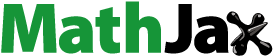
Abstract
Response characteristics of key strata during the periodic weighting process in Dongjiahe coal mine were investigated using micro-seismic (MS) monitoring. Fracture types of key strata were identified through the moment tensor inversion. Finally, fracture mechanism of roof key strata was revealed from a microscopic viewpoint. The results show that there are three concentrations of MS events distributed along the dip direction. And two MS event clusters were formed in “pairs” before and behind the working face. Along the strike direction, tensile and shear factures occur in front of the working face with a dip angle of 54°, while the micro-fractures are mainly mixed and partial shear types behind the working face. By contrast, the rock fracture usually occurs behind the working face along the dip direction. The micro-fractures are mainly tensile and mixed types before fracturing compared to mainly mixed and shear fractures with slight tensile ones after fracturing. The fracture pattern basically conforms to the “O-X” type. The X-shaped fracture extends outward with an angle of 46° with the due north. The findings in this paper provide a theoretical reference for strata behaviours and hazard risk assessment under similar conditions.
1. Introduction
The roof strata movement is the main reason for strata pressure behaviour. The fracture and failure of key strata play a vital role in the movement state of overburden during the coal mining process (Kang et al. Citation2018; Lu et al. Citation2019; Wang et al. Citation2019). The fracture of key strata can also lead to dynamic disasters, such as coal and gas outburst, rock burst, water inrush, roof collapse and ground subsidence, which severely threaten mining safety. Therefore, it is of great significance to investigate the fracture pattern and process of roof key strata in the stope stress distribution analysis and mine disaster prevention and control (Cao et al. Citation2018; Cheng et al. Citation2020).
The stability - instability - restability process of key strata structure controls the stability or failure states of overlying strata (Qian Citation1982). At present, there are many studies focusing on the key strata behaviours in the coal mining through theoretical modelling and analysis (He et al. Citation2015; Xu et al. Citation2015; Jian Citation2017; Xie and Xu Citation2017; Guo et al. Citation2019; Jiang et al. Citation2019; Wang et al. Citation2019). Jiang et al. (Citation2019) revealed the fracture mechanism of hard thick igneous key strata and calculated fracture step distance through the energy method and yield line theory assuming plate yield limit model with three sides fixed and one side simply supported. Wang et al. (Citation2019) established a theoretical model combining the key stratum and voussoir beam structure and analyzed effects of arch structure in unconsolidated layers on overlying strata breakage. He et al. (Citation2015) found there were three mechanisms of multi key strata destabilization, including shear slip of high subkey strata, rotation and slip of low subkey strata and rupture of main key strata. Xu et al. (Citation2015) numerically investigated the fracturing mechanism of key strata induced by mining and analyzed effects of key strata rupture on abutment stress. Xie and Xu (Citation2017) numerically simulated and researched effects of key layers with different thicknesses and heights on the abutment stress distribution. Jian (Citation2017) theoretically investigated the stability of the overlying strata in solid dense filling mining based on the theory of key strata. Guo et al. (Citation2019) studied the height of fractured area at the high-intensity mining panel using theoretical modes of strata overhanging stability and suspended integrity. These theoretical models focus on the law of overburden movement under general and special geological conditions, and provide guidance to mining production from the theoretical level.
To understand the fracture and failure state of overlying strata intuitively, many monitoring methods, e.g., borehole digital photography, water level observation and micro-seismic (MS) monitoring, have been introduced into the coal mining. Seismic hazard assessment was presented using regional seismotectonic studies (Mukhopadhyay et al. Citation2010; Baro et al. Citation2018). As a three-dimensional real-time monitoring method, MS monitoring can reflect the whole process from the crack initiation to final failure of rock mass from a microscopic perspective (Gibowicz et al. Citation1991; Gibowicz and Kijko Citation1994; Xu et al. Citation2011; Zhang et al. Citation2016; Zhou et al. Citation2017; Guo et al. Citation2019). Dou et al. (Citation2018) established an MS multi-parameter index system for the early warning of coal-rock dynamic failure and determining the weights of each index with the R-value scoring method. Li et al. (Citation2016) carried out MS monitoring in Xiaojihan coal mine with high-intensity mining in Western China and analyzed the variation of MS parameters before or after large scale failure. Mondal et al. (Citation2020) investigated the roof strata behaviour in the destressed zone of a shallow longwall mine located in Central India using MS monitoring and borehole televiewer data. Cai et al. (Citation2019) stated MS technique is capable of direct measurement of energy release and indirect capture of stress or strain state changes. Cao et al. (Citation2020) discussed the relationship between MS features and mining activities in a Slovenian Coal Mine and believed pre-existing natural fractures in the coal seam dominates MS activities. Wu et al. (Citation2018) studied the movement and fracture type of hard and thick key stratum during the longwall mining process. Yu et al. (Citation2017) investigated the MS event distribution in front of or behind the working face during longwall top coal caving through a case study. Zhang et al. (Citation2019) studied the movement and fracture modes of multi-hard and thick roofs by mining activities using MS monitoring and proposed control measures for rock burst. Ma et al. (Citation2020) identified the underlying floor faults using MS monitoring and stress inversion approaches.
These above-mentioned studies revealed the structure form of roof key layer during the mining process and explained the mine pressure phenomena with the distribution of MS events and energy characteristics. However, most of these studies only focus on a certain state of the roof strata under the condition of stability or instability. In addition, it is also difficult to characterize the motion state and rupture mode of the rock strata in the rotation and subsidence after fracturing only by relying on the distribution and energy information of MS events.
In this study, a typical working face in Dongjiahe coal mine in China was selected to be a case study and real-time MS monitoring was conducted. Response characteristics of MS parameters were analyzed during the fracture-instability-restabilization process of multiple key strata of the roof. The fracture surface and pattern of key strata were studied with the movement of key strata based on the moment tensor inversion method. Finally, the fracture mechanism of key strata during a whole cycle of roof activity was revealed.
2. Geological conditions and micro-seismic monitoring
2.1. Geological conditions
The strike and dip lengths of the research working face are 1217 and 185 m, respectively. No.5 coal seam of Shanxi Formation is mainly mined. The thickness of the coal seam is between 2.5 and 4.1 m, with an average of 3.3 m. It should be noted that there is a thin coal belt with a thickness of 1.15–2.2 m in the range of 540 to 765 m from the transportation lane entrance. The dip angle of coal seam is approximately 3°, which is near horizontal. The immediate roof is the dark grey coarse siltstone with a thickness of 0.65 to 1.66 m, containing a small amount of muscovitize, pyrite nodules and plant fossils. The main roof is made of grey medium fine-grained sandstone with a thickness of 5.7–11.77 m, whose composition is mainly quartz, dark stone debris and mica with some pyrite nodules.
Lithology and thickness of roof strata are shown in . According to the key stratum theory, some hard and thick strata in the overburden rock strata play a vital role in roof stability and they control the deformation and fracture of soft strata between them. These hard and thick strata that play a vital role in the stability of overlying rock mass are called key strata (Qian et al. Citation1996).According to the distribution characteristics of overlying strata, there are three key strata in the roof, named low-level, middle-level and high-level key strata. According to the influence zone of coal mining, the overlying rock strata are divided by the immediate roof, low-level, middle-level and high-level strata.
Table 1. Lithology and thickness of roof strata.
2.2. Micro-seismic monitoring system
Real-time monitoring and location of coal and rock ruptures affected by working face mining were made using the high-precision MS monitoring system, which is produced by Canadian ESG company. The system consists of MS sensors, digital signal acquisition and processing systems (Yuan et al. Citation2019). The MS sensor is a single axis geophone with a response frequency range of 15 to 1 000 Hz and a sensitivity of 43.3 V/m/s.
MS sensors are installed in the surrounding rock near the working face according to the mining and geological conditions. To be specific, one sensor is arranged every 60 m in the rich water area and one sensor is arranged every 100 m in other areas. The coordinates of MS sensors on the typical working face are listed in . The layout of MS sensors is shown in . The P- and S-wave velocities, which are employed in a homogeneous velocity model for the real-time location of MS events, were estimated by artificial blasting tests. The P- and S-wave velocities were 2800 and 1800 m/s after calibration. The location of MS events used first P-wave arrivals at 5–16 sensors and S-wave arrivals at 2–4. The location accuracy of the micro-seismic monitoring system is mostly within 10 m through analyzing the location errors of MS events.
Table 2. Coordinates of MS sensors on the typical working face.
3. Variation characteristics of microseismic (MS) parameters of key strata fracture
The MS monitoring results from October 15 to October 31, 2015, which corresponds to a cycle of roof activity, were used to analyze the initiation, propagation and coalescence process of micro fractures in the low-level and middle-level roof key strata. And then the relationship between the roof key stratum rupture and MS activities was established.
3.1. MS events and cumulative release energy
shows the number of MS events and the cumulative energy over time during the monitoring period. During October 15 to October 18, there was no mine pressure on the low-level and middle-level roof key strata and the daily MS events remained below 15, among which the cumulative energy released by MS events on October 18 increased slightly. From October 19 to October 21, periodic weighting occurred in the low-level key strata, and MS events rapidly increased to 19–25 per day. At the end of periodic weighting, the cumulative released energy suddenly increased slightly.
From October 22 to 23, the roof gradually tended to be stable after periodic weighting on low-level key strata and MS events gradually decreased to below 15 per day. The cumulative energy released by MS events on October 22 recovered to the level before the periodic weighting compared to a slight growth for October 23, which was related to the imminent rupture of the middle-level key strata.
During the period between October 24 and October 26, MS events sharply increased to the peak of 25 − 32 per day. This indicates there was mine pressure on middle-level key stratum and large-scale roof failure and strata movement occurred. It was found that on October 26 cumulative energy suddenly increased while there was no dramatic increase in the corresponding event number. Obviously, a single MS event with large energy was released and a MS event with an energy of 5040 J was captured in the coal pillar area at 11:38:41 on October 26, as shown in . From October 27 to October 31, MS event number and the cumulative released energy both recovered to the level before the mine pressure, which indicates the roof gradually stabilized.
According to the variation of MS event number and cumulative release energy during a cycle of roof activity, more than 15 events per day for two consecutive days or more can be used as the criterion for the periodic weighting on the roof. The energy released by MS events increased to different degrees before the key strata rupture and at the end of ground pressure, which could be used as the precursory information for the occurrence of periodic weighting. MS event number and cumulative energy for the fracture of middle-level key stratum were greater than those for low-level key stratum.
3.2. Distribution characteristics of moment magnitude
The moment magnitude of MS events with time is shown in . The moment magnitude during the whole monitoring period ranged from −1.3 to −0.2 and there were no MS events whose moment magnitude was greater than 0. This indicates the roof rock failure during the monitoring period was mainly caused by micro-fracture propagation. From October 15 to October 18, there was no ground pressure in the low-level and middle-level key strata. Only moment magnitudes of two MS event occurred on October 18 were greater than −0.5. From October 19 to October 21, the low-level key stratum was completely broken and periodic weighting occurred, and the maximum moment magnitude of daily MS events were all greater than −0.5. The period from October 22 to October 23 was a short period of roof re-stabilization after the periodic weighting on the low-level key strata and the moment magnitude did not significantly decrease due to the short duration.
Ground pressure of middle-level key stratum occurred and moment magnitude of MS events was uniformly distributed in the range between −1.3 and −0.2 from October 24 to October 26. This is attributed to the fact that MS events with large moment magnitude were produced by hard and thick stratum fracture and then there were MS events with small moment magnitude released by the compaction of rotation and subsidence of the middle-level key strata on the damaged strata below. From October 27 to October 31, there were little MS events with the moment magnitude greater than −0.5 and most events with small moment magnitude. This period is roof re-stablization period after the ground pressure of middle-level stratum.
Hence, the moment magnitudes of MS events range from −1.3 to −0.2 in a cycle of roof activity. The maximum moment magnitude mostly was less than −0.5 during the period of non-pressure. The maximum moment magnitude distributed in the range of −0.5 to −0.2 can be viewed as the precursor of strata pressure behaviour. There were less caving strata below and the moment magnitude of most MS events is −0.9 to −0.2 subjected to mine pressure of the low-level key strata. By contrast, there were more caving strata below and the moment magnitude of MS events was distributed between −1.3 and −0.2 subjected to mine pressure of the middle-level key strata. In addition, a large amount of MS event with small moment magnitude occurred.
To further describe the magnitude distribution of MS events, b value was calculated, as shown in . The b value decreased from the initial 1.285 to 0.694 and then to 0.261 as the periodic weighting occurred. The proportion of large energy events increased under the ground pressure of roof key strata, especially the middle-level key stratum. The b value increased to 1.209 after the ground pressure on roof strata. According to the variation of b value in a cycle of roof activity, it was found that the b-values of MS events before and after ground pressure were distributed between 1.2 and 1.5. During the periods, MS events were mainly generated by the stress redistribution of rock strata induced by mining activity. By contrast, the b value subjected to the ground pressure was below 0.8, which is mainly due to MS events caused by the slippage during the rotation and subsidence of the key rock block after fracturing.
4. Temporal and spatial evolution of MS events in roof key strata
4.1. Temporal and spatial evolution of MS events along the dip direction
Projection distribution of MS events along the dip direction during an entire roof active cycle (October 15, 2015 to October 31, 2015) is shown in . The variation law of MS parameters reflects the fracture and movement characteristics of roof strata.
Figure 5. Distribution of MS events released by roof key strata fracture along the dip direction: (a) from October 15 to 21 in 2015; (a) from October 15 to 31 in 2015.
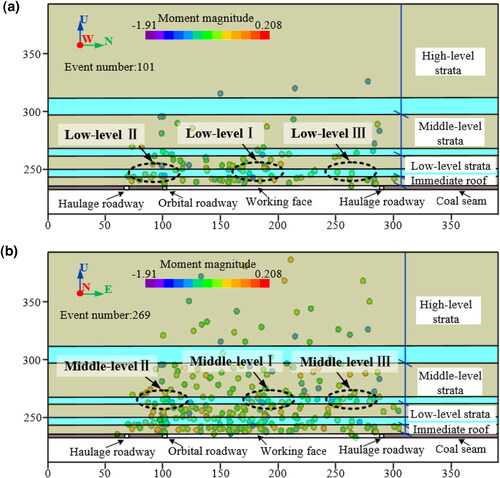
As shown in , during the period from October 15 to 21, 2015, stress concentration gradually formed in the roof as the coal seam was extracted. Micro cracks initiated inside roof stratum and outward radiating seismic waves were captured by MS sensors. In total, there were 101 events released, mostly distributed in the immediate roof and low-level stratum. Meanwhile, the MS event clusters were determined according to the degree and quantity of aggregation. There were three concentrations of MS events, named low-level I, II and III, along the dip direction. This indicates that a large number of micro fractures were produced and macroscopic fracture occurred in the middle and two edges of the low-level key strata.
According to , there were 168 events in total during the period of October 22 to 31, most of which were distributed in the middle-level and low-level strata and immediate roof. The maximum vertical height of crack propagation increased to the top interface of high-level strata. There were three concentrations in MS event distribution, called middle-level I, II and III, along the dip direction. The location of middle-level concentrations corresponds to those of low-level key strata along the dip direction. There were numerous micro fractures occurred in the middle and two edges of the middle-level key stratum along the dip direction and macroscopic rupture occurred in the middle-level key stratum, namely, periodical weighting appeared in the middle-level roof key stratum. As rock blocks of the low-level key layer rotated and sunk, a large number of micro fractures occurred in the roof, which results in the weakening in the bearing capacity of strata below the middle-level key stratum. The micro fractures in the middle-level key stratum gradually gathered and then macroscopic rupture occurred. Furthermore, numerous MS events occurred due to the dislocation and slip between the broken rock blocks.
4.2. Temporal and spatial evolution of MS events along the strike direction
shows projection distribution of MS events along the strike direction during a complete roof active cycle. As shown in , two MS event clusters, named low-level A and B, were formed in “pairs” before and behind the working face due to the fracture of low-level key stratum. The MS events between the clusters are relatively dispersed. The blocks after fracturing still have good integrity and form the key block that support each other, thus forming a supporting effect on the upper overburden rock. Moreover, the clusters were distributed in a zonal pattern and extend upward, indicating the angle of the fracture line in the strike direction of the roof strata. It can be found from that MS event clusters of low-level A and B then expanded upward and formed MS event clusters of middle-level A and B within the middle-level stratum. The concentration zone of MS events was formed through the low-level, middle-level strata and immediate roof. This is due to the fact the coal seam was further extracted and the macro fracture of roof extended upward to the middle-level strata.
Figure 6. Distribution of MS events released by roof key strata fracture along the strike direction: (a) from October 15 to 21 in 2015; (a) from October 15 to 31 in 2015.
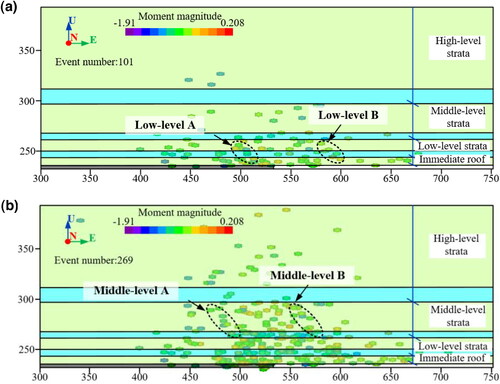
MS event clusters appeared in pairs along the strike direction during the fracture of low-level and middle-level strata. Pairs of clusters were located on the fracture line and its extension line, i.e., the two edges of key strata fracture. MS events were produced by micro crack initiation and expansion during the rotation and subsidence process of fractured key blocks and soft stratum.
5. Fracture mechanism of key strata based on moment tensor inversion
5.1. Principle of moment tensor inversion
For a point source, the solution of the Navier equation in elastodynamics on the inner surface of an object can be expressed as (Aki and Richards Citation2002; Zhao et al. Citation2019):
(1)
(1)
where u refers to the displacement at time t, the symbols *,
represent the convolution operation, moment tensor, spatial derivative of Green’s function and the time function of source, respectively.
In the Cartesian coordinate system, the moment tensor M can be expressed as:
(2)
(2)
To distinguish the fracture type of seismic source, the moment tensor (M) is usually decomposed into three parts, including isotropic component (MISO), double couple component (MDC) and compensated linear vector dipole component (MCLVD) (Knopoff and Randall Citation1970). The ISO component the volume change of the source area and the DC component represents the shear displacement of the source area without volume change, as shown in . The CLVD component characterizes the deformation except the shear deformation without the volumetric strain. The proportion of double couple component (MDC), which represents shear faulting, is determined by the following formula (Graham et al. Citation2010):
(3)
(3)
Figure 7. Schematic diagram of moment tensor decomposition (Graham et al. Citation2010).
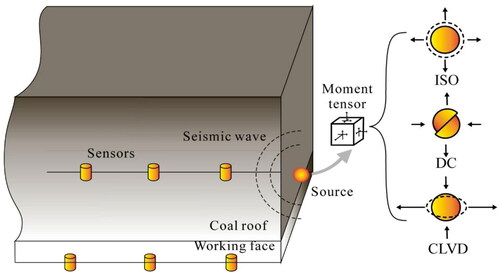
Obviously, PDC ranges from 0 to 1. There are two ultimate states for source mechanism: (1) a purely shear source when PDC is equal to 1; (2) an entirely tensile source when PDC is equal to 0. Fracture type can be determined according to the following definition proposed by Ohtsu and Uddin (Citation2008). The fracture type of the source is classified into shear fracture when PDC≥60%, whereas the fracture type is classified into tensile fracture when PDC≤40%. Other cases, i.e., 40% < PDC < 60%, belong to the mixed fracture.
In addition to the fracture properties, the geometry of fracturing at the source can be calculated by the moment tensor inversion, as shown in . The normal (n) and slip vector (l) of fracture surface at the source can be calculated by the eigenvalues (M1, M2, M3) and corresponding eigenvectors (e1, e2, e3) of the moment tensor (M) (Vavryčuk Citation2005; Zhao et al. Citation2020):
(4)
(4)
The geometric parameters of the fracture surface can be further determined by the normal (n) and slip vector (l):
(5)
(5)
(6)
(6)
where the symbols
and
refer to the strike, dip angle and slip angle of the fracture surface, respectively, which can represent the azimuth information of fracture.
5.2. Fracture mechanism of key strata
According to the analysis results in Section 4, there are concentration zones of MS events in low-level and middle-level key strata during the fracture process. The concentration zones can be inferred as the potential fracture zones of key strata. Evolution law of fracture types during the movement process of key strata can be determined using moment tensor inversion method. The fracture pattern and location of key stratum were obtained based on geometric information of micro fractures, which facilitates the accurate calculation of the fracture distance and pattern of the key strata in the next period. Then sliding direction of key strata after fracturing were real-time acquired. To explore micro failure mechanism during the fracture process of key strata, MS events distributed in middle-level I and II concentration zones along the dip direction and middle-level A and B concentration zones along the strike direction in the middle-level key strata were investigated.
The waveform data of MS events were imported into Matlab software and then the polarity of initial pulse and P-wave displacement were obtained by picking up the P-wave amplitude of waveforms. The moment tensor corresponding to the seismic source was determined and decomposed. The double couple component (PDC) of MS Event 1 is 0.22%, so the fracture type of Event 1 is tensile type. The spatial parameters of micro fracture surface were calculated by EquationEqs. 5(5)
(5) and Equation6
(6)
(6) . The strike, dip angle and slip angles for Event 1 are 285.52, 82.14 and −102.78, respectively. Similarly, fracture type and geometric parameters of micro fractures in the middle-level key strata are shown in and . Note that the period “after ground pressure” in and refers to the period after ground pressure appeared, including the periods under and after ground pressure.
Table 3. Fracture type and spatial parameters of MS events along the dip direction.
Table 4. Fracture type and spatial parameters of MS events along the strike direction.
5.2.1. Fracture mechanism of key strata along the dip direction
As shown in , 19 MS events, which are distributed in middle-level I and II concentration zones, were analyzed based on moment tensor method. shows MS event distribution with different fracture mechanism along the dip direction. Note that red, blue and green disks refer to tensile, mixed and shear events, respectively. The azimuth of disks represents the orientation of the fracture surface. It can be found that there are 8 tensile, 8 mixed and 3 shear events. Fracture types were analyzed during different stages of key strata behaviours. The exposed area of the roof above the goaf increased gradually with mining before the ground pressure. Bending tensile fractures occurred to release the accumulated energy. Thus, MS events during this period were mainly tensile and mixed types. Fractured rock formed the “masonry beam” structure of arch subjected to horizontal load, accompanied by rotation and subsidence in the key rock blocks with the mining activity. When the horizontal load between the broken key blocks was insufficient to support the “masonry beam” structure, the roof strata slipped and dynamic instability occurred. Subsequently, a shear zone appeared along the fracture surface at both ends of key blocks, which results in large scale shear failure or slip instability of broken blocks and soft stratum. The shear component (PDC) of MS events in the middle and edge of the goaf began to increase after ground pressure appeared, which indicates mixed and shear cracks occurred.
The average value of micro-crack azimuth is used to estimate the macro fracture angle of key strata. According to the relationship between micro-crack strike and due east direction, MS events distributed in the middle-level I concentration zone above the middle of the working face can be divided into two groups. In the first group, the angle between events 1, 2, 6, 8, 9, 10, 13, 14 and 15 and due east direction is less than 45°. By contrast, in the second group, the angle between events 3, 4, 5, 7, 11 and 12 and due east direction is greater than 45°. It can be found that MS events are distributed near two groups of fracture surfaces. In the first group of events, except for events 2, 6 and 10, the fracture surface strike of other events is close to each other. The angle between the fracture surface of the key stratum and the due north is approximately 290°, which indicates the fracture surface extends eastward. There is no obvious trend in the dip angle of the fracture surface. For the second group events, the strike of facture surface is within 25° on both sides of due north, which implies that the fracture surface extends northward. The dip angle of fracture surface is approximately 76° on average.
Through the analysis of Event 16–19 distributed in the middle-level II concentration zone, the broken section of the key stratum at the edge of the working face has an average angle of 85° with the horizontal plane, and obliquely extends outwards at an angle of 46° with the north direction except for Event 17. The broken key block slides and sinks to the rear goaf with an angle of 6° from the vertical direction.
It can be found that the fracture of the middle-level key stratum basically conforms to the “O-X” fracture pattern, as shown in . The strike of the first group of fractures (labeled ① in ) is within 25° on both sides of the dip of the working face, which indicates the fracture surfaces are O-type fractures. The X-type fractures (labeled ② in ) extend outward with an angle of 46° from the dip of the working face. The angle between fracture surface and the horizontal plane is averagely 85°. Furthermore, in terms of the relationship between the strike of micro-fracture surface and the rupture type, the strike of micro-tensile fracture surface mostly extends to the east; that is, it is the same as the advancing direction of working face. This is because the length of working face is too larger than the fracture distance of key stratum along the strike direction and tensile fracture is more likely to occur during the bending and subsidence process.
5.2.2. Fracture mechanism of key strata along the strike direction
A total of 13 MS events distributed in the middle-level A and B concentration zones were analyzed based on moment tensor inversion. Fracture type and spatial parameters of MS events along the strike direction are shown in . shows MS event distribution with different fracture mechanism along the strike direction. According to crack type classification, there are 3 tensile, 6 mixed and 4 shear events. Middle-level A concentration zone of MS events is in front of the working face and micro fractures in this zone are tensile and shear types. The occurrence of tensile cracks is attributed to the fact that the bending moment of rock beam is large and local strain energy is released by bending deformation. In addition, Guo et al. (Citation2003) stated a high compressive stress zone is formed in front of the coal seam due to mining activities and two high shear stress zones are distributed above and below the working face. It is easy to cause sudden rupture of coal and rock mass along the direction of maximum dominant shear stress, which explains the occurrence of shear fractures in the middle-A zone. By contrast, middle-level B concentration zone of MS events is above the goaf behind the working face and micro-crack types are mainly mixed and partial shear. This is because the rock strata in this zone was broken during the last key strata breaking process and formed a hinge with the adjacent key block, and MS events occurred during the rotation of the hinge point.
The dip angle of fracture surface of key stratum along the strike direction is approximately the average of the dip angles of every micro fractures. For the middle-level A zone, i.e., middle-level key stratum in front of the working face, the angle between the fracture surface and the horizontal plane is 54° on average. The key block of fracture is the footwall of the fracture surface. For the middle-level B zone, i.e., middle-level key stratum behind the working face, the fracture surface can be classified into two groups according to the sliding trend. The first group consists of MS events no. 27, 29 and 32, whereas the second group is made up of MS events no. 26, 28, 30 and 31. The slip angle and dip angle for the first group are −95° and 81°, respectively. The first group MS events were generated during the rotation and subsidence of the hinge of the key blocks along the fracture surface. In contrast, the slip angle and dip angle for the second group are 88° and 53°, respectively. The second group of MS events are released by the rotation of the hinge point between the key block.
6. Discussion
Taking a typical working face in Dongjiahe Coal Mine as an example, the fracture pattern and process of roof key strata in a cycle of roof activity were investigated in this study based on microseismic monitoring. The increase of MS event number and release energy can be viewed as a precursor for the occurrence of periodic weighting. Compared to b values before and after ground pressure, b value of MS events subjected to the ground pressure is much smaller. This can be attributed to the release of large energy events under the ground pressure of roof key strata. MS events distributed in middle-level strata were used to study fracture mechanism of roof key strata. It is found that the fracture of the middle-level key stratum basically conforms to the “O-X” fracture pattern, which is of much significance for targeted support for the working face. Furthermore, it is of guiding significance to effectively prevent the appearance of ground pressure on the working face. The findings in this paper provide a theoretical reference for strata behaviours and hazard risk assessment under similar conditions.
This paper takes Dongjiahe coal mine as an example to study fracture mechanism of roof strata. More engineering cases are needed due to the difference in geological conditions between different engineerings. This paper focuses on fracture mechanism of roof key strata using microseismic moment tensor. It is worth to further investigate the evolution law of stress field in roof rock strata by numerical simulation analysis.
7. Conclusions
In this study, micro-seismic (MS) signals produced by rock fracture were located during the typical working face mining process in the Dongjiahe coal mine. The evolution process of MS parameters was analyzed during the periodic weighting process of the roof key stratum. Finally, fracture modes of key strata were investigated based on the moment tensor inversion method.
According to MS event distribution, there are three concentration zones of MS events located in the middle along the dip direction and above both sides of the mining roadway when the key strata were broken. During the fracture process of the middle-level and low-level key strata, MS concentration zones always appear in pairs along the strike direction and they are located on the fracture line and its extension line (i.e., at both ends of the fractured key block) formed after the ground pressure appears. The MS parameters varied during the fracture process of roof key strata. The moment magnitude of MS events is below −0.5 and the number of events is less than 15 per day during the period of non-pressure. In contrast, during the roof periodical pressurized period, the maximum moment magnitude of daily MS events is distributed between −0.5 and −0.2. The number of daily events is 19–25 for the low-level key strata and 25–32 for the middle-level key strata.
Taking the middle-level key strata as an example, fracture types were analyzed through moment tensor inversion. Along the strike direction, tensile and shear factures occur before the working face and the angle between the fracture dip angle and horizontal plane is 54°, while the micro-fractures are mainly mixed type and partial shear type behind the working face. By contrast, the rock fracture usually occurs behind the working face along the dip direction. The micro fractures are mainly tensile and mixed types before fracturing compared to mainly mixed and shear fractures with slight tensile ones during the subsidence process after fracturing. The fracture mode basically conforms to the “O-X” shape. The X-shaped fracture extends outward with an angle of 46° with the due north and the angle between the fracture surface and the horizontal plane is averagely 85°. Along the dip direction, the first group of fracture surfaces extend eastward with an angle of 290° with the due north, while the second group of fracture surfaces extend northward and their strike is within 25° on both sides of the due north.
Disclosure statement
No potential conflict of interest was reported by the authors.
Data availability statement
The data that support the findings of this study are available from the corresponding author, Zhenghu Zhang, upon reasonable request.
Additional information
Funding
References
- Aki K, Richards PG. 2002. Quantitative seismology, 2nd Ed. Sausalito: University Science Books.
- Baro O, Kumar A, Ismail-Zadeh A. 2018. Seismic hazard assessment of the Shillong Plateau, India. Geomat Nat Haz Risk. 9(1):841–861.
- Cai W, Dou L, Si G, Cao A, Gong S, Wang G, Yuan S. 2019. A new seismic-based strain energy methodology for coal burst forecasting in underground coal mines. Int J Rock Mech Min Sci. 123:104086.
- Cao W, Durucan S, Cai W, Shi JQ, Korre A, Jamnikar S, Rošer J, Lurka A, Siata R. 2020. The role of mining intensity and pre-existing fracture attributes on spatial, temporal and magnitude characteristics of microseismicity in longwall coal mining. Rock Mech Rock Eng. 53(9):4139–4162.
- Cao W, Shi JQ, Si G, Durucan S, Korre A. 2018. Numerical modelling of microseismicity associated with longwall coal mining. Int J Coal Geol. 193:30–45.
- Cheng G, Yang T, Liu H, Wei L, Zhao Y, Liu Y, Qian J. 2020. Characteristics of stratum movement induced by downward longwall mining activities in middle-distance multi-seam. Int J Rock Mech Min Sci. 136:104517.
- Dou L, Cai W, Cao A, Guo W. 2018. Comprehensive early warning of rock burst utilizing microseismic multi-parameter indices. Int J Min Sci Tech. 28(5):767–774.
- Gibowicz SJ, Kijko A. 1994. An introduction to mining seismology. New York: Academic Press.
- Gibowicz SJ, Young RP, Talebi S, Rawlence DJ. 1991. Source parameters of seismic events at the underground research laboratory in Manitoba, Canada: scaling relations for events with moment magnitude smaller than -2. B Seismol Soc Am. 81(4):1157–1182.
- Graham CC, Stanchits S, Main IG, Dresen G. 2010. Comparison of polarity and moment tensor inversion methods for source analysis of acoustic emission data. Int J Rock Mech Min Sci. 47(1):161–169.
- Guo R, Pan CL, Yu RC. 2003. Mining theory and technology of hard rock deposits with rockburst tendency. Beijing: Metallurgical Industry Press. (in Chinese)
- Guo WY, Qiu Y, Zhao TB, Tan YL, Lan S, Chen WG. 2019. Influence of the variable stoping speed on the occurrence mechanism of rock burst. Geomat Nat Haz Risk. 10(1):2094–2105.
- Guo W, Zhao G, Lou G, Wang S. 2019. Height of fractured zone inside overlying strata under high-intensity mining in china. Int J Min Sci Techno. 29(1):45–49.
- He H, Dou L, Cao A, Fan J. 2015. Mechanisms of mining seismicity under large scale exploitation with multikey strata. Shock Vib. 2015:1–9.
- Jian S. 2017. Mechanics criterion and factors affecting overburden stability in solid dense filling mining. Int J Min Sci Tech. 27(3):407–413.
- Jiang L, Wu Q, Wu Q, Wang P, Xue Y, Kong P, Gong B. 2019. Fracture failure analysis of hard and thick key layer and its dynamic response characteristics. Eng Fail Anal. 98:118–130.
- Kang H, Lou J, Gao F, Yang J, Li J. 2018. A physical and numerical investigation of sudden massive roof collapse during longwall coal retreat mining. Int J Coal Geol. 188:25–36.
- Knopoff L, Randall MJ. 1970. The compensated linear-vector dipole: A possible mechanism for deep earthquakes. J Geophys Res. 75(26):4957–4963.
- Li Y, Yang TH, Liu HL, Wang H, Hou XG, Zhang PH, Wang PT. 2016. Real-time microseismic monitoring and its characteristic analysis in working face with high-intensity mining. J Appl Geophys. 132:152–163.
- Lu Y, Gong T, Xia B, Yu B, Huang F. 2019. Target stratum determination of surface hydraulic fracturing for far-field hard roof control in underground extra-thick coal extraction: a case study. Rock Mech Rock Eng. 52(8):2725–2740.
- Ma K, Sun X, Tang C, Wang S, Yuan F, Peng Y, Liu K. 2020. An early warning method for water inrush in Dongjiahe coal mine based on microseismic moment tensor. J Cent South Univ. 27(10):3133–3148.
- Mondal D, Roy PNS, Kumar M. 2020. Monitoring the strata behavior in the destressed zone of a shallow Indian longwall panel with hard sandstone cover using Mine-Microseismicity and Borehole Televiewer data. Eng Geol. 271:105593.
- Mukhopadhyay B, Fnais M, Mukhopadhyay M, Dasgupta S. 2010. Seismic cluster analysis for the Burmese–Andaman and West Sunda Arc: insight into subduction kinematics and seismic potentiality. Geomat Nat Haz Risk. 1(4):283–314.
- Ohtsu M, Uddin FAKM. 2008. Mechanisms of corrosion-induced racks in concrete at meso- and macro-scales. ACT. 6(3):419–429.
- Qian MG. 1982. A study of the behavior of overlying strata in longwall mining and its application to strata control. In: Proceedings of the Symposium on Strata Mechanics. New York: Elsevier Scientific Publishing Company; p. 13–17.
- Qian M, Miao X, Xu J. 1996. Theoretical study of key stratum in ground control. J China Coal Soc. 21(3):225–230. (in Chinese)
- Vavryčuk V. 2005. Focal mechanisms in anisotropic media. Geophys J Int. 161(2):334–346.
- Wang Y, He M, Ren F, Zhu C, Faramarzi L. 2019. Source analysis of acoustic emissions during granite strain burst. Geomat Nat Haz Risk. 10(1):1542–1562.
- Wang F, Xu J, Xie J. 2019. Effects of arch structure in unconsolidated layers on fracture and failure of overlying strata. Int J Rock Mech Min Sci. 114:141–152.
- Wu Q, Jiang J, Wu Q, Xue Y, Kong P, Gong B. 2018. Study on the fracture of hard and thick sandstone and the distribution characteristics of microseismic activity. Geotech Geol Eng. 36(6):3357–3373.
- Xie J, Xu J. 2017. Effect of key stratum on the mining abutment pressure of a coal seam. Geosci J. 21(2):267–276.
- Xu NW, Tang CA, Li LC, Zhou Z, Sha C, Liang ZZ, Yang JY. 2011. Microseismic monitoring and stability analysis of the left bank slope in Jinping first stage hydropower station in southwestern China. Int J Rock Mech Min Sci. 48(6):950–963.
- Xu T, Yang TH, Chen CF, Liu HL, Yu QL. 2015. Mining induced strata movement and roof behavior in underground coal mine. Geomech Geophys Geo-Energ Geo-Resour. 1(3-4):79–89.
- Yu B, Zhao J, Xiao H. 2017. Case study on overburden fracturing during longwall top coal caving using microseismic monitoring. Rock Mech Rock Eng. 50(2):507–511.
- Yuan F, Ma K, Zhuang D, Wang Z, Sun X. 2019. Preparation mechanism of water inrush channels in bottom floor of Dongjiahe coal mine based on microseismic monitoring. J China Coal Soc. 44(6):1846–1856. (in Chinese)
- Zhang W, Qu X, Li C, Xu X, Zhang S, Jin G, Wang Y. 2019. Fracture analysis of multi-hard roofs based on microseismic monitoring and control techniques for induced rock burst: a case study. Arab J Geosci. 12(24):784.
- Zhang PH, Yang TH, Yu QL, Xu T, Shi WH, Li SC. 2016. Study of a seepage channel formation using the combination of microseismic monitoring technique and numerical method in Zhangmatun iron mine. Rock Mech Rock Eng. 49(9):3699–3708.
- Zhao Y, Yang T, Zhang P, Xu H, Wang S. 2020. Inversion of seepage channels based on mining-induced microseismic data. Int J Rock Mech Min Sci. 126:104180.
- Zhao Y, Yang T, Zhang P, Xu H, Zhou J, Yu Q. 2019. Method for generating a discrete fracture network from microseismic data and its application in analyzing the permeability of rock masses: a case study. Rock Mech Rock Eng. 52(9):3133–3155.
- Zhou JR, Yang TH, Zhang PH, Xu T, Wei J. 2017. Formation process and mechanism of seepage channels around grout curtain from microseismic monitoring: a case study of Zhangmatun iron mine, China. Eng Geol. 226:301–315.