. Toxicol Lett. 163:109–120.
- Cushen M, Kerry J, Morris M, Cruz-Romero M, Cummins E. 2013. Migration and exposure assessment of silver from a PVC nanocomposite. Food Chem. 139:389–397.
- Cushen M, Kerry J, Morris M, Cruz-Romero M, Cummins E. 2014. Evaluation and simulation of silver and copper nanoparticle migration from polyethylene nanocomposites to food and an associated exposure assessment. J Agric Food Chem. 62:1403–1411.
- De Jong WH, Hagens WI, Krystek P, Burger MC, Sips AJAM, Geertsma RE. 2008. Particle size-dependent organ distribution of gold nanoparticles after intravenous administration. Biomaterials. 29:1912–1919.
- Degueldre C, Favarger P-Y. 2003. Colloid analysis by single particle inductively coupled plasma-mass spectroscopy: a feasibility study. Colloids Surfaces A Physicochem Eng Asp. 217:137–142.
- DTU Environment and the Danish Ecological Council and Danish Consumer Council. 2016. The nanodatabase. [Internet]. [ cited 2016 Jun 3]. Available from: http://nanodb.dk/en/
- Dudkiewicz A, Tiede K, Loeschner K, Jensen LHS, Jensen E, Wierzbicki R, Boxall AB, Molhave K. 2011. Characterization of nanomaterials in food by electron microscopy. Trac Trends Anal Chem. 30:28–43.
- Dutta D, Sundaram SK, Teeguarden JG, Riley BJ, Fifield LS, Jacobs JM, Addleman SR, Kaysen GA, Moudgil BM, Weber TJ. 2007. Adsorbed proteins influence the biological activity and molecular targeting of nanomaterials. Toxicol Sci. 100:303–315.
- Echegoyen Y, Nerín C. 2013. Nanoparticle release from nano-silver antimicrobial food containers. Food Chem Toxicol. 62:16–22.
- Echegoyen Y, Rodríguez S, Nerín C. 2016. Nanoclay migration from food packaging materials. Food Addit Contam Part A. 33:530–539.
- EFSA. 2009. Scientific opinion of the scientific committee on a request from the European commission on the potential risks arising from nanoscience and nanotechnologies on food and feed safety. EFSA J. 958:1–39.
- EFSA. 2011. Scientific opinion on guidance on the risk assessment of the application of nanoscience and nanotechnologies in the food and feed chain (EFSA scientific committee). EFSA J. 9:36.
- EFSA. 2016. Scientific opinion on recent developments in the risk assessment of chemicals in food and their potential impact on the safety assessment of substances used in food contact materials. EFSA J. 14:1–28.
- Elzey S, Grassian VH. 2010. Agglomeration, isolation and dissolution of commercially manufactured silver nanoparticles in aqueous environments. J Nanoparticle Res. 12:1945–1958.
- Emamifar A, Kadivar M, Shahedi M, Soleimanian-Zad S. 2010. Evaluation of nanocomposite packaging containing Ag and ZnO on shelf life of fresh orange juice. Innov Food Sci Emerg Technol. 11:742–748.
- European Commission. 2011a. Commission recommendation of 18 October 2011 on the definition of nanomaterial (2011/696/EU). Off J Eur Union. L275:38–40.
- European Commission. 2011b. Commission Regulation (EU) No. 10/2011 of 14 January 2011 on plastic materials and articles intended to come into contact with food. Off J Eur Union. 15:12–88.
- European Commission. 2016. Food contact materials. [Internet]. [ cited 2016 Jun 3]. Available from: http://ec.europa.eu/food/safety/chemical_safety/food_contact_materials/index_en.htm
- Franz R, Störmer A. 2008. Migration of plastic constituents. In: Piringer OG, Baner AL, editors. Plastic packaging. Weinheim: Wiley-VCH Verlag GmbH & Co. KGaA; p. 349–415.
- Froggett SJ, Clancy SF, Boverhof DR, Canady RA. 2014. A review and perspective of existing research on the release of nanomaterials from solid nanocomposites. Part Fibre Toxicol. 11:17.
- Gallocchio F, Cibin V, Biancotto G, Roccato A, Muzzolon O, Carmen L, Simone B, Manodori L, Fabrizi A, Patuzzi I, et al. 2016. Testing nano-silver food packaging to evaluate silver migration and food spoilage bacteria on chicken meat. Food Addit Contam Part A. 33:1063–1071.
- Gebauer JS, Malissek M, Simon S, Knauer SK, Maskos M, Stauber RH, Peukert W, Treuel L. 2012. Impact of the nanoparticle – protein corona on colloidal stability and protein structure. Langmuir. 28:9673–9679.
- Hannon JC, Cummins E, Kerry J, Cruz-Romero M, Morris M. 2015a. Advances and challenges for the use of engineered nanoparticles in food contact materials. Trends Food Sci Technol. 43:43–62.
- Hannon JC, Kerry JP, Cruz-Romero M, Azlin-Hasim S, Morris M, Cummins E. 2015b. Assessment of the migration potential of nanosilver from nanoparticle coated low density polyethylene food packaging into food simulants. Food Addit Contam Part A. 33:167–178.
- Hassellöv M, Readman JW, Ranville JF, Tiede K. 2008. Nanoparticle analysis and characterization methodologies in environmental risk assessment of engineered nanoparticles. Ecotoxicology. 17:344–361.
- Howlett J. 2012. Practical guidance for the safety assessment of nanomaterials in food – summary report of a workshop held in April 2011 in Cascais, Portugal. Brussels: ILSI Europe; p. 1–15.
- Huang J-Y-Y, Li X, Zhou W. 2015. Safety assessment of nanocomposite for food packaging application. Trends Food Sci Technol. 45:187–199.
- Huang Y, Chen S, Bing X, Gao C, Wang T, Yuan B. 2011. Nanosilver migrated into food-simulating solutions from commercially available food fresh containers. Packag Technol Sci. 24:291–297.
- International Organization for Standardization (ISO). 2015. Nanotechnologies – vocabulary – part 2: nano-objects. (ISO/TS 80004-2:2015). Geneva: International Organization for Standardization (ISO).
- John TA, Vogel SM, Tiruppathi C, Malik AB, Minshall RD. 2003. Quantitative analysis of albumin uptake and transport in the rat microvessel endothelial monolayer. Am J Physiol Lung Cell Mol Physiol. 284:L187–L196.
- Jokar M, Abdul Rahman R. 2014. Study of silver ion migration from melt-blended and layered-deposited silver polyethylene nanocomposite into food simulants and apple juice. Food Addit Contam Part A. 31:734–742.
- Katan LL. 1996. Migration from food contact materials. [place unknown]: Blackie Academic & Professional.
- Krug HF, Wick P. 2011. Nanotoxicology: an interdisciplinary challenge. Angew Chem Int Ed Engl. 50:1260–1278.
- Kumar R, Howdle S, Münstedt H. 2005. Polyamide/silver antimicrobials: effect of filler types on the silver ion release. J Biomed Mater Res Part B Appl Biomater. 75B:311–319.
- Kwak H-S. 2014. Nano- and microencapsulation for foodstle. [place unknown]: John Wiley & Sons.
- Laborda F, Bolea E, Jiménez-Lamana J. 2014. Single particle inductively coupled plasma mass spectrometry: a powerful tool for nanoanalysis. Anal Chem. 86:2270–2278.
- Laborda F, Jiménez-Lamana J, Bolea E, Castillo JR. 2011. Selective identification, characterization and determination of dissolved silver(i) and silver nanoparticles based on single particle detection by inductively coupled plasma mass spectrometry. J Anal At Spectrom. 26:1362.
- Lao LL, Venkatraman SS, Peppas NA. 2008. Modeling of drug release from biodegradable polymer blends. Eur J Pharm Biopharm. 70:796–803.
- Lee S, Bi X, Reed RB, Ranville JF, Herckes P, Westerhoff P. 2014. Nanoparticle size detection limits by single particle ICP-MS for 40 elements. Environ Sci Technol. 48:10291–11030.
- Lichtenstein D, Ebmeyer J, Knappe P, Juling S, Böhmert L, Selve S, Niemann B, Braeuning A, Thünemann AF, Lampen A. 2015. Impact of food components during in vitro digestion of silver nanoparticles on cellular uptake and cytotoxicity in intestinal cells. Biol Chem. 396:1255–1264.
- Linsinger TPJ, Chaudhry Q, Dehalu V, Delahaut P, Dudkiewicz A, Grombe R, von der Kammer F, Larsen EH, Legros S, Loeschner K, et al. 2013. Validation of methods for the detection and quantification of engineered nanoparticles in food. Food Chem. 138:1959–1966.
- Linsinger TPJ, Peters R, Weigel S. 2014. International interlaboratory study for sizing and quantification of Ag nanoparticles in food simulants by single-particle ICPMS. Anal Bioanal Chem. 406:3835–3843.
- Liu J, Sonshine DA, Shervani S, Hurt RH. 2010. Controlled release of biologically active silver from nanosilver surfaces. ACS Nano. 4:6903–6913.
- Loeschner K, Navratilova J, Grombe R, Linsinger TPJ, Købler C, Mølhave K, Larsen EH. 2015. In-house validation of a method for determination of silver nanoparticles in chicken meat based on asymmetric flow field-flow fractionation and inductively coupled plasma mass spectrometric detection. Food Chem. 181:78–84.
- Loeschner K, Navratilova J, Købler C, Mølhave K, Wagner S, von der Kammer F, Larsen EH. 2013a. Detection and characterization of silver nanoparticles in chicken meat by asymmetric flow field flow fractionation with detection by conventional or single particle ICP-MS. Anal Bioanal Chem. 405:8185–8195.
- Loeschner K, Navratilova J, Legros S, Wagner S, Grombe R, Snell J, von der Kammer F, Larsen EH. 2013b. Optimization and evaluation of asymmetric flow field-flow fractionation of silver nanoparticles. J Chromatogr A. 1272:116–125.
- Mackevica A, Olsson ME, Hansen SF. 2016. Silver nanoparticle release from commercially available plastic food containers into food simulants. J Nanoparticle Res. 18:1–11.
- McMeekin TA, Gibbs PA, Patterson JT. 1978. Detection of volatile sulfide-producing bacteria isolated from poultry-processing plants. Appl Envir Microbiol. 35:1216–1218.
- Meder F, Thomas SS, Fitzpatrick LW, Alahmari A, Wang S, Beirne JG, Vaz G, Redmond G, Dawson KA. 2016. Labeling the structural integrity of nanoparticles for advanced in situ tracking in bionanotechnology. ACS Nano. 10:4660–4671.
- Mercea P. 2008. Models for diffusion in polymers. In: Piringer OG, Baner AL, editors. Plastic packaging – interactions with food and pharmaceuticals. 2nd ed. Weinheim: Wiley-VCH Verlag GmbH & Co. KGaA; p. 123–162.
- Merrifield RC, Lead JR. 2016. Preparation and characterization of three-layer, isotopically labelled core-shell nanoparticles; a tool for understanding mechanisms of bioavailability. NanoImpact. 2:54–60.
- Meulenaer BD. 2009. Migration from packaging materials. In: Costa R, Kristbergsson K, editors. Predictive modeling and riskassessment. Vol. 4. Boston, MA: Springer US; p. 139–151.
- Miltz J. 1987. Migration of low molecular weight species from packaging materials: theoretical and practical considerations. In: Gray JI, Harte BR, Miltz J, editors. Food Prod Compat Proc. Lancaster (PA): Technomic Publishing Company, Inc.; p. 30–37.
- Mitrano DM, Lesher EK, Bednar A, Monserud J, Higgins CP, Ranville JF. 2012. Detecting nanoparticulate silver using single-particle inductively coupled plasma-mass spectrometry. Environ Toxicol Chem. 31:115–121.
- Noonan GO, Whelton AJ, Carlander D, Duncan TV. 2014. Measurement methods to evaluate engineered nanomaterial release from food contact materials. Compr Rev Food Sci Food Saf. 13:679–692.
- Nowack B, Ranville JF, Diamond S, Gallego-Urrea JA, Metcalfe C, Rose J, Horne N, Koelmans AA, Klaine SJ. 2012. Potential scenarios for nanomaterial release and subsequent alteration in the environment. Environ Toxicol Chem. 31:50–59.
- Ntim SA, Thomas TA, Begley TH, Noonan GO. 2015. Characterisation and potential migration of silver nanoparticles from commercially available polymeric food contact materials. Food Addit Contam Part A. 32:1003–1011.
- Ntim SA, Thomas TA, Noonan GO. 2016. Influence of aqueous food simulants on potential nanoparticle detection in migration studies involving nanoenabled food-contact substances. Food Addit Contam Part A. 33:905–912.
- Pace HE, Rogers NJ, Jarolimek C, Coleman VA, Gray EP, Higgins CP, Ranville JF. 2012. Single particle inductively coupled plasma-mass spectrometry: a performance evaluation and method comparison in the determination of nanoparticle size. Environ Sci Technol. 46:12272–12280.
- Panté N, Kann M. 2002. Nuclear Pore Complex Is Able to Transport Macromolecules with Diameters of ~39 nm. Mol Biol Cell. 13:425–434.
- Persistence Market Research. 2014. Global study on nano-enabled packaging for food and beverages: intelligent packaging to witness highest growth by 2020. [Internet]. [ cited 2016 Sep 12]. Available from: http://www.persistencemarketresearch.com/market-research/nano-enabled-packaging-market.asp
- Peters RJB, Rivera ZH, Van Bemmel G, Marvin HJP, Weigel S, Bouwmeester H. 2014. Development and validation of single particle ICP-MS for sizing and quantitative determination of nano-silver in chicken meat characterisation of nanomaterials in biological samples. Anal Bioanal Chem. 406:3875–3885.
- Pillai KV, Hunt PR, Duncan TV. 2014. Nanoparticles in polymer nanocomposite food contact materials: uses, potential release, and emerging toxicological concerns. In: Snedeker SM, editor. Toxicants in food packaging and household plastics. London: Springer; p. 95–123.
- Piringer O. 2007. Mathematical modelling of chemical migration from food contact materials. In: Barnes KA, Sinclar RC, Watson DH, editors. Chemical migration and food contact materials. Cambridge: Woodhead; p. 180–202.
- Piringer O. 2008. A uniform model for prediction of diffusion coefficients with emphasis on plastic materials. In: Piringer OG, Baner AL, editors. Plastic packaging: interactions with food and pharmaceuticals. 2nd ed. Weinheim: Wiley-VCH Verlag GmbH & Co. KGaA; p. 163–193.
- Poças MF, Oliveira JC, Oliveira FAR, Hogg T. 2008. A critical survey of predictive mathematical models for migration from packaging. Crit Rev Food Sci Nutr. 48:913–928.
- Ramos K, Gómez-Gómez MM, Cámara C, Ramos L. 2016. Silver speciation and characterization of nanoparticles released from plastic food containers by single particle ICPMS. Talanta. 151:83–90.
- Research and Markets. 2015. Global food packaging market 2015–2019. [Internet]. [ cited 2016 Sep 12]; p. 74. Available from: http://www.researchandmarkets.com/research/dnz2z7/global_food
- [SCENIHR] Scientific Committee on Emerging and Newly Identified Health Risks. 2009. Risk assessment of products of nanotechnologies. Brussels: European Commission.
- Schmidt B, Katiyar V, Plackett D, Larsen EH, Gerds N, Bender Koch C, Petersen JH. 2011. Migration of nanosized layered double hydroxide platelets from polylactide nanocomposite films. Food Addit Contam Part A. 28:956–966.
- Schmidt B, Petersen JH, Bender Koch C, Plackett D, Johansen NR, Katiyar V, Larsen EH. 2009. Combining asymmetrical flow field-flow fractionation with light-scattering and inductively coupled plasma mass spectrometric detection for characterization of nanoclay used in biopolymer nanocomposites. Food Addit Contam Part A. 26:1619–1627.
- Simon P, Chaudhry Q, Bakoš D. 2008. Migration of engineered nanoparticles from polymer packaging to food – a physicochemical view. J Food Nutr Res. 47:105–113.
- Sinha SK, Chong WLM, Lim S-C. 2007. Scratching of polymers—modeling abrasive wear. Wear. 262:1038–1047.
- Song H, Li B, Lin Q-B, Wu H-J, Chen Y. 2011. Migration of silver from nanosilver–polyethylene composite packaging into food simulants. Food Addit Contam Part A. 49:1–5.
- Tiede K, Boxall ABA, Tear SP, Lewis J, David H, Hassellöv M. 2008. Detection and characterization of engineered nanoparticles in food and the environment. Food Addit Contam Part A. 25:795–821.
- Vieira AC, Guedes RM, Tita V. 2014. Constitutive modeling of biodegradable polymers: hydrolytic degradation and time-dependent behavior. Int J Solids Struct. 51:1164–1174.
- von der Kammer F, Legros S, Hofmann T, Larsen EH, Loeschner K. 2011. Separation and characterization of nanoparticles in complex food and environmental samples by field-flow fractionation. Trac Trends Anal Chem. 30:425–436.
- von Goetz N, Fabricius L, Glaus R, Weitbrecht V, Günther D, Hungerbühler K. 2013. Migration of silver from commercial plastic food containers and implications for consumer exposure assessment. Food Addit Contam Part A. 30:612–620.
- Witzler M, Küllmer F, Hirtz A, Günther K. 2016. Validation of Gold and Silver Nanoparticle Analysis in Fruit Juices by Single-Particle ICP-MS without Sample Pretreatment. J Agric Food Chem. 64:4165–4170.
- Wyser Y, Adams M, Avella M, Carlander D, Garcia L, Pieper G, Rennen M, Schuermans J, Weiss J. 2016. Outlook and challenges of nanotechnologies for food packaging. Packag Technol Sci. 29:615–648.
- Yohannes G, Wiedmer SK, Elomaa M, Jussila M, Aseyev V, Riekkola M-L. 2010. Thermal aggregation of bovine serum albumin studied by asymmetrical flow field-flow fractionation. Anal Chim Acta. 675:191–198.