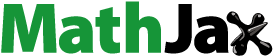
Abstract
Surface subsidence and fissures, especially surface fissures, are typical geological disasters related to groundwater activities in the Su-Xi-Chang area, China. In this study, we first analysed the geologic background and hydraulic features of earth formation in the area and constructed a physical model and its related experimental system in the laboratory. Based on the model and the system, we further experimentally studied and theoretically analysed the mechanism of ground fissure formation. The results showed that development of the earth fissures obviously undergoes the following stages. First, under self-gravity, the soil (artificial sand) layers in the model box with larger void ratios are gradually compacted, leading to uneven surface subsidence. Second, although the excess pore-water pressure could cause the surface to rise locally and macroscopically, water impounding on the aquifer lowers the friction among sand grains to certain degree depending on the strength of seepage in the horizontal direction, resulting in surface deformation and subsidence. Third, strong water pumping instantaneously releases excess pore-water pressure, resulting in soil consolidation in the vertical direction. Therefore, repeated groundwater impounding and pumping together with unique geological conditions lead to the formation of earth fissures in the Su-Xi-Chang area.
1. Introduction
Earth fissures occurring as surface or near-surface rock and soil fractures are harmful geological phenomena due to active tectonics and human activities in plains or basins, such as those in the Southwest American basin, the Indo-Gangetic plain in India, the valley in west Asia, Somalia peninsula in Africa, Iceland area, etc. (Rogers Citation1967; Hauksson Citation1983; Baruni Citation1994; Bankher and Al-Harthi Citation1999; Singh et al. Citation2011). There are more than 3000 earth fissures distributed in over 700 places in the north China plain. Among them, 78% are non-structural (Xu et al. Citation2015). Researches have shown that both internal and external dynamic geological processes and human engineering activities play crucial roles in their formation and evolution. The effects of water-related human activities, such as ground water extraction, reservoir and canal storage, seepage from irrigation, surface subsidence control, recharge, and the like, are particularly obvious on the formation of earth fissures in the shallow ground. Groundwater is a quasi-closed system influenced by topography, geological structure, climate (rainfall), etc. Its changes will significantly affect the formation and evolution of soil fractures, particularly in the near-surface area (Xu et al. Citation2013).
The surface fractures caused by over-pumping have been found worldwide. The first fissure discovered in the southern Arizona extends for about 3 km along the Picacho fault in a NE–SW direction (Leonard Citation1929; Bouwer Citation1977; Conway Citation2016). In Wadi Najran basin area of Kingdom of Saudi Arabia, increase of groundwater extraction for agricultural irrigation has caused earth fissures at various degrees in the tensile stress zone (Youssef et al. Citation2014). In the Aguascalientes Valley, México, earth fissures are formed due to vertical displacement (reaching 1.1 m) at the ground surface due to over-exploitation of deep groundwater (Pacheco-Martinez et al. Citation2013). In the Kanpur area of North India where a set of alluvial deposits in huge thickness (more than 500 m) forms, the average subsidence due to decline of piezometric head is estimated using a quasi-three-dimensional model; when a certain extraction flow (critical value) is reached, the damage to the surface and even to the surface buildings will develop (Mishra et al. Citation1993). These studies indicate that the formation of land fissures is obviously related to groundwater pumping at the aspects of time, space, and intensity.
Some researchers have shown that the participation of external water (heavy rainfall water, irrigation water, etc.) has direct and indirect effects on stratum media, which further change the balance of systems inside the stratum and finally cause surface deformation—surface subsidence and earth fissures (Mishra et al. Citation1993). Some researchers have studied the causes of earth fissures from the point of their mechanical behaviours. Some of them hold that these fissures are caused by differentially deformed confined layers in the horizontal direction, while others believe that these fissures are caused by the intensity-gradient effects of groundwater extraction (Burbey Citation2002; Hernandez-Marin and Burbey Citation2009; Budhu Citation2011). In fact, the impacts of groundwater activities on the strata have been observed in both horizontal and vertical directions. Even large block bendings have been found (Sheng et al. Citation2003; Budhu Citation2008; Xu et al. Citation2015). Some researchers believe that specific soil masses are characteristics of collapsibility and structural discontinuity. Due to water erosion, soil masses will be washed away, leading to the formation of earth fissures as those in China’s loess areas and Saudi regions (Amin and Bankher Citation1997; Li et al. Citation2000). Clearly, this theory (soil collapsibility) emphasizes the infiltration of surface water and indicates that the fissures have been developed in the soil before the action of surface water and the surface water only further extends or fills the existing earth fissures.
However, although surface subsidence has been decelerated to some degree due to natural and artificial groundwater recharges, its trend is not reversed due to excessive groundwater and surface water exploitation in some areas, such as Shanghai area and Su-Xi-Chang area (Chen et al. Citation2003; Zhang et al. Citation2015; Yin et al. Citation2016). In particular, it should be noted that despite the gradual implementation of measures prohibiting groundwater use in the area after 2000, there is still surface subsidence at the rate of 5–10 mm/year (Shi et al. Citation2014). Accurate monitoring data in Suzhou and Guangming of the Su-Xi-Chang area show that during the aquifer impounding period, land subsidence still occurs at a certain rate without obvious recovery (Gu et al. Citation2018). Therefore, it is particularly important to study the roles of groundwater level fluctuations in the formation of ground fissures. In this study, we focus on the problem in Su-Xi-Chang area based on geological survey, model test and theoretical analysis.
2. Geological background
The Su-Xi-Chang area locates in the hinterland of Yangtze River Delta, the southeast corner of the North China Plain, bordering the Yangtze River in the northeast direction and neighbouring the Taihu Lake in the southwest (). The area lies between E 119°40′ and E 121°18′ and N 30°46′ and N 32°05′ with a total area of about 12,000 km2. Since the 1980s, especially 1990s, excessive exploitation and utilization of the land surface due to rapid population growth, industrialization and urbanization have greatly damaged its original geological environment, resulting in surface subsidence, soil impoverishment, etc. Especially, earth fissures, as the indicator of surface environment deterioration, have been found in up to 21 places.
Figure 1. Geologic background and earth fissures’ distribution in the Su-Xi-Chang area. 1—land subsidence displacement is <600 mm. 2—land subsidence displacement is between 600 and 1200 mm. 3—land subsidence displacement is >1200 mm. 4—bedrock outcrop, 5—earth fissure, 6—fault, 7—underlying bedrock, 8—anticline, 9—syncline. F1—Su-Xi-Chang fault, F2—Huzhou-Suzhou fault, F3—Kunshan-Jiading fault, F4—Wujiang-Anting fault, F5—Taicang-Zhitang fault, F6—Shaxi-Ouqu fault, F7—Wuxi-Chongming fault, F8—Meili-Dongbang fault, F9—Yuewang-Heshi fault. The line ‘P–P?’ is the stratigraphic section line (shown in ). The points ‘BK1’ and ‘BK2’ are the locations of drilling bores. The point ‘TC’ is the location of the trench.
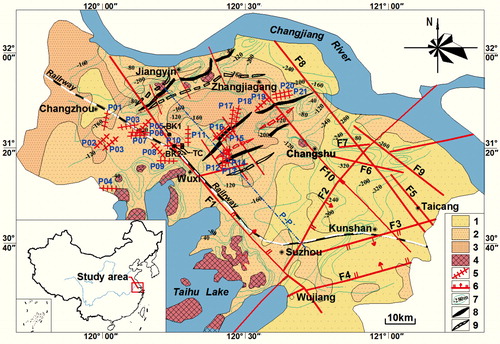
The study area is located in Yangtze platform. Its base belongs to the metamorphic strata in the Middle or Late Proterozoic. Influenced by the interaction of both Indo-Chinese movement and Yanshan Mountain movement, the tectonics of its base is very complicated and has great impact on later geological environment, controlling the distribution of ancient river beds and the formation of ancient hydrodynamic conditions and influencing the structure of the Quaternary sedimentary strata. Such geological structure creates a unique shallow and undulating base structure in the buried hill. As shown in , these bedrocks are densely distributed in the area between the south bank of the Yangtze River and the Taihu Lake. Some exploratory researches have shown that some bedrocks are exposed to the surface (Wu et al. Citation2003), and most bedrocks have minimum buried depth of 76 m and maximum buried depth of 163 m (). The Quaternary alluvial deposits mainly the clays and sands could be observed at the top of the bedrocks. The interlaced sedimentary stratum structure is ideal for studies on the occurrence and movement of groundwater, as well as the development of earth fissures.
Figure 2. Stratigraphic section map of Su-Xi-Chang area (modified from Wu et al. Citation2003). Its location is shown in (P–P?). 1—pebbly sand, 2—clay, 3—silt, 4—silty clay, 5—sandy clay, 6—bedrock.
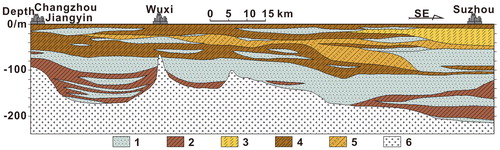
3. Basic characteristics of ground fissures
3.1. Distribution
Based on the surface distribution, earth fissures in the Su-Xi-Chang plain are mainly concentrated in the Northwest block (Dangkou-Baimao fault depression). Only a small number of earth fissures are distributed in other blocks. The field survey shows that these earth fissures are 100–2000 m long. Among them, 24% are over 500 m long and distributed in 6 places, and 76% are less than 500 m and distributed in 19 places. Most exposed ground fissures are close to linear cracks on the surface with some displacements (), and the resultant surface scarps wreak various damages on roads, farmland and houses () with their influential scope from 30 to 400 m (Liu et al. Citation2004).
Figure 3. Disasters and manifestations of earth fissures in Su-Xi-Chang area (modified from Liu et al. Citation2004). (a) Yinguoan fissure (, P10), (b) Honglian School fissure (, P05), (c) Changjng fissure (, P17), d—Tangqiao fissure (, P20).
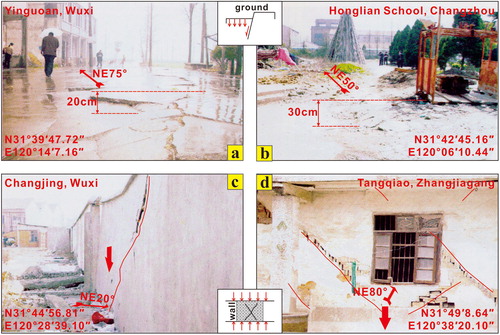
Apparently, these earth fissures are in zonal distribution on the surface and can be divided into two groups by the Su-Xi-Chang fault (, F1). One group distributes along the NE direction and locates in the northern research area, which is the core area of surface subsidence with subsidence depth of more than 3 m and basically distributes along the uplift of the buried bedrocks () (Liu Citation2011). Taking Changjing of Wuxi as an example, their shallow seismic lines indicate a good relation between the earth fissures and the uplift of bedrocks, which have buried depth between 105 and 152 m, in the NE direction (Wang et al. Citation2010). Above the bedrocks, there are various alluvial layers of hard plastic silty clays, clays and sands. The other group locates in the northwestern Su-Xi-Chang area in the NW direction. These earth fissures are developed with smaller surface subsidence with subsidence displacement less than 3 m. They basically extend along the Wuxi-Changzhou direction and show no obvious bedrock uplift in the related deep stratum (). Their flat base provides an excellent channel for groundwater flowing. Overall, compared with earth fissures in other regions, earth fissures in the Su-Xi-Chang area are formed complicatedly and developed along the zone with greater hydraulic slope.
3.2. Sectional structure
To explain the profile shape of these earth fissures, we selected a typical earth fissure developed in the Yingguoan area, a Wuxi suburb, as the study object and applied a series of methods such as trenching, drilling and geophysically prospecting to reveal its profile characteristics. This earth fissure is about 2000 m long in the NE direction and the longer one of two nearly parallel cracks in the region (Yu et al. Citation2006). The trenching results show that there is a complete up–down dislocation on both sides of the earth fissure about 3 m below the surface and there are two cracks extending to the surface in a “V” shape, causing a scarp with the level difference of 26 cm () (Zong Citation2005). The results of surface wave measurement show that the earth fissure almost cuts through the whole Quaternary strata with the buried depth of 36 m (Liu et al. Citation2004). The drilling data show that the formation of the earth fissure has the characteristics of duality (double stratum group) and anisotropy (Liu et al. Citation2004). The stratum structure in the region mainly contains both compacted silt group and loose sand group. In addition, the sand layers are uneven and fluctuant in different places. Earth fissures are often developed in buried hill with larger changes (such as northern earth fissure groups) and thicker sand layers (such as northwestern earth fissure groups).
Figure 4. Drilling and trenching profile of earth fissures in Su-Xi-Chang area (modified from Zong Citation2005). (a) Drilling profile from bore ‘BK1’ whose location is shown in , ‘BK1’. (b) Drilling profile from bore ‘BK2’ whose location is shown in , ‘BK2’. (c) Trenching profile whose location is shown in , ‘TC’. 1—silty clay, 2—clay, 3—muddy clay, 4—coarse sand, 5—fine sand, 6—filling soil in fissure.
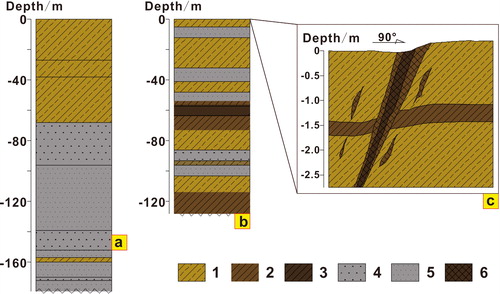
3.3. Hydrological characteristics
In the study area, the formation of ground fissures is often accompanied with surface subsidence and has a good coincidence with its temporal and spatial distribution (Hu et al. Citation2009). For Suzhou region in the Su-Xi-Chang area, the regional groundwater extraction can be divided into three phases. In the 1960s, Suzhou’s industry and agriculture development was relatively slow and groundwater was mainly used for human life. At that time, the level of groundwater remained at a high level (about –40 m). Between 1970s and 1990s, rapid development of regional economy led to the excessive pumping of groundwater, leading to a significant drop of groundwater level to the lowest point (–58 m). Since 1990s, local government has realized the negative effect of surface subsidence on geological environment and taken a series of macroscopic measures to restrict the excessive exploitation of groundwater, making groundwater level gradually rise. It is worth of note that the surface subsidence still continues regardless of groundwater level rising or lowering (Shi et al. Citation2007; Zhang et al. Citation2010). Thus, a sudden rise in groundwater level also causes surface subsidence or earth fissures.
4. Physical model experiment
4.1. Experimental model
The above analyses show that the formation of earth fissures is closely related to groundwater activities. In order to observe the various ground displacement processes, intra-soil stress as well as earth fissure formation process under repeated water pumping and impounding, we established an indoor physical model based on similar ground fissures formation process. The model system mainly consists of a model box and a water storage tank, as shown in . The model box is a high strength steel cylinder with diameter of 100 cm and height of 78 cm and used as the core part of the system to ensure the stability of the experiment environment and compactly filled in turn with various types of layers from the bottom to the top of the box, namely concrete layer, clay layer, coarse sand layer and silt layer. The bottom is an undulating concrete layer used to simulate the real bedrock shape. Both the clay and concrete layers have a good water-sealing effect and form the corresponding confined aquifers allowing water permeating at some extent into the coarse sand layers. The water inlet and outlet have been pre-installed on the sides of the model box () to control water impounding and pumping, respectively. The top silt layer is about 20 cm thick because silt is sensitive to ground displacement and is more in line with the actual surface soil.
Figure 5. Indoor physical fissure-caused experiment model. (a) Structure diagram, (b) front view, (c) top view, (d) strain gage. 1—silt, 2—fine sand, 3—clay, 4—bedrock (concrete). ①—model box (steel plate), ②—water outlet (with filter), ③—wire net, ④—well, ⑤—waterproof material, ⑥—water storage tank, ⑦—dial gauge, ⑧—water valve. The impounding effect is simulated by opening the valve of water storage tank (⑥) and slowly injecting a certain amount of natural water to the aquifer. The pumping effect is simulated by opening the valve of the model box (①).
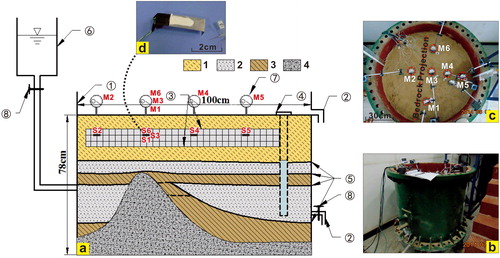
To make experimental simulation closer to reality and maximize the similarity between the model and prototype at experimental conditions, the geometric scale of the model is designed to be 1:100 by referencing to the ground fissure test model in Xi’an, China (Zhao and Wang Citation1995). In other words, its geometric similarity coefficient () is 100. As the model uses soil extracted from the field, the mass ratio of the test material to the actual mass (Cγ) is 1. According to the similarity principle, the relationship between its measured value (
) and the actual value (
) is
(1)
(1)
Six dial gauges (M1–M6, ) with precision of 0.001 mm are installed on the surface to monitor subtle surface displacement, as shown in . Moreover, six sets of strain gauges (S1–S6, ) are buried inside the silt layers at positions corresponding to the dial gauges in the vertical direction to monitor and record the changing characteristics of the deep soil.
4.2. Experimental process and results
Before filling, the materials were under load and stood for about 3 months to reduce the vertical displacement due to their weight to a negligible range (<0.001 mm). According to the experimental design, four groups of ‘water impounding and pumping’ processes are carried out and marked as T1–T2, T3–T4, T5–T6 and T7–T8 in time, among which, T1, T3, T5 and T7 refer to water-impounding and T2, T4, T6 and T8 refer to water-pumping. The final stable values are considered as the recorded results in each experiment. The experimental strength, or the volume ratio of water to the coarse sand layers, is controlled by water inflow and outflow. According to the proportion of water (inflow) to the water capacity of aquifer, which is about 5.0 × 104 mL for the coarse sand layer, the strength could be divided into three levels, i.e. the low level (20%, that is, the volume ratio of water in the coarse sand layer is about 1.0 × 104 mL), middle level (50%, i.e. the volume ratio of water is about 2.5 × 104 mL) and high level (80%, i.e. the volume ratio of water is about 4.0 × 104 mL). The strength is at low level in processes of T1–T2 and T3–T4, middle level in process of T5–T6 and at high level in process of T7–T8. Accordingly, the experiment process can be divided into three stages.
4.2.1. Stage I (T1–T2, T3–T4)
This stage aims to observe the changes of the internal strata and ground surface due to low-strength water impounding and pumping. The experiment results based on changes in surface displacement and strain in the soil show that the soil only has minor subsidence and deformation without obvious differences among all monitoring points. The displacement curves at T1–T2 and T3–T4 stages () show that the relative displacement of ground surface is 0.05–0.2 mm. In other words, the corresponding actual displacement is 0.5–2 cm. The strain curves at T1–T2 and T3–T4 stages () show that the relative strain change inside the soil is 0%–0.005%. These results show that the sand layer is in a disorderly state in natural geological environment and has an unstable structure with numerous large pores. The surface subsidence is just the manifestation of such self-compaction effect as indicated as the overall upward strain curves at T2–T3 and T4–T5 stages in . This rearrangement also makes the overall structure more stable. Under the pumping effect, pore water pressure declines and effective stress increases, which reinitiate the self-compaction, thus leading to surface subsidence and deformation. It is important to note that subsidence changes usually show hysteresis, which could be avoided by long-time monitoring. However, the undeniable fact is that this subsidence has a significant correlation with the pumping-water storage effect, that is, deformation occurs as water volume increases or decreases.
4.2.2. Stage II (T5–T6)
After repeated deformation at stage I, a set of moderate-strength water impounding and pumping process is simulated at stage II (T5–T6). The displacement and strain curves at stage II show two new characteristics. The first obvious characteristic is ‘sudden change’. The subsidence deformation is non-uniformly distributed at each point and sudden changes could be observed in each curve at the positions of bedrock uplift where earth fissures are likely to occur. At the M3 position in T6 curve in , the difference in subsidence is up to 0.2–0.3 mm, corresponding to actual displacement of 2–3 cm. The second characteristic is the ‘differentiation’. Comparing the water impounding process (T5) with the water pumping process (T6), it was found that there are differences in the amount, rate and pattern of subsidence change. The subsidence due to water storage is smaller than that due to water pumping, and the former has no obvious regularity. The greater subsidence can even be observed in the corresponding position of bedrock uplift in the strain curve, as shown by the downward concave shape at S3 in the T5 curve (). Thus, the ‘water-impounding-induced subsidence’ and ‘water-pumping-induced subsidence’ are not the same in mechanism.
4.2.3. Stage III (T7–T8)
In the fourth set of water impounding and pumping processes (T7–T8), the experiment model was observed for a long period of time. The results show that sudden changes occurred in both water impounding and pumping processes. The inflection points were observed at corresponding bedrock positions and the earth fissures were also observed (), indicating that the surface has experienced great deformation with the maximum relative subsidence reaching 1.3 mm, which corresponds to the actual displacement of 13 cm (, M3–T8). Clearly, the hydraulic properties of the soil under the action of water are strengthened with the increase of test water in the experiment. However, compared with the water pumping process, the differential subsidence caused by water storage activity is much smaller and soil strain is relatively constant (, S3–T7), indicating the difference in deformation caused by water impounding is subtle among different locations. Therefore, we can conclude that the impact of water impounding is mainly in the horizontal direction, while that of water pumping is obvious in the vertical direction.
Figure 8. Earth fissures on the surface under the effect of high-intensity water-related activities.
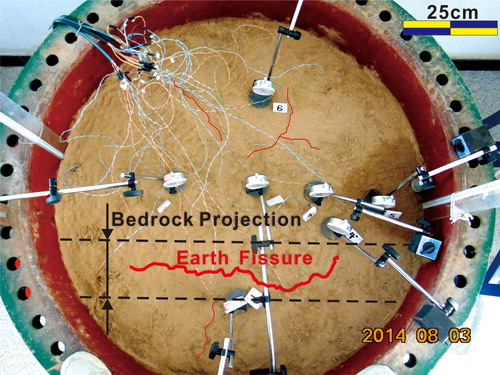
The combined water impounding and pumping experiments show that the deformation of soil mass can be discussed from two aspects. In the water impounding process, a slow and uniform deformation occurs inside the soil, while in the water pumping process, a great subsidence deformation occurs. The combined of water impounding and pumping effect causes great surface subsidence, resulting in the formation of earth fissures.
5. Theoretical analyses
In essence, the formation of macroscopic earth fissures reflects the microcosmic deformation of soil mass. In the deformation process, the effects of some factors on fissure formation are strengthened while those of others are weakened. They also exert different controls on the stress–strain distribution and evolution, such as the water impounding and pumping effects in model experiments. To reveal the effects of various factors (such as self-gravity, water impounding, water pumping) on the formation of earth fissures during the physical experiment, based on micro- and macro-deformation, we put forward the ‘three-stage theory’ based on the microstructure and macroscopic deformation, and believe that the soil layers are subjected to water action under different mechanisms at the three stages.
5.1. Mechanism of self-compaction
The self-gravity compaction is caused by the decrease in pore volume. In other words, under the effect of gravity stress, the sand becomes denser. At relatively loose state, sand mass is in a nature environment and even forms larger fracture channels due to thawing, hydraulic erosion, biological actions and other geological processes (Donnelly Citation2008). In this experiment, at the stage I (T1–T2, T3–T4) with low-strength water impounding and pumping, the surface subsidence changed slightly, smaller than 1.3 mm, as shown in . The sand mass can be simplified as a spherical structure as shown in . Assuming the sand mass with certain grade has a greater void ratio () without external disturbance, under the gravity action of the upper layer, some lower sand layers at a certain depth undergo a compressive deformation with a new void ratio (
). Based on the assumption that the compression of a stratum is entirely undertaken by the sand layer with thickness of
(i.e. the above strata with large porosity such as the clay layers are ignored), a coordinate system is established with its
-axis direction opposite to the bedrock uplift direction on the surface,
-axis direction parallel to the bedrock strike, and
-axis direction perpendicularly downward pointing to the uplift. For the point
in the sand layer, its projection on the x–y plane is
Assuming that the additional stress on the point is
and the resulting stratigraphic compression is
(, sand strata), one has
(2)
(2)
where
is the coefficient of volume compressibility obtained from the ‘stress–void ratio’ variation curve. Its average value is
(3)
(3)
As shown in , assuming that the initial height of groundwater level (the distance from the level to the bedrock or aquifer floor) is and
is the projection point of the top of the sand layer (horizontal surface) on the
-axis, and
is the projection point of the original groundwater level on the
-axis (
), the form of bedrock surface can be determined and expressed by the function
Thus,
and
can be expressed as follows:
(4)
(4)
(5)
(5)
Bring EquationEq. (4)(4)
(4) into EquationEq. (2)
(2)
(2) finds
as follows:
(6)
(6)
5.2. Mechanism of water impounding disturbance
Naturally, the sand layer is developed in specific sedimentary environment, such as fluvial facies, lacustrine facies, and neritic sedimentary facies. Under water erosion disturbance, the sand layers with different sedimentary sequence structures have different movement behaviours in degree and direction (Cao et al. Citation2013). This is because the void ratio inside the sand layer is determined by micromechanical structures. As shown in , sand mass in a nature state maintains an interactive balance among the gravity (), buoyancy force (
), friction (
), and seepage force (the effect of water on sand) (
). Thus, the resultant force in the vertical direction is
(7)
(7)
where
is the total friction between specific sand and the surrounding sand, and
is the angle between
and the vertical direction. Similarly, the seepage force (
) in the horizontal direction is found as follows:
(8)
(8)
It is important to note the seepage force of water on the sand frame is equal to the resistance of sand to seepage, but has the opposite direction. In nature, the process of water storage is an incremental process of groundwater overcoming the gravity and its vertical rise is actually the accumulation of water flowing in the horizontal plane. Namely, the seepage force () occurs along
-axis,
-axis and
-axis, and the sand mass has a corresponding displacement increment. Actually, the impact of water storage activity on the sand layer mainly depends on the direction and velocity of water seepage (Bjorlykke et al. Citation1993). When the groundwater flows in the vertical seepage direction, the smaller the flow velocity is, the smaller the friction (
) among sands. Under the action of buoyancy, the sand layer experiences a rebound effect. When the seepage moves mainly in the horizontal direction, the friction (
) also becomes smaller, but surface subsidence will occur under the gravity (
). Therefore, in the water pumping process at the stage II, both measured displacement and strain curves show sudden changes (, M3–T5; , S3–T5). Thus, the sand layer can be considered as an elastomer. In this case, let the vertical elastic modulus of the sand layer due to water impounding be
and the Poisson’s ratio be
both of which are different from the elastic parameters in one-dimensional drainage test (Al-Tabbaa and Wood Citation1991; Ghabezloo and Sulem Citation2010).
Assuming that the groundwater level rises from to
the stress increments along the
-axis,
-axis and
-axis are
and
respectively, and the vertical stress increment is
Then, according to Hooke’s law, the variation in water storage,
is found to be
(9)
(9)
5.3. Mechanism of water pumping consolidation
According to the effective stress principle, a decrease in groundwater level from to
() will lead to an increase in the effective stress on sand mass and to uniform compression under the effect of water pumping. The experiments found that both the surface displacement curve and the intra-soil strain curve due to water pumping show a consistently dropping trend (M6 and M8 in ; S6 and S8 in ). This deformation can be seen as a result of excess pore water pressure dissipation. The amount of deformation is the sand layer’s subsidence. Relevant investigations and experimental studies show that sand and soil are similar in essence (they are all weathering products). In the case of groundwater level fluctuation, the change of pore size can cause certain consolidation characteristics (Niu et al. Citation2013; Chang et al. Citation2017). Therefore, according to Terzaghi’s theory of one-dimensional consolidation, the excess pore water pressure (
) at any moment
and any depth
could be obtained from
(10)
(10)
(11)
(11)
(12)
(12)
where
is the initial hydrostatic pressure,
is an integer,
is dimensionless time factor, and
is the consolidation coefficient of the sand. To indicate the dissipation degree of hydrostatic pressure in the sand, the degree of consolidation (
) is introduced as follows:
(13)
(13)
For the land layer, if the groundwater level decreases to a certain range, the average consolidation degree U can be expressed as
(14)
(14)
Therefore, given the average strain, the amount of sand compression () due to water pumping is found as
(15)
(15)
The above analyses tell us the variational process of the sand layer under the effect of water impounding and pumping in the experiment. When the sand layer is in its natural environment, its subsidence deformation is mainly caused by the change of void ratio. As shown in EquationEq. (6)(6)
(6) , the void ratio changes from
to
Because the stratum in the study area is formed presumably in Middle Pleistocene to Late Pleistocene (Liu et al. Citation2004), the effect of self-gravity decreases gradually over time and even can be ignored. The water impounding activity in local area makes groundwater intrude into the space among the sand grains. Due to the presence of pressures from the upper layers, the seepage expands in the horizontal direction, driving sand grains to move horizontally and the sand layer to subside vertically—this subsidence is related to the magnitude of horizontal seepage force permeability, as shown in EquationEq. (8)
(8)
(8) . As the seepage intensity weakens, the pore water pressure increases accordingly, resulting in elastic deformation of sand grains and an increase in the thickness of the sand layer due to increase in buoyancy. Under the condition of slow rise of groundwater level, the aquifer (or even the surface) may have a ‘recovery effect’, which is related to the impounding mode and strength of groundwater, as shown in EquationEq. (9)
(9)
(9) . During water pumping, the excess hydrostatic pressure among sands disappears from deep to surface, leading to increased effective stress on the sand layer and further compression of the sand layer (EquationEq. 15
(15)
(15) ). However, in practice, it is very hard using water injection method to introduce excess hydrostatic pressure to the sand layer. Therefore, in the water impounding process, surface subsidence is observed rather than surface rise.
6. Discussion and conclusions
The results of this study can be summarized as follows. First, from the field survey, the geological structure of the Su-Xi-Chang area has both duality and inhomogeneity. Both of them determine the regional distribution feature of earth fissures in this area. The earth fissures are distributed with a greater hydraulic gradient change, not only along the bedrock uplift zone. Second, from the model experiments and theoretical analysis, the formation mechanism of earth fissures under the effect of water activities is very complex. Just like water pumping, water impounding can also cause great ground subsidence and even earth fissures. Third, according to ‘the stage theory’, the formation of fissures in soil mass (strata) undergoes three stages, namely self-gravity compaction, water-impounding disturbance, and water-pumping consolidation. Each action causes different deformations of the soil mass. Overall, water impounding activity induces surface subsidence or rebounding depending on the seepage intensity in the plane direction.
It is necessary to point out that our theory is a primary theory that needs to be verified through a long-term, controllable monitoring of earth fissures in the area. Compared with other related researches, this model experiment reveals an important phenomenon that the surface subsidence or earth fissures caused by groundwater pumping is a reversible process and combined water pumping and impounding measures may worsen this kind of geological disaster. The Su-Xi-Chang area has plenty of rainfall and is located between the Yangtze River and Taihu Lake. Thus, this area has strong groundwater extraction and natural groundwater recharge, forming a repeated water impounding and pumping mechanism. The water impounding effect increases the disturbance of soil particles and the water pumping effect causes the vertical compression of the strata. Finally, the differential surface subsidence worsens and leads to earth fissures above the unique shallow buried hill base. Therefore, the earth fissures with similar characteristics can be defined as ‘groundwater-related’ (impounding and pumping) type. It is necessary to point out that similar phenomena have been found in other areas of the North China Plain, such as the Hejian area in Hebei Province and the Tianjin area, especially in the plains with frequent water-related activities along coastlines and riversides (Xu et al. Citation2012; Deng et al. Citation2018). In the study of Su-Xi-Chang earth fissures, revealing these three modes, especially the mechanism of water-impounding disturbance, will not only help deepen our understanding of the nature of this type of earth fissures and the function of repeated groundwater pumping and impounding, but also provide a reference for the prevention and control of ground fissures-induced disasters. In the study of geological disaster prevention and control, it is necessary to take more factors such as the stratum conditions, groundwater recharge, artificial water storage modes and intensity into consideration.
Disclosure statement
No potential conflict of interest was reported by the authors.
Correction Statement
This article has been republished with minor changes. These changes do not impact the academic content of the article.
Additional information
Funding
References
- Al-Tabbaa A, Wood DM. 1991. Horizontal drainage during consolidation: insights gained from analyses of a simple problem. Géotechnique. 41(4):571–585.
- Amin A, Bankher K. 1997. Causes of land subsidence in the Kingdom of Saudi Arabia. Nat Hazards. 16(1):57–63.
- Bankher KA, Al-Harthi AA. 1999. Earth fissuring and land subsidence in Western Saudi Arabia. Nat Hazards. 20(1):21–42.
- Baruni S. 1994. Earth fissures caused by groundwater withdrawal in sarir south agricultural project area, Libya. Hydrogeol J. 2:45–52.
- Bjorlykke K, Cloetingh S, Sassi W, Horvath F, Puigdefabregas C. 1993. Fluid flow in sedimentary basins. Sediment Geol. 86:137–158.
- Bouwer H. 1977. Land subsidence and cracking due to ground-water depletion. Groundwater. 15(5):358–364.
- Budhu M. 2008. Mechanics of earth fissures using the Mohr-Coulomb failure criterion. Environ Eng Geosci. 14(4):281–295.
- Budhu M. 2011. Earth fissure formation from the mechanics of groundwater pumping. Int J Geomech. 11(1):1–11.
- Burbey TJ. 2002. The influence of faults in basin-fill deposits on land subsidence, Las Vegas Valley, Nevada, USA. Hydrogeol J. 10(5):525–538.
- Cao GL, Zheng CM, Scanlon BR, Liu J, Li WP. 2013. Use of flow modeling to assess sustainability of groundwater resources in the North China Plain. Water Resour Res. 49(1):159–175.
- Chang WJ, Chou SH, Huang AB. 2017. Physical simulation of aquifer compression due to groundwater fluctuation. Eng Geol. 231:157–164.
- Chen CX, Pei SP, Jiao JJ. 2003. Land subsidence caused by groundwater exploitation in Suzhou City, China. Hydrogeol J. 11(2):275–287.
- Conway BD. 2016. Land subsidence and earth fissures in south-central and southern Arizona, USA. Hydrogeol J. 4:649–655.
- Deng X, Li F, Zhao Y, Li S. 2018. Regulation of deep groundwater based on MODFLOW in the water intake area of the South-to-North Water Transfer Project in Tianjin, China. J Hydroinform. 20(4):989–1007.
- Donnelly LJ. 2008. Subsidence and associated ground movements on the Pennines, northern England. Q J Eng Geol Hydrogeol. 41(3):315–332.
- Ghabezloo S, Sulem J. 2010. Effect of the volume of the drainage system on the measurement of undrained thermo-poro-elastic parameters. Int J Rock Mech Min Sci. 47(1):60–68.
- Gu K, Shi B, Liu C, Jiang H, Li T, Wu J. 2018. Investigation of land subsidence with the combination of distributed fiber optics sensing techniques and microstructure analysis of soils. Eng Geol. 240:34–47.
- Hauksson E. 1983. Episodic rifting and volcanism at Krafla in North Iceland - growth of large ground fissures along the plate boundary. J Geophys Res. 88(B1):625–636.
- Hernandez-Marin M, Burbey TJ. 2009. The role of faulting on surface deformation patterns from pumping-induced groundwater flow (Las Vegas Valley, USA). Hydrogeol J. 17(8):1859–1875.
- Hu J, Shi B, Inyang HI, Chen J, Sui Z. 2009. Patterns of subsidence in the lower Yangtze Delta of China: the case of the Suzhou-Wuxi-Changzhou region. Environ Monit Assess. 153(1–4):61–72.
- Leonard RJ. 1929. An earth fissure in Southern Arizona. J Geol. 37(8):765–774.
- Li YL, Yang JC, Hu XM. 2000. Origin of ground fissures in the Shanxi Graben System, Northern China. Eng Geol. 55(4):267–275.
- Liu C, Yuan X, Zhu J. 2004. The earth fissures in Su-Xi-Chang. Wuhan, China: China University of Geosciences Press Co., Ltd.
- Liu F. 2011. Analysis on developing tendency of land subsidence around Suzhou-Wuxi-Changzhou area as well as its impacts on Beijing-Shanghai high speed railway. Railway Invest Surv. 1:33–37.
- Mishra SK, Singh RP, Chandra S. 1993. Prediction of subsidence in the Indo-Gangetic basin carried by groundwater withdrawal. Eng Geol. 33(3):227–239.
- Niu WJ, Wang ZY, Chen F, Li HR. 2013. Settlement analysis of a confined sand aquifer overlain by a clay layer due to single well pumping. Math Probl Eng. 2013:1–13.
- Pacheco-Martinez J, Hernandez-Marin M, Burbey TJ, Gonzalez-Cervantes N, Angel Ortiz-Lozano J, Eduardo Zermeno-De-Leon M, Solis-Pinto A. 2013. Land subsidence and ground failure associated to groundwater exploitation in the Aguascalientes Valley, Mexico. Eng Geol. 164:172–186.
- Rogers TH. 1967. Active extensional faulting north of Hollister near the Calaveras fault zone. Bull Seismol Soc Am. 4:813–816.
- Sheng ZP, Helm DC, Li J. 2003. Mechanisms of earth fissuring caused by groundwater withdrawal. Environ Eng Geosci. 9(4):351–362.
- Shi XQ, Xue YQ, Ye SJ, Wu JC, Zhang Y, Yu J. 2007. Characterization of land subsidence induced by groundwater withdrawals in Su-Xi-Chang area, China. Environ Geol. 52(1):27–40.
- Shi X, Feng Z, Yao B, Huang X, Wu J. 2014. Study on the deformation characteristics of soil layers after banning groundwater pumping in Su-Xi-Chang area. Quaternary Sci. 24:56–63.
- Singh RP, Mehdi W, Naeimi M, Sahoo A, Kumar R. 2011. Analysis of widespread fissures associated with groundwater depletion and extreme rainfall using multi sensor data. GRACE, remote sensing and ground-based methods in multi-scale hydrology. Vol. 343. Wallingford: International Association of Hydrological Science; p. 28–33.
- Wang GY, You GG, Shi B, Wu SL, Wu JQ. 2010. Large differential land subsidence and earth fissures in Jiangyin, China. Environ Earth Sci. 61(5):1085–1093.
- Wu Z, Yu Q, Zhang Y. 2003. Forming process of earth fissure hazard in the Suzhou-Wuxi-Changzhou area. Hydrogeol Eng Geol. 1:67–72.
- Xu J, Ma R, Peng J, Yang X, Ma X, An H. 2012. Study on fractal features of ground fissures in the area of Hejian. J Nat Disasters 21:177–183.
- Xu J, Meng L, An H, Wang L. 2015. The bending mechanism of Anping ground fissure in the Hebei Plain, North China. Environ Earth Sci. 74(9):6859–6870.
- Xu YS, Shen SL, Du YJ, Chai JC, Horpibulsuk S. 2013. Modelling the cutoff behavior of underground structure in multi-aquifer-aquitard groundwater system. Nat Hazards. 66(2):731–748.
- Yin J, Yu D, Wilby R. 2016. Modelling the impact of land subsidence on urban pluvial flooding: A case study of downtown Shanghai, China. Sci Total Environ. 544:744–753.
- Youssef AM, Sabtan AA, Maerz NH, Zabramawi YA. 2014. Earth fissures in Wadi Najran, Kingdom of Saudi Arabia. Nat Hazards. 71(3):2013–2027.
- Yu J, Chen G, Wang X, He H, Xu X, Wu J. 2006. Formation mechanism analysis on ground fissure based on 3D seismic exploration data in Yinguo'an area, Wuxi City. Hydrogeol Eng Geol. 33:117–120.
- Zhang Y, Wu J, Xue Y, Wang Z, Yao Y, Yan X, Wang H. 2015. Land subsidence and uplift due to long-term groundwater extraction and artificial recharge in Shanghai, China. Hydrogeol J. 23(8):1851–1866.
- Zhang Y, Xue Y-Q, Wu J-C, Shi X-Q, Yu J. 2010. Excessive groundwater withdrawal and resultant land subsidence in the Su-Xi-Chang area, China. Environ Earth Sci. 61(6):1135–1143.
- Zhao Q, Wang L. 1995. Study on geomechanical simulation of tectonic gravity spreading mechanism. J Eng Geol. 3:21–27.
- Zong K. 2005. Research on ground fissure disaster in Su-Xi-Chang Area. In: Survey CG, editor. Proceedings of the Geological Environment and Urban Development. Beijing: China University of Geosciences Press. 1–10.