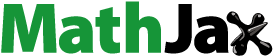
Abstract
This study measured the natural radioactivity of 226Ra, 232Th and 40K in 20 granite samples obtained from the Erongo Mountain Belt and Usakos Dome of the Erongo region, Namibia. All the samples were analysed using high purity germanium (HPGe) detector after 30-days to allow for secular equilibrium. The average activity concentrations of 226Ra, 232Th and 40K were 39.22 ± 3.79Bqkg−1, 35.76 ± 17.18Bqkg−1 and 1601.45 ± 212.18 Bqkg−1 respectively. The average activity concentrations of 226Ra, 232Th and 40K were used to estimate the average hazard indices and the results obtained further showed that radium equivalent activity, absorbed dose rate in air, external hazard indices (in and out), internal hazard index, external radioactivity index, internal radioactivity index, activity utilization index, annual effective dose equivalent (in and out door), exposure rate, and excess lifetime cancer risk were 213.85 Bqkg−1, 105.95 nGyh−1, 107.11 nGyh−1, 0.58, 0.29, 0.68, 0.82, 0.20, 1.77, 0.52 mSv.y−1, 0.13 mSv.y−1, 462.03 µRh−1 and 1.82 respectively. The results obtained revealed that the radiological hazard indices were lower than their respective acceptable critical values. However, the average value of effective dose equivalent (indoor) obtained was higher than the acceptable limit.
1. Introduction
Human population has always received natural radionuclides on a daily basis (Omeje et al. Citation2017). Humans are exposed to radiation externally through two main sources namely; terrestrial gamma rays and cosmic rays. Terrestrial gamma radiation originates from natural radionuclides associated with 238U and 232Th decay series and 40K radionuclide, and are found in the earth’s crust (UNSCEAR Citation2000; Murty and Karunakara Citation2008; Psichoudaki and Papaefthymiou Citation2008). They building materials obtained from the earth’s crust (rocks and soils), which contain radionuclides such as Uranium and Thorium series (238U and 232Th) as well as radioactive isotope of Potassium (40K), which are emitters of either beta or alpha particles may be ingested or inhaled and can ultimately increase internal exposure. Moreover, other radiation emitters may emit gamma radiation following their nuclear decay (Sharma et al. Citation2016; Alshammari et al. Citation2017). Since natural radionuclides are not uniformly distributed, the knowledge of the natural radioactivity in building materials is important for the evaluation of population exposure to radiation, and as most individuals spend more than 80% of their time indoors, the internal and external radiation exposures from building materials create prolonged exposure situations (ICRP Citation1999; Lu and Zhang Citation2007).
Granite is an intrusive igneous rock that is strongly enriched with Uranium (U) and Thorium (Th) (on an average content, 5 ppm Uranium and 15 ppm Thorium) (Faure Citation1986; Ménager et al. Citation1994). For centuries, granite has been widely used in building construction and architectural design. Granitic materials are exteriorly used in building bridges, paving and monuments (El-Taher et al. Citation2004). In addition, interior indoor polished granite slabs and tiles are used in countertops, tile floor and stair treads (Allam et al. Citation2013). In building construction, the existence of natural radionuclides in the granite may lead to undesirable radiation exposure to the public (Omeje et al. Citation2018). Most worrisome is the fact that the concentrations of 238U, 232Th and 40K in building material such as granite vary depending on the local geological and geographical conditions as well as the geochemical characteristics of the parent materials (Joel et al. Citation2018). A large number of studies carried out in the Erongo region of Namibia have shown that some parts of the Erongo region have high background radiation (Zivuku et al. Citation2016; Onjefu et al. Citation2017; Zivuku et al. Citation2018).
Thus, in this study, the radiological analysis of granite samples collected from Erongo granite belt and Usakos dome was carried out to assess the radiation exposure risk associated with the use of that granite which has found wide applications as building and ornamental materials in Namibia and sub region.
2. Methods and materials
2.1. Study area
The study area is located along the D1935 road and north of Usakos town in the Erongo Region, western central Namibia (). Earlier study indicated that the granite covered in this study falls within the Central Zone of the Damara orogen, Namibia. The granite in the area forms part of the regionally widespread granite suite that intruded the local landscape of amphibolite-facies rocks of the Damara Super-group at ca. 550-540 Ma (Miller and Mc Citation1983). It is a product of millions of years of geological evolution of continental mass collisions, melting and cooling processes. The earliest granites are represented by biotite-rich megacrystic granites, followed by leucocratic megacrystic granites and a final stage of voluminous, garnetiferous and tourmaline-bearing, medium-grained leucogranites (Ostendorf and Jung Citation2010).
Granites are key sources of modern raw material-base and supports for both large and small-scale building industries in Namibia. The different granite-based industries in Namibia include:
Large-scale uranium mining such as the Rössing Uranium Mine;
Medium size dimension stone mining widely used for kitchen decorations, table tops, tiles, building interior and exterior walls cladding, tombstones, monuments as well as various construction and decorative applications of granite-based fragments, and;
Small-scale gemstones mining widely used in granite landscapes.
Granites are also part of the natural landscape and underground conditions where houses, settlements and towns are built, and the fractured granite terrains are a great source of groundwater supply for rural communities in the arid and semiarid regions of Namibia including the Usakos area.
The industrial and socio-economic significance of these granite rocks in Namibia clearly show that the people are in constant contact with these rocks at various levels of the society. In addition to their various minerals compositions, granites are also a source of radioactivity linked to their contents of some accessory minerals such as uranium, thorium, and potassium. Thus, it is expedient to determine the levels of radioactivity associated with granites, such as the Erongo granite which found wide applications under different local conditions, and advise on its suitability as building material based on regulatory guideline limits.
2.2. Samples collection and preparations
In this study, 20 samples of granites were collected from two different mining areas in the Erongo region: Erongo mountain belt and Usakos dome as listed in . Each labelled sample was crushed and ground to small powder in an agate motor separately. After which 1 kg in weight were dried in an oven at about 110 °C to ensure the complete removal of moisture content. The oven-dried samples were transferred into 500 ml Marinelli beakers, firmly sealed, and stored for four weeks to ensure secular equilibrium is attained (Onjefu et al. Citation2017).
Table 1. 226Ra, 232Th and 40K concentrations in different granite samples.
2.3. Gamma spectroscopy analysis of the radionuclides
The counting time for each prepared sample was measured for 50,000–100,000 s depending on the concentration of the radionuclides using the coaxial high purity germanium (HPGe) detector (ORTEC model GC4520 SN 10882) with a relative efficiency of 45% and full width at half maximum (FWHM) of 1.7 kev energy resolution for the 1332 kev gamma ray line of 60Co. The spectrum was analyzed using Genie 2000 software made by Canberra Industries Inc, USA. The detector is 10-cm thickness lead to reduce the background radiation from other sources.
The gamma spectrometry system was energy calibrated using a range of gamma-ray energies from 0.060 MeV to 2 MeV, and efficiency calibrated using a mixed radionuclides standard in a 500 ml Marinelli beaker, counted for 12 hours. The energy range were analysed for absolute photo-peak efficiency and energy calibration of the HPGe detector using a multi-nuclide calibration standard with an initial activity of 40 kBq homogeneously distributed in silicone matrix, supplied by Eckert & Ziegler Nuclitec GmbH, Germany, SN. AM 5599.
Energy calibration was performed by matching the gamma energy peaks in the spectrum of the reference standard with the spectrometer channel number. The centroid channels and corresponding radionuclide energy peaks were then recorded and used to make a calibration curve of Energy versus Channel Number. The calibration curve performed through the counting of standard radionuclides with known activities and gamma energy peaks from 60 keV to 2000 keV.
The efficiency calibration was validated by running the same standard and analysed against certified activity. The mathematical efficiency was calculated from EquationEquation 1(1)
(1) .
(1)
(1)
where NT represents the total counts under a photopeak, NB denotes the background count, PE is the gamma ray yield, ASTD represents the activity of calibration standard during the time of measurement in Becquerels (Bq), while TSTD represents the counting time of the standard.
The energy calibration and relative efficiency calibration in this study used the gamma sources of 60Co and 40K because of the wide range of gamma-ray energies emitted over the entire energy range of interest. The activity concentration of 226Ra was determined as the weighted mean from the average concentrations of 214Pb gamma transition of 295.21 keV and 351.92 keV and 214 Bi gamma transition of 609.32 keV. Similarly, the gamma-ray lines of 212Pb (238.63 keV), 208Ti (583 keV), 228Ac (911 keV) and 228Ac (969 keV) were employed for the activity concentration for 232Th. The activity concentration of 40K was determined by its single gamma transition of 1461 keV. Each radionuclide activity was obtained using EquationEquation 2(2)
(2) (IAEA Citation1989a):
(2)
(2)
where
is the activity concentration in Bq.kg – 1of each radionuclide in the granite sample,
represent the net number of count in the photo peak,
is the detector efficiency of the specific gamma-ray,
is the intensity at the corresponding gamma-ray energy,
is the time taken for each sample to be counted in seconds and
denotes the mass of the sample in kg.
3. Results and discussion
3.1. Radioactivity concentrations
The distribution of natural radionuclides in granite samples is presented in . The highest activities concentrations of 226Ra, 232Th, and 40K in the granite samples are 106.46 9.15
(Usakos Dome), 207.54
17.18
(Usakos Dome), and 2306.98
301.51
(Usakos Dome), respectively, while the lowest result obtained in the same granite samples were 10.78
1.01
(Usakos Dome), 2.07
0.34
(Erongo Mountain Belt), and 893.67
117.06
(Erongo Mountain Belt) respectively. The average value of 226Ra is 39.22
3.79
and it was found to be 0.78 time lower than the permissible maximum value of 50
(UNSCEAR Citation2008). For 232Th, the average value obtained was 35.76
3.16
and is lower than the allowed value of 50
by a factor of 0.72 (UNSCEAR Citation2008). However, the average value of 40K (1601.45
212.18) was higher than the permissible maximum value of 500 by a factor of 3.20 (UNSCEAR Citation2008; Sahin Citation2018). The higher average value of 40K may be attributed to the chemical composition and formation of molten rock with a simultaneous sedimentation of mineral particles containing uranium and thorium, which may have informed the elevated activity concentrations (Omeje et al. Citation2018).
The average activity concentrations of 226Ra, 232Th, and 40K in the samples follow the order of 40K 226Ra
232Th. This result is consistent with the finding of an earlier study by Omeje et al. (Citation2018).The results of the activity concentrations of 226Ra and 232Th in this work are lower than the results for granite in Turkey, Egypt, Greece and Brazil (Moura et al. Citation2011; Amin Citation2012; Onargan et al. Citation2012; Papadopoulos et al. Citation2013). However, the concentration of 40K was higher than the results of 40K published in these countries. The results of the correlation analyses between the average activity concentrations of 226Ra, 232Th, and 40K as shown in revealed weak positive correlations with respective coefficient of (R2 = 0.01) for 40K and 226Ra, (R2 = 0.184) for 40K and 232Th, and (R2 = 0.09) for 232Th and 226Ra. The weak positive correlations indicate that while the activity concentrations of these radionuclides tend to go up in response to one another, the relationship between them is not very strong and hence, could be attributed to their varying sources of inputs in the granite.
3.2. Radiological health risk parameters
3.2.1. Radium equivalent (Raeq) activity
In order to overcome the non-uniformity of radionuclides 226Ra, 232Th, and 40K in natural samples, the radium equivalent activity index was used.
activities for all the samples were obtained on the basis of the estimation of 370
of 226Ra, 259
of 232Th, and 4810
of 40K (Beck Citation1980; Krieger Citation1981; Beretka Citation1985; IAEA 1989b; Papadopoulos et al. Citation2013). The
measured in
was calculated using EquationEquation 3
(3)
(3) :
(3)
(3)
where
and
are the specific activities of 226Ra, 232Th, and 40K measured in
respectively. The
values obtained in this study varied from the maximum concentration of 564.42
in Usakos Dome to the minimum concentration of 108.35
in Erongo Mountain Belt, with an average concentration value of 213.85
Although the average concentration of the
in this study is below the critical value of 370
values from UD-14, UD-16 and UD-17 exceeded the recommended limit of 370
(UNSCEAR Citation2000). On comparing between Erongo Mountain Belt (EMB) and Usakos Dome (UD), it was observed that values of
obtained for EMB were lower than those obtained for UD (). This could be attributed to the high activity concentrations of radionuclides distribution in the granite samples from Usakos Dome. The granite samples from Usakos Dome contain more of these radioactive elements than those of EMB, and this depends on the chemical composition and formation of the parent rock. Geologists provide an explanation of this behaviour in the course of partial melting and fractional crystallization of magma (Aquino and Pecequilo Citation2015). The correlation analysis between
and 226Ra activity concentration also indicated weak positive correlation with coefficient of R2 = 0.223 as shown in .
Table 2. Radium equivalent indoor absorbed dose rate in air
outdoor absorbed dose rate in air
, indoor external hazard index
outdoor external hazard index
and internal hazard index
recorded in the different granite samples.
3.2.2. Absorbed gamma dose rate in air (
)
The terrestrial absorbed dose rates due to terrestrial gamma rays at 1 m above the ground surface were evaluated from the activity concentrations of 226Ra, 232Th, and 40K using the conversion factors (0.462, 0.604 and 0.0417, respectively), as given by UNSCEAR (UNSCEAR Citation2000) and Sahin (UNSCEAR Citation2008). The indoor absorbed dose rate in air measured in unit of
was calculated using EquationEquation 4
(4)
(4) (UNSCEAR Citation2008):
(4)
(4)
The calculated indoor absorbed gamma dose rate in air ranged between 55.96 and 261.84
All the present
values, except for seven granite samples (EMB-1, EMB-2, EMB-3, EMB-4, EMB-6, EMB-7, EMB-8), are higher than the critical value of 80
(). This suggests that people using this samples will receive the excessive gamma radiation that may demand further attention from radiation safety point of view.
The conversion coefficients used to evaluate the outdoor absorbed gamma dose rate in air per unit radioactivity concentration of 226Ra, 232Th, and 40K in
was calculated using the relationship in EquationEquation 5
(5)
(5) (UNSCEAR Citation2008):
(5)
(5)
The outdoor absorbed dose rate recorded in this study varied from 56.05 to 265.37
The average value of
due to the presence of 226Ra, 232Th, and 40K in the samples was 107.11
This value is higher than the critical value of 57
by a factor of 1.9 () owing to the contribution from some granite rock samples of high thorium and potassium content. Again, these contributions are consistent with those obtained for indoor absorbed dose rate in air.
3.2.3. External hazard index 

The external hazard index calculated from the activity concentrations of 226Ra, 232Th, and 40K determined in the present study is shown in . The
was calculated for two cases. Case 1,
is a scenario for a room in the house where the inhabitants live with infinitely thick walls without windows and doors for good ventilation (Cox Citation1991; Al-Zahrani Citation2017). Case 2,
is a scenario for a room in the house where the inhabitants live with infinitely thick walls having windows and doors for proper ventilation (Darwish et al. Citation2015; Maxwell et al. Citation2018).
and
were estimated using EquationEquations 6
(6)
(6) and Equation7
(7)
(7) respectively.
(6)
(6)
(7)
(7)
where
and
are the average activity concentrations of 226Ra, 232Th, and 40K in
respectively.
For the radiation hazard to be acceptable, it is recommended that the values of and
be less than unity (Baykara et al. Citation2011; Allam et al. Citation2013). The calculated values of
in all samples from the Erongo Mountain Belt are lower than the 1 limit value. However, the values of
in Usakos Dome UD-14 and UD-16 exhibited higher values greater than 1. It may be due to the geological formation of UD-14 and UD-16. The estimated
for all the samples varied from 0.15 to 0.76 with an average value of 0.29.
3.2.4. Internal hazard index 

The internal hazard index for this present study is shown in . This index gives the internal exposure to radon and its progeny and was calculated using EquationEquation 8(8)
(8) (Bendibbie et al. Citation2013; Papadopoulos et al. Citation2013):
(8)
(8)
For a building material to be considered radiologically safe, the internal hazard must be less than 1 (Xinwei Citation2005; Ghose et al. Citation2012; Onjefu et al. Citation2017). In the present study, all the values of are less than 1 except EMB-9, UD-14, UD-16 and UD-17 where the samples exhibited higher values than the 1 limit value, due to the high activity concentrations of radionuclides distribution in the samples. This suggests that the granite samples are not radiologically safe to use in building construction without restrictions because of its 40K and 232Th content that were twice as much as the average values.
3.3.5. External radioactivity level index 

The external radioactivity level index which give the distribution of the values of the gamma index for the building materials measured in this study are presented in . The recommended critical value is unity (UNSCEAR Citation2008). According to European Commission Radiation Protection Report (EC-RP 112), controls on the activity concentrations of any material employed for building purposes can be based on the dose criterion and exemption level. Effective dose exceeding the dose criterion of 1 mSv.y−1 should be important in terms of radiation protection and safety (Kant et al. Citation2006; UNSCEAR Citation2008; Alharbi et al. Citation2011; Sahin Citation2018).
Table 3. External radioactivity level index internal radioactivity level index
activity utilization index
indoor annual effective dose equivalent
outdoor annual effective dose equivalent
exposure rate
and excess lifetime cancer risk
of the granite samples.
The external radioactivity level index is calculated using EquationEquation 9
(9)
(9) (EC Citation1999):
(9)
(9)
As shown in , the values of ranged from 0.44 to 2.09 with an average value of 0.82. Clearly, the results of the calculated values of
in the samples shows higher values for samples UD-14, UD-16, and UD-17 than the recommended limit of unity. These high values may be attributed to the kind of soil, rock and geological formation of the samples (UNSCEAR Citation2008).
3.3.6. Internal radioactivity level index 

The internal radioactivity index is an important index associated with excess alpha radiation from radon emanating from building materials. If the radium activity level in building material exceeds the values of 200
there may be radon build up exceeding 200
The International Commission on Radiation Protection recommended an action level of 200
for radon in dwellings structures (Kant et al. Citation2006; Uosif et al. Citation2014; Omeje et al. Citation2018). Similarly, radium activity levels below 100
is indicative of radon exhalation from building materials which may not cause indoor concentration greater than 200
(ICRP Citation1994). The critical value of
is 1 and is calculated from Equationequation 10
(10)
(10) (UNSCEAR Citation2008; Xinwei and Xiaolon Citation2008):
(10)
(10)
The calculated results of in the present study varied from 0.05 to 0.53 with an average value of 0.20. This results obtained for
suggest that all the samples have values smaller than the recommended limit of 1 ().
3.3.7. Activity utilization index 

The natural radionuclides activity concentrations (226Ra, 232Th, and 40K) in building materials are the main contributors that affect the indoor absorbed dose. The calculation of the activity utilization index involves the combination of the activity concentrations of 226Ra, 232Th, and 40K and is evaluated using EquationEquation 11(11)
(11) (UNSCEAR Citation2000):
(11)
(11)
where
and
are fractional percentages to the total dose rate from 226Ra, 232Th, and 40K, respectively (
) (UNSCEAR Citation2008). The activity utilization index is 2 by definition and it implies a dose rate of 80 nGy h −1 (UNSCEAR Citation2000). As presented in , activity utilization index
of the radionuclides studied varied from 0.97 to 4.01. The highest value of 4.01 was recorded at Usakos Dome-14 and the lowest value of 0.97 was noted in Erongo Mountain Belt. However, all the samples recorded activity utilization index below the critical value of 2 except for UD-14, UD-16 and UD-17 respectively with values above 2.
2.3.8. Annual effective dose equivalent 

The indoor and outdoor annual effective dose equivalent is estimated from the indoor absorbed dose rate in air and outdoor absorbed gamma dose rate in air
the occupancy factors of 80% and 20% and 8766 h in a year.
in this present study was determined using the relation in EquationEquations 12
(12)
(12) and Equation13
(13)
(13) below:
For indoor:
(12)
(12)
For outdoor:
(13)
(13)
where
is the conversion factor used to convert the absorbed dose in air to effective dose received by the adult (Uosif et al. Citation2014).
The values of ranged from 0.27 to 1.29
for indoor and 0.06 to 0.33
for outdoor. The average values were found to be 0.52
and 0.13
for indoor and outdoor respectively. The average value of
is higher than the critical value by a factor of 1.08 while the value of
is lower than the critical value by a factor of 0.27. The high average value of
may be attributed to the presence of relatively high amount of radioactivity in some monitoring locations. The variation of natural radioactivity levels at the different sampling sites was due to the variation of concentrations of radionuclides in the geological formations. Hence, the variation in the AEDE. According to Kinyua et al. (Citation2011), younger granites represent the highest elevation while the older rock is relatively low. The presence of such high values in younger granites may be attributed to the presence of relatively increased amount of accessory minerals such as zircon, iron oxides, fluorite and other radioactive related minerals .
3.3.9. Exposure rate 

The exposure rate was estimated using the relation in EquationEquation 14(14)
(14) (Kinyua et al. Citation2011).
(14)
(14)
The calculated values of are presented in and the results ranged from 239 to 1162
with an average value of 462.03
All the samples were below the recommended limit. However, samples UD-14, UD-16 and UD-17 displayed a higher value than the critical limit of 600
Again, these values are consistent with those of internal and external hazard indices, activity utilization index, and the internal and external radioactivity indices. Therefore, the samples may pose an internal radiological risk when used as building material.
3.3.10. Excess lifetime cancer risk 

Excess lifetime cancer risk (ELCR) was evaluated by using the relation in EquationEquation 15(15)
(15) :
(15)
(15)
where DL is the duration of life taken to be 70 years and RF is risk factor (Sv−1) fatal cancer risk per Sievert. For stochastic effect, (ICRP 60) employs the value of 0.05 for the general public (UNSCEAR Citation2000; Akhtar et al. Citation2005; Taskin et al. Citation2009). The calculated values of ELCR in the present study are presented as . The mean value for ELCR was estimated and found to be below the recommended limit of 3.75 × 10 −3 (UNSCEAR Citation2000). However, the values of samples UD-14, UD-16 and UD-17 were above the critical value. This might constitute population exposure concerns from the three-sampled points, as granites obtained from the study area are valuable building materials widely used in Namibia. It has been identified that long-term exposure to radiation has some risks of causing cancer (Holm and Ballestra Citation1989). Radiation exposure can cause cancer in any living tissue, but high-dose whole-body external exposure is mostly associated with leukemia (Ménager et al. Citation1994). This is even as research report has shown that in today's world, cancer is a major disease for communities (Ibikunle et al. Citation2018) and that one of the cancer reasons is the radiation effect on biological cell (US EPA Citation1999; Abbasi Citation2017; Abbasi and Bashiry Citation2020). Elsewhere in the United States of America, report from Surveillance, Epidemiology, and End Results (SEER) Cancer Statistics Review by the National Cancer institute showed that American men have a 44% lifetime risk of cancer, while their women have a 38% lifetime risk (Holm and Ballestra Citation1989). The review further showed that long time exposure to cancer-causing materials resulted in additional risk that someone might have cancer in a lifetime (Holm and Ballestra Citation1989). This is a major concern in the study area as the radiation-bearing granites are utilized in different forms for building houses where humans live for their lifetime.
4. Conclusion
The levels of natural radioactivity of 226Ra, 232Th and 40K in granite samples collected from Erongo granite belt and Usakos dome were determined using gamma ray analysis, and to assess the radiation exposure risk associated with the use of the granite widely used as building and ornamental materials in Namibia and the sub region. Based on the results obtained, the average activity concentrations of 226Ra and 232Th in the granite samples were below their maximum permissible values for the protection of human health. However, the average activity concentration of 40K in the granite samples was higher than its acceptable health regulatory limit. In particular, the calculated radiological parameters for the granite samples UD-14, UD-16 and UD-17 exceeded their health regulatory limits and thus, present radiological exposure risk to humans in using the granite as building and ornamental materials. It is therefore recommended that all appropriate regulatory measures in compliance with the basic safety standards to minimize indoor exposure to natural radionuclides should be adopted to screen the granite in the study area prior to its use as building and ornamental materials. This could help towards devising practical approaches aimed at minimizing natural radiation exposure associated with the use of the granite as building materials.
Acknowledgements
We acknowledge the Namibia University of Science and Technology for the financial support provided to carry out this study. We are also thankful to the Risk based Solution Limited, Windhoek for providing transport and logistic to facilitate the fieldwork of the study. Finally, we thank Department of Natural and Applied Sciences, Namibia University of Science and Technology for availing the High Purity Germanium Detector used for the spectrometric analysis.
Disclosure statement
No potential conflict of interest was reported by the authors.
References
- Abbasi A. 2017. Modeling of lung cancer risk due to radon exhalation of granite stone in dwelling houses. J Cancer Res Ther. 13(2):208–212.
- Abbasi A, Bashiry V. 2020. Estimation of cancer risk due to radiation exposure. J Cancer Res Therapeut.
- Akhtar N, Tufail M, Ashraf M, Mohsin Iqbal M. 2005. Measurements of environmental radioactivity for estimation of radiation exposure from saline soil of Lahore, Pakistan. Radiat Meas. 39(1):11–14.
- Alharbi WR, Al Zahrani JH, Abbady AG. 2011. Assessment of radiation hazard indices from granite rocks of the southeastern Arabian Shield, kingdom of Saudi Arabia. Aust J Basic Appl Sci. 5:672–682.
- Allam KA, Ramadan AA, Taha A. 2013. Dose assessment for natural radioactivity resulting from tiling granite rocks. Radiat Prot Environ. 36(3):99–105.
- Alshammari H, Algammidi A, Algammidi A. 2017. The experimental gamma radiation dose rate for radiation hazard into adhesive building materials in Saudi Arabia. OJRad. 07(04):272–291.
- Al-Zahrani JH. 2017. Estimation of natural radioactivity in local and imported polished granite used as building materials in Saudi Arabia. J Radiat Res Appl Sci. 10(3):241–245.
- Amin RM. 2012. Gamma radiation measurements of naturally occurring radioactive samples from commercial Egyptian granites. Environ Earth Sci. 67(3):771–775.
- Aquino RRD, Pecequilo BRS. 2015. Activity concentrations of natural radionuclides in commercial granite samples from Espirito Santo State, Brazil. Int Nuclear Saf J. 4(3):53–58.
- Baykara O, Karatepe Ş, Doğru M. 2011. Assessment of natural radioactivity and radiological hazards in construction materials used in Elazig, Turkey. Radiat Meas. 46(1):153–158.
- Beck HL. 1980. Exposure rate conversion factors for radionuclides deposited on the ground. Department of Energy, Environmental Measurements Lab, New York.
- Bendibbie MM, Maina DM, Jp P. 2013. Radiological analysis of suitability of Kitui South limestone for use as building material. Int J Fund Phys Sci. 3(2):32–35.
- Beretka J, Matthew PJ. 1985. Natural radioactivity of Australian building materials, industrial waste sand by-products. Health Phys. 48:87–95..
- Cox PA. 1991. The elements: the origin, abundance and distribution. Oxford (UK): Oxford University Press.
- Darwish DAE, Abul-Nasr KTM, Khayatt AM. 2015. The assessment of natural radioactivity and its associated radiological hazards and dose parameters in granite samples from South Sinai, Egypt. J Radiat Res Appl Sci. 8(1):17–25.
- EC (European Commission). 1999. Radiological protection principles concerning the natural radioactivity of building materials. Radiation Protection 112. Directorate General Environment, Nuclear safety and Civil Protection. Geneva.
- El-Taher A, Uosif MAM, Orabi AA. 2004. Natural radioactivity levels and radiation hazard indices in granite from Aswan to Wadi El-Allaqi South Eastern Desert, Egypt VII Radiation Physics & Protection Conference, Ismailia-Egypt; 27–30 November 2004.
- Faure G. 1986. Principles of isotope geology. 2nd ed. New York (NY): John Wiley & Sons.
- Ghose S, Kh A, Zaman N. 2012. Radiological significance of marble used for construction of dwellings in Bangladesh. Radioprot. 47(1):105–118.
- Holm E, Ballestra S. 1989. Measurement of radionuclides in food and the environment, a guidebook. IAEA Tech. Rept. Vienna.
- IAEA (International Atomic Energy Agency). 1989a. Construction and use of calibration facilities for radiometric field equipment. Technical Reports Series no.309, IAEA, Vienna.
- IAEA (International Atomic Energy Agency). 1989b. IAEA. Measurement of radionuclides in food and environment. Technical Report Series No. 295, Vienna.
- Ibikunle SB, Arogunjo AM, Ajayi OS. 2018. Characterization of radiation dose and excess lifetime cancer risk due to natural radionuclides in soils from some cities in Southwestern Nigeria. J Forensic Sci Criminal Inves. 10(4):555793.
- ICRP (International Commission on Radiological Protection). 1994. Protection against Rn-222 at home and at work. ICRP Publication 65. Ann ICRP. 23(2):1–48.
- ICRP (International Commission on Radiological Protection). 1999. Protection of the public in situation of prolonged radiation exposure. Publication 82, Ann. ICRP. 29(1-2)). Elsevier Sciences, B.V. International Commission on Radiological Protection.
- Joel ES, Maxwell O, Adewoyin OO, Ehi-Eromosele CO, Embong Z, Saeed MA. 2018. Assessment of natural radionuclides and its radiological hazards from tiles made in Nigeria. Radiat Phys Chem. 144:43–47.
- Kant K, Upadhyay SB, Sonkawade RG. 2006. Radiological risk assessment of phosphate fertilizers in soil. Iranian J Radiat Res. 4(2):63–70.
- Kinyua R, Atambo VO, Ongeri RM. 2011. Activity concentrations of 40K, 232Th, 226Ra and radiation exposure levels in the Tabaka soapstone quarries of the Kisii Region, Kenya. Afr J Environ Sci Technol. 5(9):682–688.
- Krieger R. 1981. Radioactivity of construction materials. Betonwerk Fertigteil Techn. 47:468–473.
- Lu X, Zhang X. 2007. Radionuclide content and associated radiation hazards of building materials and by-products in Baoji, China. Radiat Prot Dosim. 128(4):471–476.
- Maxwell O, Adewoyin OO, Joel ES, Ehi-Eromosele CO, Akinwumi SA, Usikalu MR, Emenike CP, Embong Z. 2018. Radiation exposure to dwellers due to naturally occurring radionuclides found in selected commercial building materials sold in Nigeria. J Radiat Res Appl Sci. 11(3):225–227..
- Ménager ΜT, Heath MJ, Ivanovich M, Montjotin C, Barillon R, Camp J, Hasler SE. 1994. Igration of uranium from uranium mineralized fractures into the rock matrix in granite. Radiochim Acta. 66-67(s1):47–83.
- Miller R, Mc G. 1983. The Pan-African Damara Orogen of South West Africa/Namibia. Spec Publ Geol Soc S Afr. 11:431–515.
- Moura CL, Artur AC, Bonotto DM, Guedes S, Martinelli CD. 2011. Natural radioactivity and radon exhalation rate in Brazilian igneous rocks. Appl Radiat Isot. 69(7):1094–1099.
- Murty VRK, Karunakara N. 2008. Natural radioactivity in the soil samples of Botswana. Radiat Meas. 43(9-10):1541–1545.
- Omeje M, Adewoyin OO, Joel ES. 2018. Natural radioactivity concentration of 226Ra, 232Th, and 40K in commercial building materials and their lifetime cancer risk assessment in dwellers. Hum Ecol Risk Assess. 24(8):2036–2053.
- Omeje M, Olusegun OA, Joel ES, Ehi-Eromosele CO, Emenike CP, Usikalu MR, Akinwumi SA, Zaidi E, Saeed MA. 2017. The role of science in novel research and advances in technology. Proceedings of International Conference on Science and Sustainable Development (ICSSD), Center for Research, Innovation and Discovery, Covenant University, Nigeria, J Inform and Math Sci. 9(2): p. 423–436.
- Onargan T, Gür F, Kaya E, Güneri S. 2012. Assessment of natural radioactivity in commercial granites used in Turkey. J Environ Sci Health A Tox Hazard Subst Environ Eng. 47(12):1825–1830.
- Onjefu SA, Taole SH, Kgabi NA, et al. 2017. Assessment of natural radionuclide distribution in shore sediment samples collected from the North Dune beach, Henties bay, Namibia. J Radiat Res Appl Sci. 10(4):201–306.
- Ostendorf J, Jung S. 2010. Petrogenesis of syn-orogenic basement-derived granites (Central Damara Orogen, Namibia): insights from Nd, Sr and Pb isotopes.
- Papadopoulos A, Christofides G, Koroneos A, Papadopoulou L, Papastefanou C, Stoulos S. 2013. Natural radioactivity and radiation index of the major plutonic bodies in Greece. J Environ Radioact. 124:227–238.
- Psichoudaki M, Papaefthymiou H. 2008. Natural radioactivity measurements in the city of Ptolemais (Northern Greece). J Environ Radioact. 99(7):1011–1017.
- Sahin SB. 2018. The determination of concentrations of radioisotopes in some granite samples in Turkey and their radiation hazards. Radiat Eff Defects Solids.. 173(5-6): 353-366
- Sharma N, Singh J, Esakki SC, Tripathi RM. 2016. A study of the natural radioactivity and radon exhalation rate in some cements used in India and its radiological significance. J Radiat Res Appl Sci. 9(1):47–56.
- Taskin H, Karavus M, Ay P, Topuzoglu A, Hidiroglu S, Karahan G. 2009. Radionuclide concentrations in soil and lifetime cancer risk due to gamma radioactivity in Kirklareli, Turkey. J Environ Radioact. 100(1):49–53..
- Tufail M, Nasim A, Sabiha J, et al. 2007. Natural radioactivity hazards of building bricks fabricated from soil of two districts of Pakistan. J Radiol Prot. 27:481–492. e
- UNSCEAR (United Nation Scientific Committee on the effects of Atomic Radiation). 2000. Sources, effect and risks of ionizing radiation. Report to the General Assembly with Scientific Annexes. United Nations, New York.
- UNSCEAR (United Nation Scientific Committee on the effects of Atomic Radiation). 2008. Sources, effect and risks of ionizing radiation. Report to the United Nations Scientific Committee on the Effects of Atomic Radiation: Fifty-Sixth Session. No.46. United Nations, New York.
- Uosif MA, Issa SAM, Ebrahim AA. 2014. Determination of natural radioactivity in building raw materials from the quarries of Assiut Cement Company, Assiut, Egypt. Int J New Horizons Phys. 1(1):25–32.
- US EPA (US Energy Protection Agency). 1999. Cancer risk coefficients for environmental exposure to radionuclides. Federal Guidance Report. EPA 402-R-99-001. Vol. 13. Tennessee, US Energy Protection Agency; p. 212–228.
- Xinwei L. 2005. Natural radioactivity in some building materials of Xi’an, China. Radiat Meas. 40(1):94–97.
- Xinwei L, Xiaolon Z. 2008. Natural radioactivity measurements in rock samples of Chihua Mountain National Geological Park, China. Radiat Pro Dosim. 128:77–82.
- Zivuku M, Kgabi NA, Tshivhase VM. 2016. Elemental concentration of natural occurring radioactivity materials in soil nearby uranium sites of Erongo region, Namibia. Eur J Sci Res. 4:402–410.
- Zivuku M, Kgabi NA, Vm T. 2018. Excess lifetime cancer risk due to natural radioactivity in soils: Case of Karibib town in Namibia. Afr Rev Phys. 13:0012.