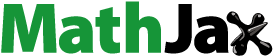
ABSTRACT
For improved wound healing, antimicrobial adhesives are one path forward. However, with the current challenge of bacterial resistance, it is essential to choose the included drug carefully. Octenidine is an obvious choice due to its broad antimicrobial efficacy and no reported bacterial resistance. In its pure form, octenidine complexes efficiently with the platinum catalyst in the silicone composition, inhibiting the targeted hydrosilylation reaction and hindering curing. This obstacle is overcome by screening octenidine with cyclodextrins in homogeneously dispersed glycerol droplets, suppressing Pt inhibition in the silicone phase. Curing efficiency is demonstrated using rheology, which shows that it is possible to incorporate one wt% of octenidine into glycerol–silicone adhesives in the presence of (2-hydroxypropyl)-β-cyclodextrin without affecting the adhesives’ mechanical properties. The interaction between octenidine and (2-hydroxypropyl)-β-cyclodextrin through an inclusion complex is confirmed by ROESY spectroscopy. Despite this screening, octenidine is still released efficiently from the glycerol–silicone adhesives upon contact with water, and the resulting antimicrobial action is subsequently demonstrated. This new technology constitutes a simple and efficient method for preparing wound care adhesives that actively inhibit the growth of four bacteria strains and one fungus.
GRAPHICAL ABSTRACT
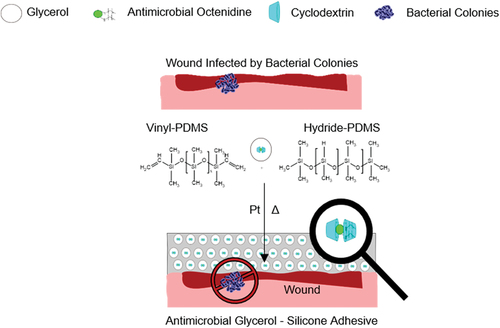
1. Introduction
Chronic wounds, such as those often found in elderly and diabetic patients, result from anomalies in cellular and molecular wound repair mechanisms [Citation1]. Specially designed materials are required to promote correct wound healing and closure in these patients, among which skin adhesives are of significant current research interest. Broadly, a skin adhesive must allow moisture transport to avoid skin maceration due to the presence of excess liquids (mainly sweat and exudate) while still maintaining a humid environment in the wound [Citation2,Citation3]. In addition, the ability to reduce or prevent bacterial infections would be a valuable feature in skin adhesives for chronic wounds. There are several reasons why bacteria and fungi are particularly unwanted in healing wounds: Bacterial or fungal infections lead to the formation of biofilm. Biofilm consists of dormant bacteria that act as a protective layer around the bacteria, making them less receptible to antibiotics and the immune response. This makes it extremely difficult for the body to heal the wound when an infection occurs [Citation4]. Furthermore, the presence of bacteria and fungi triggers the immune system and may thus lead to inflammation. Continued inflammation caused by infection may interrupt tissue regeneration. Infections can furthermore lead to delayed granulation tissue formation and impaired collagen synthesis, which delays wound closure and healing. Moreover, several specific bacteria, such as Staphylococcus aureus and Pseudomonas aeruginosa [Citation5], and fungi, such as the candida species [Citation6], may cause chronic wounds, which are wounds that fail to heal within a certain time frame and may last for years due to the infection and accompanying inflammation that prevents the wound from healing. Chronic wounds are currently a billion-dollar health problem that affects up to 45 million people worldwide, especially the elderly and diabetic patients [Citation7].
Common wound dressing materials include polyurethane foams, hydrogels, hydrocolloids, and alginates. More advanced wound dressings have recently emerged, including microneedle patches [Citation8], 3D fibrous scaffolds [Citation9,Citation10], 3D porous aerogels [Citation11], and a large number of 3D-printed dressings made from a variety of materials such as hydrogels [Citation12,Citation13], chitosan [Citation14], polycaprolactone [Citation15], polyurethane [Citation16], and PDMS with and without embedded actives [Citation17].
Our previous work thoroughly described a new glycerol–silicone hybrid elastomer adhesive with significantly improved fluid handling properties and increased oxygen permeability [Citation18]. These elastomers consist of a bi-continuous or closed-cell structure, depending on the glycerol content. The elastomers are prepared using a simple processing method that consists of mixing silicone prepolymers and glycerol under high shear forces. After curing, these materials are free-standing and stable two-phase elastomers with inherent liquid glycerol droplets. SEM images of these materials can be found in Mazurek et al. [Citation19] The materials can be processed as thin films, bulk elements, or foams. The adhesive performance, evaluated in terms of mechanical properties, peel, and tack, was thoroughly investigated by Chiaula et al. [Citation18]
The silicone prepolymers are, during curing, reacted to form silicone elastomer through a hydrosilylation reaction [Citation18]. Hydrosilylation-cured silicone elastomers are widely used in industry—e.g. as biomedical devices and medical skin adhesives [Citation20–22]. In general, hydrosilylation curing is a reliable method for preparing elastomers, as the reaction between the hydride and vinyl groups proceeds with high conversion and limited side reactions [Citation23–26]. Nevertheless, preventing the inhibition or poisoning of the platinum (Pt) catalyst used in the hydrosilylation curing reaction remains a challenge in certain cases. In general, all substances that complex Pt with an efficiency comparable to or higher than that of the vinyl groups are potential inhibitors, since hydrosilylation curing requires a labile Pt atom complexed to the vinyl group of the silicone [Citation23]. For example, sulfur-, phosphorus-, and nitrogen-containing compounds are all inhibitors, as they form competitive, irreversible complexes with Pt, resulting in slowed or unsuccessful curing [Citation23,Citation27].
The healthcare industry is particularly interested in silicone adhesives with topical antimicrobial properties. Among topical antimicrobials, silver has been known for decades to prevent microbial growth and, more recently, has been incorporated directly into silicone dressings [Citation28–30]. Nevertheless, if in close contact to skin for an extended time, silver might be absorbed and lead to argyria, a local or systemic deposition of this metal in the skin itself [Citation31]. Chlorhexidine and triclosan are other commonly used antimicrobials. However, their extensive use is associated with antibiotic resistance and collateral effects, such as severe rashes and breathing difficulties [Citation32,Citation33]. Alternative antimicrobial products are therefore needed.
Octenidine () is a positively charged bis-pyridinamine with a broad spectrum of antibacterial (both toward Gram-Positive and Gram-Negative bacteria) and antifungal properties – as well as some antiviral ones – and is capable of inhibiting biofilm formation [Citation35,Citation36]. Additionally, octenidine has high biocompatibility, no known bacterial resistance, and is currently used as a wound cleansing agent and topical antiseptic [Citation37,Citation38]. Because its chemical structure includes long alkyl chains and two amine groups, octenidine’s direct incorporation into silicone-based skin adhesives is not trivial, since the combination of alkyl chains and amine groups renders it amphiphilic and the amine groups inhibit the hydrosilylation reaction. A new strategy to allow octenidine incorporation into silicone adhesives is thus required.
Figure 1. Schematic illustration of the preparation method for glycerol–silicone elastomer adhesives with active substances [Citation19,Citation34]. a) Chemical structure of the guest molecule octenidine. b) chemical hosts β-cyclodextrin (β-CD) and (2-hydroxypropyl)-β-cyclodextrin (HPβCB).
![Figure 1. Schematic illustration of the preparation method for glycerol–silicone elastomer adhesives with active substances [Citation19,Citation34]. a) Chemical structure of the guest molecule octenidine. b) chemical hosts β-cyclodextrin (β-CD) and (2-hydroxypropyl)-β-cyclodextrin (HPβCB).](/cms/asset/182accd0-1e5d-4f41-8b33-f88b19b284b9/tsnm_a_2240743_f0001_c.jpg)
The use of glycerol–silicone elastomers as drug delivery systems was thoroughly described by our group in Mazurek et al. [Citation19], who successfully demonstrated that glycerol domains act as reservoirs for small, hydrophilic molecules. Cyclodextrins are well known to have the ability to increase the aqueous solubility and stability of drugs with poor water solubility via the formation of inclusion complexes [Citation39,Citation40]. In addition, cyclodextrins are known to improve drugs’ chemical stability, and in some cases to improve their efficiency [Citation41,Citation42]. Due to the chair conformation of the glucopyranose units, cyclodextrins attain the shape of a truncated cone (), resulting in a hydrophobic central cavity and a hydrophilic outer surface [Citation43]. This particular conformation allows cyclodextrins to host hydrophobic molecules inside their cavity, improving their aqueous solubility.
In this work, we describe a new method for incorporating octenidine into glycerol–silicone elastomer adhesives via cyclodextrins. Specifically, we demonstrate a method for efficiently curing glycerol-silicone hybrid adhesives through hydrosilylation in the presence of octenidine via the formation of inclusion complexes, as demonstrated by ROESY spectroscopy. We find that cyclodextrins suppress the interference of octenidine with the Pt catalyst. We further demonstrate these adhesives’ ability to release octenidine, enabling the preparation of silicone adhesives with antimicrobial properties.
2. Experimental
2.1 Materials
A commercially available, two-part system (part A and part B) hydrosilylation curing soft silicone (medical grade) adhesive kit was used (MG7–9900 DowDuPont Inc. ™). Medical grade glycerol was provided by AppliChem GmbH (DE). β-Cyclodextrin (βCD), (2-hydroxypropyl)-β-cyclodextrin (HPβCD), perylene (synthesis grade), and sulforhodamine B acid chloride (technical grade) were acquired from Sigma-Aldrich (DK). Octenidine dihydrochloride (Oct) was purchased from Dishman Group (UK). Gram-Positive bacteria strains Pseudomonas aeruginosa (ATCC 27,853) and Escherichia coli (ATCC 53,498), Gram-Negative bacteria strain Staphylococcus aureus, (ATCC 25,923) and the fungus Candida albicans (ATCC 10,231) were purchased from Miclev (SE). Staphylococcus epidermidis (NCTC 11,047) was purchased from Sigma Aldrich. Lecithin was purchased from VWR Chemicals (DK). Coloplast A/S (US) provided commercial products containing silver (Mepitel Ag). Polyethylene terephthalate (PET) and polyurethane (PU) backing films were purchased from Mitsubishi Polyester Film (DE) and Coveris (UK), respectively. A fluorinated ethylene propylene (FEP) release liner was purchased from Lohmann (UK). All components were used as received.
2.2. Methods
2.2.1 Sample preparation
The two parts of the commercial soft silicone adhesive kit were weighed off in a 1:1 ratio, according to the manufacturer’s instructions. The desired amount of glycerol was subsequently added to the silicone adhesive premix. The abbreviation ‘phr,’ used to describe glycerol content in all compositions, corresponds to glycerol weight per hundred parts of silicone adhesive: e.g. 20 phr means that 20 g of glycerol was used per 100 g of silicone adhesive premix.
For samples containing cyclodextrins (CDs) and octenidine (Oct), the mass of Oct was set at 1 wt% of the combined mass of adhesive premix and glycerol in all experiments. The amount of CDs was adjusted according to the desired CD:Oct molar ratio; mixtures were prepared in molar ratios ranging from 0.25 to 4 and dissolved in glycerol at 80°C using magnetic stirring until clear solutions were obtained. The molar ratio r is defined as:
The desired amount of glycerol containing CD:Oct mixture was subsequently added to the silicone adhesive premix. Sample names were formed using the patterns GX_Y, where ‘G’ and ‘X’ stand for glycerol and glycerol phr, respectively. ‘Y’ accounts for various parameters discussed in the following sections. Occasionally, ‘Y’ will be followed by a number, which stands for the molar ratio between CD and Oct. A full description of all investigated samples can be found in Table S1 in the Supplementary Information.
The mixtures (silicone and glycerol, or silicone and glycerol containing CD:Oct) were stirred for a total of 2 min: 1 min by hand-mixing with a spatula, followed by 1 min at 3500 rpm with a dual asymmetric centrifuge SpeedMixer DAC 150 FVZ-K (DE). No additional degassing of the formulations was necessary. The obtained glycerol-in-silicone emulsions were coated onto a PET backing film with a commercial knife (gap size of 0.4 mm) and an RK K Control Coater with a speed of 23 mm/s to obtain adhesives approximately 0.3 mm thick. The samples were subsequently cured at 80 ± 1°C for 1 h. All samples were covered with an FEP release liner after coating; this liner was removed before any measurement took place. Adhesive thicknesses were measured prior testing between two liners with pre-determined thicknesses using a Mitutoyo digital thickness gauge (DE).
2.2.2 Curing profiles of glycerol–silicone adhesives and linear viscoelastic measurements
Curing profiles of glycerol–silicone adhesives containing different CD:Oct molar ratios were obtained by time sweep tests at 80°C for 10 min on a controlled stress-strain ARES G2 rheometer (TA Instruments), using a parallel plate geometry 25 mm in diameter. The instrument was set to a controlled strain mode ensured to be within the linear regime. Frequency and strain were set at 1 Hz and 2%, respectively. The gap between the plates was set to approximately 1 mm. Frequency sweeps were subsequently performed on the cured adhesives from 100 to 0.1 Hz at 32°C to evaluate the linear viscoelastic properties of the cured adhesives.
2.2.3 Morphology of glycerol–silicone adhesives
A Leica TCS SP5 X confocal microscope was used to investigate the morphology of cured composites. The composites were labeled with differently colored dyes in the manufacturing process: sulforhodamine B (0.1 wt% relative to glycerol) for the glycerol phase and perylene (10 µL of a perylene solution in isopropanol 2.53 mg/mL) for the silicone phase. To determine the average size distribution of the spherical glycerol droplets in the formulations, droplet diameters were measured by counting the droplets directly from microscopy pictures using ImageJ software.
2.2.4 NMR studies
For NMR studies, HPβCD:Oct samples (r = 2) were dissolved in D2O in a concentration of 20 mg/mL. All NMR spectra were acquired using a Bruker 300 MHz spectrometer at 293 K. For 1D 1H NMR, 16 to 128 scans were collected. 2D Rotating frame Overhauser Effect SpectroscopY (ROESY) spectra were collected using a mixing time of 200 ms for detection of intermolecular nuclear Overhauser effects (NOEs). Sixteen scans were collected for each spectrum. The data were analyzed using TopSpin version 3.5 pl 7 from Bruker. The 1H NMR prediction software package in ChemOffice 2016 was used as an aid in assigning peaks.
2.2.5 Release profiles of glycerol–silicone adhesives
Oct release profiles from glycerol–silicone adhesives were obtained by immersing specimens containing various amounts of glycerol in deionized water. Measurements were performed at room temperature. Oct release was monitored by measuring the changes in Oct concentration of the aqueous solution. A UV–vis spectrophotometer POLARstar Omega microplate reader (BMG LabTech) was used for the tests, and measurements were translated into concentrations using a calibration curve for aqueous HPβCD:Oct solutions. Oct quantification was performed at 280 nm. A representative UV–vis spectrum for Oct showing a peak maximum at 280 nm can be found in [Citation44]. CDs did not show any absorbance peak in the UV–vis spectrum. The UV–vis spectrum of β-CB is shown in Figure S6 in supporting information.
2.2.6 Antimicrobial properties of adhesives
Antimicrobial tests on glycerol–silicone adhesives containing HPβCD:Oct mixtures (r = 2) were performed using a modified version of the ISO 22,196 method. P. aeruginosa (ATCC 27,853) and E. coli (ATCC 53,498) bacteria strains were chosen for the tests of Gram-Negative bacteria and grown in a 0.9 wt% NaCl solution. S. aureus (ATCC 25,923) and S epidermis (NCTC 11,047) bacteria strains were chosen for the tests of Gram-Positive bacteria and grown in a 0.9 wt% NaCl solution. Two hundred microliters of bacteria-containing solution was then placed on the bottom of a cell culture plate, and discs of cured adhesive (diameter 34 mm, thickness 0.3 mm) were put in contact with the liquid. Samples without HPβCD:Oct and a commercial product containing silver, Mepitel Ag, were used as controls. Only in the case of Gram-Negative bacteria was the commercial Mepitel used. Tests were run in triplicate for each sample. Samples in direct contact with bacterial solutions were allowed to incubate for 24 h in a humid environment. After incubation, the samples were washed with 1.8 mL of 2 wt% lecithin solution to neutralize Oct activity (according to the US Pharmacopeia). Samples of the liquid were then plated onto agar and incubated for 18–26 h at 35°C (medium temperature between body temperature (37°C) and skin temperature (32°C)), and colony forming units (CFU) were counted manually. Microbial concentrations were determined using a Biochrom WPA CO8000 Cell Density Meter. The reduction of microorganisms relative to initial concentrations was calculated and expressed in terms of ‘Log10 CFU.’ A description of the expressions to determine ‘Log10 CFU’ is provided in the Supplementary Information.
3. Results and discussion
3.1 Investigation of the best molar ratio CD:Oct through the evaluation of the curing profiles of glycerol–silicone adhesives
Oct contains two amine groups () and is well known to inhibit hydrosilylation curing. A novel synthesis strategy is therefore needed that does not include toxic components, such as the less sensitive tin catalyst. One possibility is to add Oct in a different phase than the Pt catalyst, which is possible with glycerol–silicone adhesives. However, even if Oct is predominantly in the glycerol phase, its presence at the glycerol–silicone interface inhibits the curing of the silicone phase. For this reason, we used CDs to screen the Oct from the Pt. Two different CDs were investigated here: βCD and HPβCD (). The influence of the CD:Oct ratio on gelation time (transition from liquid to solid) and elastic modulus after full cross-linking was investigated to find the optimal concentration of CD in the system.
Glycerol–silicone adhesives’ gelation time and elastic modulus after curing are reported in as a function of CD:Oct ratio. Adhesives containing up to 50 phr of glycerol were selected, as we in an earlier study thoroughly examined for which compositions there were no cohesive failure modes from various substrates [Citation18]. The samples are compared to the corresponding complex-free compositions, i.e. with no CD or Oct. The curing profiles of pure glycerol–silicone adhesives are shown in Figure S1 (Supplementary Information). As expected, the pure glycerol–silicone adhesives have different gelation times due to their different volume fractions of elastically active material. The pure silicone adhesive reaches gelation in 100 s, and gelation time is shortened when the glycerol content is increased. This is due to the fact that glycerol droplets in the soft silicone adhesive premixes contribute to elasticity in the liquid state due to their interfacial energies, introducing a so-called solid stiffening effect [Citation18,Citation45]. In principle, the larger the number and smaller the size of the glycerol droplets, the greater the elastic contribution. The cross-over over G’ and G’’, which is commonly used as measures of gelation time [Citation24,Citation46], is reached before the true gelation of the silicone phase due to the elastic contribution from the interfaces. The curing profile of G50 is made from a different batch of silicone adhesive, and the gelation time is therefore not consistent with the remaining data. An even lower gelation time would be expected if these samples were from the same adhesive batch.
Figure 3. Investigation of the optimum CD:Oct ratio through evaluation of gelation times and elastic moduli after full cross-linking. Measurements were conducted in the linear viscoelastic regime at 2% strain. All data was obtained from the curing profiles at 80°C at a frequency of 2 Hz. a) Gelation times of glycerol–silicone adhesives as a function of CD:Oct molar ratio and as a function of the glycerol amount. Horizontal lines correspond to gelation times of the complex-free, i.e. with no CDs or Oct, reference compositions. b) Elastic moduli of glycerol–silicone adhesives as a function of CD:Oct molar ratio and as a function of glycerol amount. Horizontal lines correspond to the elastic moduli of the reference compositions.
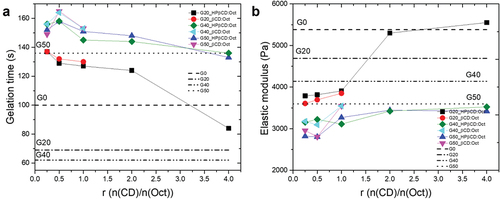
Gelation times were found to decrease and elastic moduli to increase with increasing CD:Oct ratio (). When r = 2 and 4, gelation times and elastic moduli are comparable to or higher than those of the corresponding complex-free compositions. In particular, the elastic modulus of G20 increases from approximately 4000 Pa to over 5000 Pa when r = 2 and 4 (). Oct amounts are always 1 wt% of overall mass. The mass of silicone decreases from G20 to G50 due to dilution with glycerol, but the Pt:silicone ratio remains constant for all compositions. However, this means that the Pt:Oct ratio is lower in compositions with higher glycerol content, which also have increased surface areas (the effect of glycerol surface area on mechanical properties is discussed in detail in section 3.3). HPβCD allows for the formation of CD:Oct complexes with r up to 4, while βCD only allows complex formation for r up to 1 due to its lower solubility in glycerol compared to HPβCD. As shows, higher CD:Oct ratios allow adhesives to reach elastic moduli comparable to those of the corresponding reference samples. Importantly, the elastic moduli of adhesives containing CD:Oct ratios of 2 and 4 are comparable. This indicates that Pt inhibition is sufficiently suppressed at the lower ratio to ensure efficient cross-linking. Further increasing of CDs content only changes the apparent gelation time due to the higher initial G’ of the premixes. To better understand the interaction between CDs and Oct at one of the favorable ratios – which, as mentioned previously, can only be achieved with HPβCD – a model NMR study of the system HPβCD:Oct with r = 2 was conducted using ROESY spectroscopy in the following section. The system HPβCD:Oct with r = 2 was preferred over the system with r = 4 due to the lower viscosity of the initial mixture and, therefore, higher simplicity in the overall manufacturing process.
3.2 2D ROESY of HPβCD:Oct complexes
Guest-host complexes between CDs and various guest molecules are examined using spectroscopic techniques such as NMR [Citation47], which enable determination of the complexation constant and complex stoichiometry. However, the characterization of HPβCD:Oct complexes is potentially more complicated than that of other CD:drug systems for several reasons: in order to suppress chemical shift effects from surfactant micellization, complex formation should ideally be analyzed below the critical micelle concentration (CMC) [Citation48,Citation49], which is reported to be 3.79 mM for Oct [Citation37]. Due to NMR’s relatively low sensitivity, however, getting reliable measurements at such low concentrations requires a specific instrumental setup. In addition, there are reports that imply that structurally similar gemini surfactants may form complexes with either one or two CDs [Citation49], which would invalidate the use of the common Job’s plot for determining complex stoichiometry [Citation50]. More importantly, NMR studies of HPβCD are complicated by the fact that this molecule consists of a number of closely related isomers, which gives the resulting spectrum relatively broad features rather than well-defined sharp peaks [Citation51].
Despite these complications, NMR was used to provide further details on the complexation between Oct and HPβCD (r = 2) in deuterated water (D2O). Initially, 1D 1H NMR and 2D COSY spectra were acquired separately for Oct and HPβCD (). As reported, the 1H NMR spectrum of HPβCD exhibits several broad features between 3 and 4.5 ppm that do not allow for the exact assignment of individual protons [Citation51]. On the other hand, the 1D 1H NMR 2D COSY spectra of HPβCD:Oct in D2O allow full assignment of several protons for this compound (). In particular, both aromatic protons and methylene protons α, β, and ω to the nitrogen atoms can be assigned based on the combination of COSY, comparison to known compounds, and chemical prediction software. The assigned structure is given in .
Figure 4. Characterization of HPβCD:Oct 2:1 mixture by 1H NMR. a) Assigned structure of Oct. b) 1H NMR spectrum of HPβCD:Oct (r = 2) mixture in D2O. c) Enlarged region of ROESY spectrum emphasizing through-space (NOE) interaction between aromatic Oct signals and cyclodextrin. d) Enlarged region of ROESY spectrum emphasizing through-space.
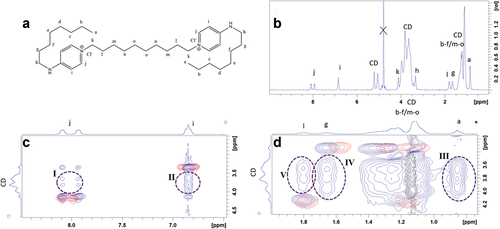
The complexation between Oct and HPβCD was investigated using ROESY spectroscopy, a technique which exploits the nuclear Overhauser effect (NOE) to detect through-space interactions between protons [Citation52]. The signal intensity scales with distance to the minus sixth (r−6) and is therefore highly sensitive to the average distance between protons. In practice, however, distances above 4 Å do not give rise to any signal [Citation47]. shows the ROESY spectrum of a HPβCD:Oct mixture with r = 2 in blue. This is superimposed on ROESY spectra of Oct (red) and HPβCD (black) in order to visualize additional signals in the mixture, indicating little distance between the two molecules.
Due to the broad signals that HPβCD generates, it is impossible to determine exactly which protons in the CD interact with Oct—i.e. whether the interaction is associated with cavity protons or external protons – limiting the possibility of exactly elucidating the complex structure. However, several conclusions can be drawn from the highlighted interaction regions in . In particular, protons a (region III), g (region IV) and i (region II) show greater interaction with HPβCD than protons l (region V, compared with region IV) and j (region I, compared with region II). Protons a and g are assigned to the terminal chains and protons i are ortho to the terminal chain, whereas protons l are assigned to the central chain and protons j are ortho to the polar pyridinium group. These results indicate that the terminal chains are particularly associated with the hydrophobic HPβCD cavity and that the aromatic ring constitutes the interface with the exterior, with the charged group being stabilized by the more polar environment. These findings are consistent with reports on complexes between structurally related gemini bis-(dodecyl dimethylammonium)diethyl ether dibromide surfactants and βCDs [Citation49]. Due to the lack of fine structure in the spectral features of HPβCD, the ROESY spectrum cannot determine whether one or two HPβCDs complex to the Oct. However, previous work on related gemini complexes has indicated that complexes with both one and two CDs are possible, corresponding to one or both terminal chains embedded in the hydrophobic part of the CD [Citation49]. Complexes containing four βCDs were also reported for a naphthalene-containing cationic gemini surfactant [Citation53].
While elucidating the exact complex structure is beyond the scope of this work, the ROESY spectra do clearly indicate that Oct’s aniline groups interact strongly with the HPβCD. This interaction should suppress competitive complexation with the Pt catalyst, thereby suppressing inhibition of the polymerization process. Importantly, however, this argument is only valid if the HPβCD:Oct mixture has a similar structure in glycerol. Unfortunately, the acquisition of high-quality ROESY spectra in glycerol was not feasible due to the high viscosity of this solvent combined with a significant overlap between residual solvent signal and HPβCD. However, based on the observation that the solubility of Oct in glycerol is significantly increased in the presence of HPβCD, as well as the results from the curing experiments described in , it can be assumed that similar complexation occurs in this solvent.
3.3 Evaluation of the linear viscoelastic properties of glycerol–silicone adhesives containing CD:Oct mixtures
To investigate how the incorporation of CD:Oct into glycerol–silicone adhesives affects their mechanical behavior, shear rheology in the linear regime was performed. The full spectra of storage moduli (G’) and viscous moduli (G’’) are shown in Figures S2 and S3 in the Supplementary Information. Dissipation factors (tan δ = G’’/G’) are shown in as a function of both CD:Oct ratio and glycerol content (as a reference).
Figure 5. Linear viscoelastic properties of adhesives. Measurements were conducted with controlled strain at 2% and frequency sweeps from 100 Hz to 0.1 Hz at T = 32°C. a) Tan δ of glycerol–silicone adhesives containing 20, 40 and 50 phr, respectively, compared to a pristine silicone adhesive sample. b-d) Tan δ of G20, G40, and G50 adhesives, respectively, containing CD:Oct mixture with r ranging from 0.25 to 4.
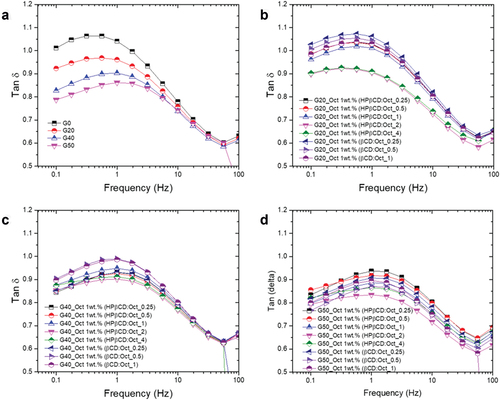
Counterintuitively, shows that tan δ decreases across the entire frequency range when liquid content increases. In other words, the composites become more solid with increased liquid content. Such behavior has previously been observed for glycerol–silicone adhesives with increasing glycerol content [Citation18] and can be explained by the fact that the cross-linked emulsions have two distinct elastic components: the elasticity arising from the silicone network itself, and the interfacial energy between the two phases. Style et al. and the extended Eshelby theory previously described this so-called solid-stiffening effect caused by the glycerol droplets in soft silicone elastomers. They demonstrated that surface tension helps to keep liquid inclusions spherical, opposing any applied force that would make them elliptical [Citation45,Citation54]. Furthermore, the mechanical response of an isolated liquid inclusion depends on its size. In particular, when comparing two soft elastomers with the same interfacial area, the one with smaller droplets is the stiffer of the two. According to the extended Eshelby theory, the length l and the width w of a stretched droplet embedded in an elastic solid can be calculated via the equations given below [Citation45,Citation55–57]:
and
R is the radius of the droplet, and
are the applied far-field plane-stress boundary conditions for which
,
and
, x and y are the strain directions, and
is the Poisson’s ratio; γ is the surface tension of the droplet and E is the Young’s modulus of the solid. If γ/ER
1, the effect of the surface tension which keeps the droplets spherical is predominant. The effect of CDs incorporated into glycerol–silicone adhesives is investigated further in . A higher elastic response – i.e. a lower tan δ—is observed for adhesives containing HPβCD:Oct mixtures with r > 1, indicating that a change in the interfacial energies has occurred. To investigate whether the presence of HPβCD affects the size of the glycerol droplets, the adhesives’ morphology was analyzed. Confocal microscopy images of cross-sections of cured adhesives containing a HPβCD:Oct mixture with r = 2 are shown in . The relative internal glycerol surface area per adhesive volume is shown in . The viscous elastic losses, usually referred to via the loss tangent (tan δ), were measured by linear rheology and calculated as tan δ = G’’/G’, where G’’ is the measured loss modulus and G’ is the measured storage modulus. The results of tan δ as function of the glycerol amount in phr are shown in .
Figure 6. a) Confocal microscopy pictures of cured glycerol–silicone adhesives without (top) and with (bottom) HPβCD:Oct mixtures. All compositions with HPβCD:Oct contain 1 wt% of Oct and r of 2. Scale bars correspond to 100 µm. b) Glycerol surface area per adhesive volume with and without HPβCD:Oct (r = 2) as a function of glycerol loading. c) Tan δ at 1 Hz of adhesives with and without HPβCD:Oct (r = 2) as a function of glycerol loading. Measurements were conducted with controlled strain of 2% at 32°C.
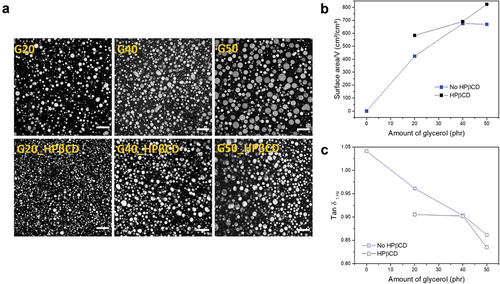
Confocal image analysis confirms that the average droplet diameter decreases for samples containing HPβCD:Oct compared to their pure counterparts, resulting in a greater glycerol surface area per volume (). This is not surprising, as CDs are known to cause a stabilizing effect in emulsions [Citation58,Citation59]. In addition, adhesives containing HPβCD possess lower tan δ compared to pure compositions (), most likely due to their increased interfacial area.
To better understand how interfacial energies affect elasticity, we further analyzed the adhesives’ dynamics. The characteristic frequency, ωmax, at which , is shown for pure glycerol–silicone adhesives and for drug-containing ones in respectively. For pure glycerol–silicone adhesives, ωmax increases in correlation to glycerol loading. In other words, the relaxation dynamics within the adhesive speed up when glycerol loading increases, while overall elasticity is also increased. Such behavior contradicts classical rubber elasticity theories in which dynamics slow down with increasing elasticity, emphasizing the importance of interfacial contributions to elasticity for these very soft materials. Indeed, these findings indicate that interfacial energies contribute significantly to the higher mobility observed at the glycerol–silicone interface compared to in the adhesive bulk. The characteristic frequency is not affected in the same manner for adhesives containing CD:Oct mixtures, and remains constant for G40 and G50 regardless of CDs content (). On the other hand, a drop in characteristic frequency is observed for G20 compositions with high CDs content, indicating that the CDs interact with the glycerol–silicone interface, either by changing the interfacial energy or by physically hindering free motion of the polymers at the interface. To better understand the influence of interfacial energies on adhesive elasticity, as well as how these energies change when CDs are introduced, the Maxwell relaxation time, τmax, can be used. The Maxwell model defines the characteristic time by defining tan δmax as [Citation60]:
Figure 7. a-b) Characteristic frequency, ωmax, as a function of glycerol loading and CD:Oct ratio, respectively. c-d) Characteristic relaxation times, τmax, determined from the Maxwell model as function of the glycerol surface area per volume adhesive without (c) and with HPβCD:Oct (r = 2) (D), respectively.
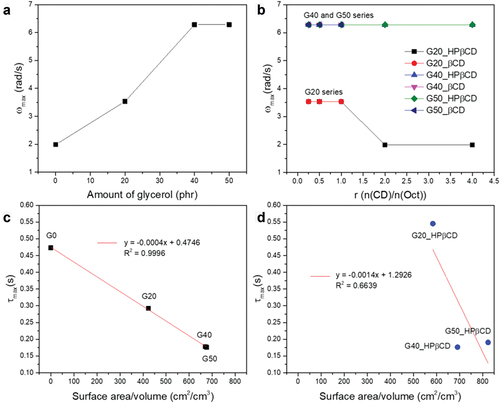
Where tan δmax and are determined as described earlier, and τmax is determined and plotted against surface area per volume in . While τmax scales linearly with surface area per volume for pure glycerol–silicone adhesives (), the same linearity is not achieved for compositions containing CD:Oct mixtures (), further suggesting that CDs and/or drugs interact with the interface. Since curing of the silicone adhesive was made possible by the efficient screening described previously, it must be the CDs that interact with the interface to reduce interfacial energies, also leading to the smaller elastic contribution observed for the samples, particularly the adhesives with HPβCD ().
3.4 Release profiles of oct from glycerol–silicone adhesives and antimicrobial activity
As reported in our previous work, glycerol–silicone composites can absorb significant amounts of water when coming into contact with an aqueous phase due to the hygroscopic nature of glycerol. Thereby, the volume of the droplets inside the silicone phase increases. Glycerol and any compounds in the glycerol phase are thus released from the droplets, following zero- or first-order kinetics [Citation18,Citation19,Citation61]. It is therefore expected that the substances investigated herein, which are incorporated into the glycerol domain reservoirs, will be released from the silicone matrix. Release profiles of Oct complexed with HPβCD at a ratio of r = 2 from glycerol–silicone adhesives with different glycerol contents are shown in . The corresponding release rates are plotted against glycerol content in . The release of active compounds is measured at room temperature since the effect of temperature on the release kinetics is negligible. The results indicate that Oct is released faster from adhesives with higher glycerol content, in agreement with previously published findings for elastomers. The apparent decrease of the cumulated release of G50 is due to a relatively large measurement uncertainty.
Figure 8. a) Oct release profiles for G20, G40 and G50 glycerol–silicone adhesives with HPβCD:Oct ratio equal to 2. b) Release rate dependence on glycerol content. c-d) Bacteriological results of ISO 2219 tests and ‘Log10CFU’ of the commercial samples and G50_Oct 1 wt% (HPβCD:Oct with r = 2), respectively. The adhesive samples were in direct contact with 200 µL of bacteria solutions containing P. aeruginosa and E. coli, respectively, for 24 h. Visible colonies appeared on both G50 samples without Oct and the commercial samples exposed to bacteria for 24 h after the liquid samples were plated onto agar. No colonies were visible after bacteria exposure of G50_Oct 1 wt% (HPβCD:Oct with r = 2). e-f) Histograms representing the antibacterial activity, represented as ‘Log10 CFU,’ of G50, Mepitel Ag and G50_Oct 1 wt% (HPβCD:Oct with r = 2), respectively, against P. aeruginosa (blue) and E. coli (green).
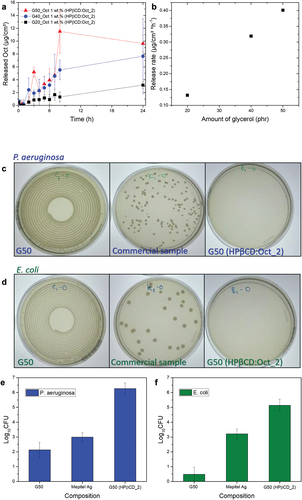
Adhesives were subsequently tested for antimicrobial activity against P. aeruginosa and E. coli bacteria strains. Both pathogens appear relevant candidates for this indicative study as they are commonly found in wound infections and are difficult to eliminate [Citation62–64]. For applications in real wounds, a broader spectrum of fungi, as well as both Gram-Positive and Gram-Negative bacteria, should be investigated. The commercial antibacterial adhesive, Mepitel Ag, was used as a control. Silicone dressings containing silver are currently the most common antimicrobial products, and therefore new antimicrobial silicone dressings must have an antibacterial activity at least comparable to that of the silver-based product.
A screening study showed no antimicrobial activity of G20 samples, most likely due to too slow drug release compared to the growth rate of the investigated bacteria. Therefore, stable compositions with the highest possible glycerol content and, thus, the highest drug release rate – G50 – were chosen as candidates for the antimicrobial activity study. Bacterial colony growth on agar plates is shown in . While large Gram-Negative bacterial colonies were present for both the pristine G50 sample and the commercial sample containing silver, Mepitel Ag, no colonies were visible for G50 samples containing HPβCD:Oct with r = 2. For the modified G50 samples, the reduction of microorganisms relative to their initial amount, expressed as ‘Log10 CFU,’ was 6 for P. aeruginosa and 5 for E. coli, indicating efficient antimicrobial activity against both Gram-Negative bacteria strains (.
To show a greater versatility of the technology, also the antibacterial efficiency toward two Gram-Positive bacteria was investigated along with the antifungal properties toward one fungus. Data is shown in . For all three tests, the pure silicone-glycerol adhesive showed increased growth over time, whereas the compositions with Oct showed values of Log10 CFU between 4.5 and 5, indicating a reduction of the colonies by factors between 104.5 and 105.
Table 1. Antibacterial and – fungal activity data for gram-positive bacteria and fungus.
Changes in the mechanical properties and the solid-stiffening effect of the adhesives could also potentially influence antimicrobial activity [Citation65]. It has been shown that the attachment of bacteria to silicone adhesives with a 0.1 MPa Young’s modulus is approximately 99% higher compared to that of silicone elastomers with Young’s modulus of 2.6 MPa [Citation65]. However, in this study, the mechanical properties among glycerol–silicone adhesives remain relatively identical, and therefore, the mechanical properties are assumed not to alter the results. Therefore, this has not been further investigated in this paper since it requires extensive data to convolute the effect from the interrelated parameters, namely Young’s modulus, surface energies, and chemical effect.
4. Conclusion
We developed a novel antimicrobial glycerol–silicone adhesive capable of releasing Oct via CDs. Oct incorporation was achieved via CDs through formation of an inclusion complex with the drug. Rheological characterization of glycerol–silicone adhesives with varying CD:Oct ratios showed that HPβCD:Oct complexes with a ratio >1 allowed the incorporation of 1 wt% of Oct without compromising adhesives’ mechanical properties. The interaction between HPβCD and Oct via an inclusion complex was further characterized and confirmed using ROESY spectroscopy. Finally, we demonstrated the possibility of releasing Oct from glycerol–silicone adhesives and showed that a minimum release rate, obtained in G50 samples, is required to kill bacteria. Efficient antimicrobial activity against four bacteria and one fungus was achieved by G50 samples containing HPβCD:Oct in a molar ratio equal to 2. This shows that technology has great opportunities to deliver antimicrobial and – fungal capabilities to wound care products.
Due to their favorable properties, they display, together with their relatively simple preparation process, glycerol–silicone adhesives with Oct incorporated via CDs should be considered potential candidates for advanced wound care applications, in which it is necessary to effectively promote wound healing and prevent bacterial infection. A possible application of this adhesive is to act as a preliminary antibacterial agent in order to avoid potential biofilm formation before the effect of, e.g. an antimicrobial foam sets in. Furthermore, this wound dressing type, which is extremely soft due to the combination of the soft silicone and moisturizing glycerol, would be ideal for pediatric patients who often experience anxiety and discomfort during wound dressing changes. This adhesive would offer improved flexibility, less pain during wound dressing changes, and enhanced moisture absorption, which would significantly enhance the wound medical care for children.
As presented herein, this novel wound dressing type displays several promising properties such as soft antibacterial wound dressing. Further research is, however, needed to fully uncover the commercial potential of the material. Investigations into wound healing abilities in vitro and in vivo, biocompatibility, cytotoxicity, manufacturing scalability, and regulatory considerations will be conducted in the future.
Supporting information
Log reductions in antimicrobial testing; list of investigated adhesive samples; curing profiles of pure glycerol–silicone adhesives; evaluation of the linear viscoelastic properties of glycerol–silicone adhesives containing CD:Oct mixtures.
Acknowledgments
We acknowledge the funding from the Innovation Fund Denmark for the financing of the industrial PhD position for Valeria Chiuala. The microbial department at Coloplast is acknowledged for the help with the antimicrobial data.
Disclosure statement
No potential conflict of interest was reported by the authors.
Additional information
Funding
References
- Han G, Ceilley R. Chronic wound healing: a review of current management and treatments. Adv Ther. 2017;34(3):599–610. doi: 10.1007/s12325-017-0478-y.
- Cutting KF. Impact of adhesive surgical tape and wound dressings on the skin, with reference to skin stripping. J Wound Care. 2008;17(4):160–162. doi: 10.12968/jowc.2008.17.4.28836
- Hansen D, Zajforoushan Moghaddam S, Eiler J, et al. Performance of polymeric skin adhesives during perspiration. ACS Appl Polym Mater. 2020;2(4):1535–1542. doi: 10.1021/acsapm.9b01214
- Bjarnsholt T, Kirketerp-Møller K, Jensen PØ, et al. Why chronic wounds will not heal: a novel hypothesis wound repair and regeneration. Wound Repair Regener. 2008;16(1):2–10. doi: 10.1111/j.1524-475X.2007.00283.x
- Serra R, Grande R, Butrico L, et al. Chronic wound infections: the role of Pseudomonas aeruginosa and staphylococcus aureus. Expert Rev Anti Infect Ther. 2015 May;13(5):605–613.
- Ge Y, Wang Q. Current research on fungi in chronic wounds. Front Mol Biosci. 2022 Jan;9:1057766. doi: 10.3389/FMOLB.2022.1057766
- Yazdanpanah L, Nasiri M, Adarvishi S. Literature review on the management of diabetic foot ulcer. World J Diabetes. 2015;6(1):37–53. Accessed May 25, 2023. [Online]. Available: https://www.ncbi.nlm.nih.gov/pmc/articles/PMC4317316/
- Wang Y, Gao B, He B, et al. Toward efficient wound management: bioinspired microfluidic and microneedle patch. Small. 2023;19:2206270. doi: 10.1002/smll.202206270
- Sivakumar S, Murali R., Arathanaikotti D., et al. Ferulic acid loaded microspheres reinforced in 3D hybrid scaffold for antimicrobial wound dressing. Int J Biol Macromol. 2021; 177:467–473. doi: 10.1016/j.ijbiomac.2021.02.124.
- Chen H, Peng Y, Wu S, et al. Electrospun 3D fibrous scaffolds for chronic wound repair. Materials. 2016;9(4):272. doi: 10.3390/ma9040272
- Jose J, Pai AR, Gopakumar DA, et al. Novel 3D porous aerogels engineered at nano scale from cellulose nano fibers and curcumin: an effective treatment for chronic wounds. Carbohydr Polym. 2022;287:119338. DOI:10.1016/j.carbpol.2022.119338
- Tsegay F, Elsherif M, Butt H. Smart 3D printed hydrogel skin wound bandages: a review. Polymers. 14(5): MDPI:1012. Mar. 1, 2022. 10.3390/polym14051012
- Mirani B, Pagan E, Currie B, et al. An advanced multifunctional hydrogel-based dressing for wound monitoring and drug delivery. Adv Healthc Mater. 2017;6(19):1700718.
- Long J, Etxabide Etxeberria A, Nand AV, et al. A 3D printed chitosan-pectin hydrogel wound dressing for lidocaine hydrochloride delivery. Mater Sci Eng C. 2019;104:109873. doi: 10.1016/j.msec.2019.109873
- Muwaffak Z, Goyanes A, Clark V, et al. Patient-specific 3D scanned and 3D printed antimicrobial polycaprolactone wound dressings. 2017. doi: 10.1016/j.ijpharm.2017.04.077.
- Seol Y-J, Lee H, Copus JS, et al. 3D bioprinted biomask for facial skin reconstruction. Bioprinting. 2018;10:e00028. doi:10.1016/j.bprint.2018.e00028
- Shi G, Wang Y, Derakhshanfar S, et al. Biomimicry of oil infused layer on 3D printed poly(dimethylsiloxane): non-fouling, antibacterial and promoting infected wound healing. Mater Sci Eng C. 2019;100:915–927. doi:10.1016/j.msec.2019.03.058
- Chiaula V, Mazurek P, Eiler J, et al. Glycerol-silicone adhesives with excellent fluid handling and mechanical properties for advanced wound care applications. Int J Adhes Adhes. 2020;102:102667. DOI:10.1016/j.ijadhadh.2020.102667
- Mazurek P, Brook MA, Skov AL. Glycerol-silicone elastomers as active matrices with controllable release profiles. Langmuir. 2018;34(38):11559–11566. doi: 10.1021/acs.langmuir.8b02039
- Moon J, Huh Y, Park J, et al. Adhesion behavior of catechol-incorporated silicone elastomer on metal surface. ACS Appl Polym Mater. 2020;2(6):2444–2451. doi: 10.1021/acsapm.0c00387
- Bejenariu AG, Yu L, Skov AL. Low moduli elastomers with low viscous dissipation. Soft Matter. 2012;8(14):3917–3923. doi: 10.1039/c2sm25134e
- González L, Baoguang M, Li L, et al. Encapsulated PDMS microspheres with reactive handles. Macromol Mater Eng. 2014;299(6):729–738. doi: 10.1002/mame.201300319
- Mazurek P, Vudayagiri S, Skov AL. How to tailor flexible silicone elastomers with mechanical integrity: A tutorial review. Chem Soc Rev. 2019;48(6):1448–1464. doi: 10.1039/c8cs00963e.
- Winter HH, Chambon F. Analysis of linear viscoelasticity of a crosslinking polymer at the gel point. J Rheol (N Y N Y). 1986;30(2):367–382. doi: 10.1122/1.549853.
- Villar MA, Bibbó MA, Vallés EM. Influence of pendant chains on mechanical properties of model poly(dimethylsiloxane) networks. 1. analysis of the molecular structure of the network. Macromolecules. 1996;29(11):4072–4080. doi: 10.1021/ma9506593.
- Villar MA, Vallés EM. Influence of pendant chains on mechanical properties of model poly(dimethylsiloxane) networks. 2. viscoelastic properties. Macromolecules. 1996;29(11):4081–4089. doi: 10.1021/ma9506602.
- Chen S, Zhang J. Catalytic activity and inhibition of platinum complexes for room temperature vulcanized silicone rubber. Recent Pat Mater Sci. 2010;2(2):158–166. doi: 10.2174/1874465610902020158
- Hallerstig LM, Granath P, Lindgren L, et al. Determination of silver in soft silicone wound dressings using dodecylbenzene sulfonic acid digestion and inductively coupled plasma optical emission spectroscopy. Anal Methods. 2017;9(1):149–153. doi: 10.1039/c6ay02230h
- Vimbela GV, Ngo SM, Fraze C, et al. Antibacterial properties and toxicity from metallic nanomaterials. Int J Nanomedicine. 2017;12:3941–3965. doi: 10.2147/IJN.S134526
- Nam G, Rangasamy S, Purushothaman B, et al. The application of bactericidal silver nanoparticles in wound treatment. Nanomater Nanotechnol. 2015;5(July):23. doi: 10.5772/60918.
- Brandt O, Mildner M, Egger AE, et al. Nanoscalic silver possesses broad-spectrum antimicrobial activities and exhibits fewer toxicological side effects than silver sulfadiazine. Nanomedicine. 2012;8(4):478–488. doi: 10.1016/j.nano.2011.07.005
- Williamson DA, Carter GP, Howden BP. Current and emerging topical antibacterials and antiseptics: agents, action, and resistance patterns. Clin Microbiol Rev. 2017;30(3):827–860. doi: 10.1128/CMR.00112-16.
- Vigano L, Nosotti MG, Orlova N, et al. Use of chlorhexidine, side effects and antibiotic resistance. Biointerface Res Appl Chem. 2018;8(3):3265–3266.
- Mazurek P, Frederiksen NS, Silau H, et al. Glycerol–silicone membranes for sustained and controlled topical delivery of antimicrobial and pain-relief drugs. Adv Mater Interfaces. 2021 Mar;8(5):2001873.
- Thaha KA, Varma RL, Nair MG, et al. Interaction between octenidine-based solution and sodium hypochlorite: a mass spectroscopy, proton nuclear magnetic resonance, and scanning electron microscopy–based observational study. J Endod. 2017;43(1):135–140. doi: 10.1016/j.joen.2016.09.015
- Eldeniz AU, Guneser MB, Akbulut MB. Comparative antifungal efficacy of light-activated disinfection and octenidine hydrochloride with contemporary endodontic irrigants. Lasers Med Sci. 2015;30(2):669–675. doi: 10.1007/s10103-013-1387-1.
- Stewart CA, Finer Y, Hatton BD. Drug self-assembly for synthesis of highly-loaded antimicrobial drug-silica particles. Sci Rep. 2018;8(1):1–12. doi: 10.1038/s41598-018-19166-8.
- Alkhatib Y, Dewaldt M, Moritz S, et al. Controlled extended octenidine release from a bacterial nanocellulose/Poloxamer hybrid system. Eur J Pharm Biopharm. 2017;112:164–176. doi: 10.1016/j.ejpb.2016.11.025
- Promzeleva M, Volkova T, Proshin A, et al. Improved biopharmaceutical properties of oral formulations of 1,2,4-thiadiazole derivative with cyclodextrins: In Vitro and in vivo evaluation. ACS Biomater Sci Eng. 2018;4(2):491–501. doi: 10.1021/acsbiomaterials.7b00887
- Loftsson T, Másson M, Brewster ME. Self-association of cyclodextrins and cyclodextrin complexes. J Pharm Sci. 2004;93(5):1091–1099. doi: 10.1002/jps.20047.
- Popielec A, Loftsson T. Effects of cyclodextrins on the chemical stability of drugs. Int J Pharm. 2017;531(2):532–542. doi: 10.1016/j.ijpharm.2017.06.009.
- Marques J, Braga TM, Almeida Paz FA, et al. Cyclodextrins improve the antimicrobial activity of the chloride salt of ruthenium(II) chloro-phenanthroline-trithiacyclononane. Biometals. 2009;22(3):541–556. doi: 10.1007/s10534-009-9211-x
- Valente AJM, Carvalho RA, Söderman O. Do cyclodextrins aggregate in water? insights from NMR experiments. Langmuir. 2015;31(23):6314–6320. doi: 10.1021/acs.langmuir.5b01493
- Chougule V, Rajmane M, Chougule N, et al. Development of reverse-phase high-performance liquid chromatographic and UV-Spectrophotometric method with validation for octenidine dihydrochloride. Am J PharmTech Res. 2022;12(3): [Online]. Available. http://www.ajptr.com/www.ajptr.com
- Style RW, Boltyanskiy R, Allen B, et al. Stiffening solids with liquid inclusions. Nat Phys. 2015;11(1):82–87. doi: 10.1038/nphys3181
- Frankaer SMG, Jensen MK, Bejenariu AG, et al. Investigation of the properties of fully reacted unstoichiometric polydimethylsiloxane networks and their extracted network fractions. Rheol Acta. 2012;51(6):559–567. doi: 10.1007/s00397-012-0624-z
- Schneider HJ, Hacket F, Rüdiger V, et al. NMR studies of cyclodextrins and cyclodextrin complexes. Chem Rev. 1998;98(5):1755–1785. doi: 10.1021/cr970019t
- Kamal MS. A review of gemini surfactants: potential application in enhanced oil recovery. J Surfactants Deterg. 2016;19(2):223–236. doi: 10.1007/s11743-015-1776-5.
- Guerrero-Martínez A, González-Gaitano G, Viñas MH, et al. Inclusion complexes between β-cyclodextrin and a gemini surfactant in aqueous solution: an NMR study. J Phys Chem B. 2006;110(28):13819–13828. doi: 10.1021/jp0615813
- Hill ZD, MacCarthy P. Novel approach to job’s method: an undergraduate experiment. J Chem Educ. 1986;63(2):162–167. doi: 10.1021/ed063p162.
- Fernandes CM, Carvalho RA, Pereira da Costa S, et al. Multimodal molecular encapsulation of nicardipine hydrochloride by β-cyclodextrin, hydroxypropyl-β-cyclodextrin and triacetyl-β-cyclodextrin in solution. structural studies by 1H NMR and ROESY experiments. Eur J Pharmaceut Sci. 2003;18(5):285–296. doi: 10.1016/S0928-0987(03)00025-3.
- Upadhyay SK, Kumar G. NMR and molecular modelling studies on the interaction of fluconazole with β-cyclodextrin. Chem Cent J. 2009;3(1):1–9. doi: 10.1186/1752-153X-3-9.
- Zhu B, Jia L, Guo X, et al. Controllable assembly of a novel cationic gemini surfactant containing a naphthalene and amide spacer with β-cyclodextrin. Soft Matter. 2019;15(15):3198–3207. doi: 10.1039/c9sm00172g
- Eshelby JD. The determination of the elastic field of an ellipsoidal inclusion, and related problems. Proc R Soc Lond A Math Phys Sci. 1957;241(1226):376–396. doi: 10.1007/1-4020-4499-2_18.
- Style RW, Wettlaufer JS, Dufresne ER. Surface tension and the mechanics of liquid inclusions in compliant solids. Soft Matter. 2015;11(4):672–679. doi: 10.1039/c4sm02413c.
- Duan HL, Wang J, Huang ZP, et al. Eshelby formalism for nano-inhomogeneities. Proc R Soc A. 2005;461(2062):3335–3353. doi: 10.1098/rspa.2005.1520
- Wang J, Duan HL, Huang ZP, et al. A scaling law for properties of nano-structured materials. Proc R Soc A. 2006;462(2069):1355–1363. doi: 10.1098/rspa.2005.1637
- Cheng J, Hu Y, Luo Z, et al. Preparation and properties of octenyl succinate β-cyclodextrin and its application as an emulsion stabilizer. Food Chem. 2017;218:116–121. doi: 10.1016/j.foodchem.2016.09.019
- Makhlof A, Miyazaki Y, Tozuka Y, et al. Cyclodextrins as stabilizers for the preparation of drug nanocrystals by the emulsion solvent diffusion method. Int J Pharm. 2008;357(1–2):280–285. doi: 10.1016/j.ijpharm.2008.01.025.
- Fried JR. Polymer science and technology. Third edit ed. Westford (MA): Prentice Hall; 2014.
- Mazurek P, Hvilsted S, Skov AL. Green silicone elastomer obtained from a counterintuitively stable mixture of glycerol and PDMS. Polymer (Guildf). 2016;87:1–7. doi: 10.1016/j.polymer.2016.01.070
- Kirketerp-Møller K, Jensen PØ, Fazli M, et al. Distribution, organization, and ecology of bacteria in chronic wounds. J Clin Microbiol. 2008;46(8):2717–2722. doi: 10.1128/JCM.00501-08
- Rhoads DD, Wolcott RD, Sun Y, et al. Comparison of culture and molecular identification of bacteria in chronic wounds. Int J Mol Sci. 2012;13(3):2535–2550. doi: 10.3390/ijms13032535
- Omar A, Wright J, Schultz G, et al. Microbial biofilms and chronic wounds. Microorganisms. 2017;5(1):9. doi: 10.3390/microorganisms5010009.
- Song F, Brasch ME, Wang H, et al. How bacteria respond to material stiffness during attachment: a role of Escherichia coli flagellar motility. ACS Appl Mater Interfaces. 2017;9(27):22176–22184. doi: 10.1021/acsami.7b04757