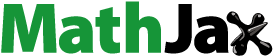
Abstract
The selection of structural strength indicators is of utmost importance for slope engineering safety. This paper, with the backdrop of the destruction of high liquid limit clay layers in the Huai River slope, aims to investigate the influence of dry-wet (D-W) cycles on the structural and mechanical properties of undisturbed high liquid limit clay. Through unconfined compression tests, scanning electron microscopy (SEM) tests, and triaxial shear tests, the structural behavior, stress-strain curves, porewater pressure-strain curves, and effective stress paths of undisturbed samples taken at three different angles and reconstituted samples were analyzed under the condition of maximum drying stress with 0 and 1 D-W cycle. Based on the impact of D-W cycles on the effective stress path, the shear failure mode of structurally high liquid limit clay under the influence of D-W cycles was identified. A method for evaluating the anisotropic level of structural clay after experiencing D-W cycles was proposed. The test results show that compared with reconstructed soil, the undisturbed high liquid limit clay with structure is more significantly affected by the D-W cycle. After D-W cycles, the CU shear strength of high liquid limit clay increased significantly. The failure mode transitioned from a hardening-shear dilation mode to a softening-partial shear contraction-partial shear dilation mode. The appearance of the phase transition state (PTS) point may be attributed to the partial action of effective stress on cracks inside the sample, resulting in shear contraction. D-W cycles weakened the structural properties (anisotropy) of high liquid limit clay.
1. Introduction
High liquid limit clay contains a significant amount of hydrophilic minerals and is widely distributed in the river and lake deposit areas. In its natural state, it typically exhibits high moisture content, poor permeability, and a tendency to crack when drying. External climate changes can lead to drastic fluctuations in soil moisture content, subsequently causing deformation in soil structure and alterations in mechanical properties. Therefore, the ‘Technical Specifications for Highway Subgrade Construction’ (JTGF10 Citation2006) (China) stipulates that high liquid limit clay must undergo modification and meet specific standards before it can be used in engineering projects (Ma et al. Citation2022). However, in practical construction, the use of high liquid limit clay remains difficult to avoid. After heavy rain, the slopes in the Bengbu area of the main stream of the Huai River collapsed and were damaged. On-site investigation revealed a natural deposit of high liquid limit clay layer with noticeable vertical cracks, as shown in . According to the survey, prior to the damage, the surface miscellaneous fill soil of the slope in the Bengbu section was cleared due to the construction of new projects, exposing the high liquid limit clay. During the period from May to October, the Bengbu region experienced continuous high temperatures and drought conditions. After a heavy rain, the slope suffered damage. Therefore, immediate and in-depth experiments are being conducted to reveal the reasons for the damage and determine effective remediation measures, which is of great practical significance.
Figure 1. The destruction site of the bengbu section of the mainstream of the Huaihe River (a) China map; (b) bengbu slope collapse behavior; (c) the destruction site.
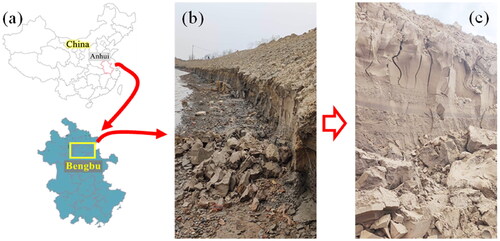
Worldwide, many scholars have reported on engineering events related to high liquid limit clay. Jiang and Cui (Citation2022) conducted a study on the stability of high liquid limit clay slopes induced by rainfall. Through field monitoring and numerical simulations, they investigated the impact of different rainfall intensities and durations on slopes composed of high liquid limit clay. Zhang et al. (Citation2021) analyzed the mechanisms of landslides in a village in Liuzhou, Guangxi, China, and pointed out that high liquid limit clay, affected by wetting and drying cycles, develops significant cracks, leading to rapid rainwater infiltration and the formation of a sliding surface at the soil-rock interface. Cai et al. (Citation2019) conducted centrifuge model experiments to study the stability of channel slopes composed of high liquid limit clay in northern Xinjiang, China. Their analysis indicated that D-W cycles associated with water flow and cessation of water flow caused shallow landslides, distinguishing them from traction-induced landslides. These examples illustrate the significant impact of D-W cycles on high liquid limit clay, emphasizing its importance in engineering contexts. Many scholars have analyzed the effects of factors like dry density, initial moisture content, and cycle number on the shear strength parameters, initial tangent modulus, and crack evolution patterns of soil through UU tests and direct shear tests (Lü et al. Citation2009; Deng et al. Citation2017; Yuan et al. Citation2017; Liu et al. Citation2021). These studies provide a detailed discussion of the mechanisms involved in the deterioration of clay due to D-W cycles. However, Tu et al. (Citation2017) pointed out that actual soil may experience certain gravitational or overburden load effects after D-W cycling. Therefore, UU tests may not fully reflect objective patterns. Some scholars, in experiments considering the simulated impact of D-W cycles on slope stability, have conducted CU or CD tests. Xiao et al. (Citation2013) proposed suggestions for selecting soil strength parameters in the analysis of shallow slope failure based on CU test results. Mu et al. (Citation2016) conducted CD tests on red clay with varying D-W cycle numbers and moisture content, demonstrating the hazards of red clay slopes. Tu et al. (Citation2017) discussed the influence of experimental methods on simulated slope test results and suggested that crack development is a cause of soil strength deterioration. Yang et al. (Citation2018) summarized the strength decay patterns of undisturbed soil based on CU test results with three different soils and recommended the use of a double-linear function for representation. Scholars have made significant contributions to understanding the long-term mechanical properties of soil concerning D-W cycle numbers. However, Liu et al. (Citation2017) pointed out that, at low cycle numbers, the magnitude of the drying stress history has a more significant impact on soil mechanical properties than the number of D-W cycles. Liu et al. (Citation2017) conducted a comparative study of the influence of drying stress history on soil mechanical properties for zero and one D-W cycle, comprehensively discussing the effect of matrix suction in unsaturated soil on the mechanical properties of saturated soil. The above studies provide important reference points for the destruction of the Huai River slope. However, from the perspective of the slope failure form in the Huai River, distinct from typical landslides, it appears to be a collapse failure where horizontal strength has seemingly been lost, closely related to anisotropic strength. The selection of structural strength indicators is crucial for the safety of slope engineering (Li et al. Citation2014; Citation2016). For instance, in the sliding failure of a slope or foundation, the actual stress states at different positions are different. Relying solely on parameters in a single vertical direction may deviate from the actual situation, posing significant risks to engineering construction (Liang et al. Citation2012). Therefore, adopting rational methods and approaches to reveal the mechanical properties of undisturbed natural soils is of utmost importance (Yao et al. Citation2012; Huang et al. Citation2016). Clay from natural deposits exhibits distinct structural characteristics (Bradford Citation1981; Zhang et al. Citation2010; Zhu et al. Citation2015). Existing literature has extensively reported on structural studies, including the research on quantitative parameters for structural strength (Xie and Qi Citation1999), the establishment of structural constitutive models (Wang and Shen Citation2007; Yao et al. Citation2019), microcharacterization of structure (Li et al. Citation2022; Liang et al. Citation2023), and mechanical properties (Newson et al. Citation2006; Ng et al. Citation2017). A few scholars have also developed constitutive models for the structural effects of D-W cycles: Shao et al. (Citation2006) described the damage characteristics of Q3 loess under D-W cycles based on damage mechanics theory; Sun et al. (Citation2010) established an elastoplastic model considering the influence of saturation on unsaturated soil. Hao et al. (Citation2021) introduced structural parameter transformation into the Duncan-Zhang model, establishing a structural constitutive model that considers the impact of D-W cycles. However, there is currently limited research on the effects of dry-wet cycles on the structural and mechanical properties of structured clays.
In summary, at present, The existing literature has reported in detail the impact of the number of D-W cycles on soil strength parameters, crack development, deterioration mechanisms, test types and constitutive relationships, which provides an important reference value for the damage of the Huaihe River slope. However, starting from practical engineering problems, high liquid limit clay is different from ordinary soil because the large amount of hydrophilic minerals in high liquid limit clay makes it special. External climate changes can easily cause drastic changes in the moisture content of the soil, causing changes in the structure and mechanical properties of the soil. At the same time, the influence of D-W cycles on the anisotropic strength of high liquid limit clay is a factor that cannot be ignored in the failure of Huaihe River slopes, but there are few reports in the existing literature. Therefore, it is necessary to study the mechanical properties of structural high liquid limit clay under the influence of D-W cycles. This paper primarily presents two-part research through triaxial consolidated undrained tests: The first part of the study focuses on the structural and mechanical properties of high liquid limit clay, starting from microstructure, the stress-strain curve, porewater pressure-strain curve, and shear strength of high liquid limit clay. The second part investigates the mechanical properties of high liquid limit clay after 1 cycle of D-W cycle. It places special emphasis on discussing the impact of D-W cycles on the failure mode and structural nature of high liquid limit clay. The experimental conclusions provide important references for the safety and stability of high liquid limit clay in slope engineering and hold practical research significance.
2. Materials and methods
2.1. Materials
The undisturbed soil was collected from the Bengbu section of the Huaihe River Basin (location: longitude 117.38, latitude 32.95) at a depth of 2-3 meters. The soil is formed from the deposition of river sediments and exhibits a stratified structure. Laboratory tests conducted on the retrieved soil samples indicate that the liquid limit of this soil is 53.5%, and the plasticity index is 27.1. According to AASHTO (Citation1991) established by the American Society of Civil Engineers and the American National Standards Institute, this soil is classified as high liquid limit clay. The particle size distribution of tested clay and the basic physical properties of the tested clay are shown in and . The clay mineral composition was analyzed using X-ray diffraction (XRD), and the mineral composition is shown in .
Table 1. Basic physical properties of the tested clay.
Table 2. Mineral composition of high liquid limit clay.
2.2. Experimental methods
2.2.1. Experimental program
To study the mechanical properties of structural high liquid limit clay before and after the influence of D-W cycles, relevant experiments were designed, which can be primarily divided into two parts:
To investigate the impact of the structural nature of high liquid limit clay on its mechanical properties. Firstly, unconfined compression tests and scanning electron microscopy (SEM) tests were conducted to assess the structural characteristics of undisturbed samples. Secondly, reconstituted samples and three differently oriented undisturbed samples, sampled at angles θ = 0°, 45°, and 90° as shown in , were subjected to consolidated undrained (CU) shear tests to study the effect of structural characteristics on the mechanical properties of high liquid limit clay. The triaxial compression test of high liquid limit clay is shown in .
To investigate the influence of D-W cycles on the mechanical properties of structural high liquid limit clay. CU shear tests were conducted on reconstituted samples and undisturbed samples obtained at three different angles (θ = 0°, 45°, and 90°) after 1 cycle of D-W conditions. The sampling angles are shown in , and the triaxial compression test of high liquid limit clay with 1 D-W cycle is shown in .
Table 3. Triaxial compression test of high liquid limit clay.
Table 4. Triaxial compression test of high liquid limit clay with 1 D-W cycle.
This paper focuses on the impact of the maximum drying stress history on the mechanical properties of the soil, rather than the number of D-W cycles (the maximum drying stress history refers to the complete dehydration of the soil). Liu et al. (Citation2017) pointed out that the greater the drying stress history, the more pronounced the changes in the mechanical properties of the soil. Before the damage occurred in the slope section of Bengbu, the Bengbu area experienced prolonged periods of high-temperature drought, followed by destruction after heavy rainfall. Therefore, experimental research was conducted on samples with a low number of cycles under the maximum drying stress history.
2.2.2. Unconfined compression tests and the triaxial shear tests
Unconfined compression tests: The unconfined compression test on a natural strong structured clay were conducted according to the China National Standards GB/T 50123-2019 (Standardization Administration of China Citation2019).
The triaxial shear tests: In the triaxial shear tests, all samples were fully saturated. The saturation process includes a 1-h vacuum followed by 24 h of immersion. The shear testing equipment employed in the experiment is the TSZ-2 automated triaxial device, as shown in . Install the samples into the triaxial devices according to China National Standards GB/T 50123-2019 (Standardization Administration of China Citation2019) and apply cell pressure according to the predetermined plan. By monitoring Skempton’s pore-pressure parameter ‘B’ value, if it falls within the range of 0.97 to 1.0, it indicates that the sample is essentially saturated, and the devices automatically enters the consolidation phase. If the B value does not meet the saturation criteria, the triaxial devices will automatically apply cell pressure and back pressure (in increments of 20 kPa) until the B value under saturated conditions is reached. This process is aimed at ensuring that the soil sample remains adequately saturated throughout the testing procedure. When the consolidation degree exceeds 95%, consolidation is considered complete, and the system transitions to the shear phase. Throughout the shearing procedure, the equipment maintains a shearing rate of 0.02 mm/min, with shearing being terminated either upon reaching an axial strain of 15% or upon sample failure.
2.2.3. SEM tests
The samples need to have good conductivity, requiring them to be completely dry. Prior to loading, the samples should undergo air-drying treatment. Use appropriate tools to cut the samples and place them into the sample tray, as shown in . To ensure accuracy in observation, the plane to be observed on the sample should not have any secondary damage during the sampling and loading process. To ensure clear observation results, apply sputtering treatment to the samples. The sputtering instrument used is the SBC-12 ion sputtering instrument, as shown in . Open the sample chamber, as shown in . place the sample into the sample chamber, and tighten the screws to secure the sample tray. The instrument used for SEM analysis is the KYKY-EM6200 model, as shown in . Start the instrument, and set the scanning voltage to 15 kV, and the filament current to 2.34 A. Adjust relevant parameters such as contrast, brightness, and objective magnification to obtain the target image, and proceed with image capture.
2.3. Sample preparation
Preparation of undisturbed samples: To ensure the structural nature of undisturbed samples, a Shelby tube method was used to collect samples from the undisturbed soil. The tube walls were coated with vaseline, and samples were collected vertically. The collected soil was wrapped in plastic wrap to prevent moisture loss, and efforts were made to minimize disturbance during transportation. The collected soil was subjected to initial dry density and moisture content measurements, with an initial dry density of 1.39 g/cm3 and an initial moisture content of 31.2%. During sample preparation, samples were cut at three different angles, with an initial angle of 0° in the vertical direction, as shown in . Samples with a diameter of 39.10 mm and a height of 80 mm were prepared using a sample trimmer, as shown in . A mass-based approach was employed to exclude samples that were either too heavy or too light to ensure the consistency of undisturbed samples. The acceptable sample mass should be (175.1 ± 2) g.
Figure 6. Sample preparation equipment (a) sample trimmer; (b) dry oven; (c) soil sieve; (d) electronic scale; (e) mold and tamper; (f) saturation device.
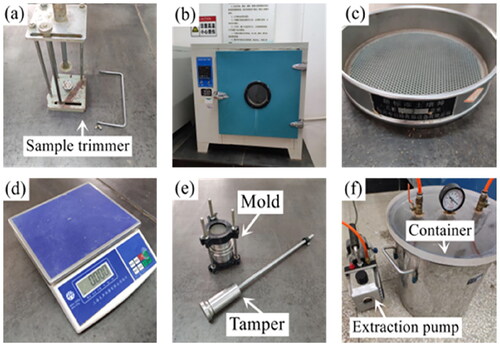
Preparation of reconstituted samples: The high liquid limit clay collected from the field was placed in a dry oven to dry and then crushed. It was sieved through a 2 mm sieve for later use. Using an electronic scale (accurate to 0.01 g), the sieved soil and water were mixed in the appropriate proportions, thoroughly stirred, and sealed for 24 h until the soil and water were uniformly mixed. The soil was subsequently portioned by mass into three equal segments. These portions were sequentially added to the mold in three distinct layers, each compacted by tamping and trimmed. Demolding the sample after it has been formed. The sample preparation equipment is shown in .
D-W cycles method: soil exhibits stress-path dependency, meaning that the extent of moisture loss, the rate of moisture loss or gain, and the number of cycles all exert an influence on the soil’s response. In practical terms, prior to commencing D-W cycle tests, it is necessary to consider parameters such as the upper limit of wetting, lower limit of drying, cycle rate (rate of desaturation and resaturation), and the number of cycles. These specific parameters should be set based on the actual conditions. The upper limit of wetting was determined based on the saturated moisture content of the high liquid limit clay, assuming that the soil reaches a saturated state after rainfall or flooding. The lower limit of desaturation was set to the moisture content of oven-dried soil samples, which is 2%. The desaturation process was controlled by the desaturation rate. For soil samples with the same dry density and moisture content and when temperature is the only external variable, the temperature will determine the rate of desaturation. The experiment used a dry oven with a temperature set at 30 °C. The samples were removed from the oven at 1-h intervals, and their masses were measured and used to calculate the moisture content. When approaching the target moisture content, the samples were weighed every 5 min until the target moisture content was reached. The wetting process used the vacuum saturation method to wet the samples to their saturated moisture content. 1 cycle was completed after 1 cycle of desaturation and wetting.
3. Results and analysis
3.1. The structural behavior of high liquid limit clay
Sensitivity is an indicator for assessing the structural nature of undisturbed soil. It is defined as the ratio of the unconfined compression strength of an undisturbed sample to that of its reconstituted sample and is calculated using the following formula:
(1)
(1)
where qu is the unconfined compression strength of the undisturbed soil, and qr is the unconfined compression strength of the reconstituted soil.
shows the unconfined compression strength curve of the high liquid limit clay. Calculated using EquationEquation (1)(1)
(1) , the sensitivity of the high liquid limit clay is determined to be 2.60. According to the classification by AASHTO (Citation1991), liquid limit clay is categorized as medium sensitivity cohesive soil. In other words, Huai River’s natural liquid limit clay exhibits structural characteristics.
shows 10kx electron scanning images of undisturbed and reconstituted samples of high liquid limit clay. To further confirm the structural nature of undisturbed high liquid limit clay, electron scanning was conducted on both undisturbed and reconstituted samples.
Figure 8. 10.0kx SEM images of undisturbed and reconstituted samples of high liquid limit clay (a) undisturbed sample θ = 0°; (b) undisturbed sample θ = 45°; (c) undisturbed sample θ = 90°; (d) reconstituted sample.
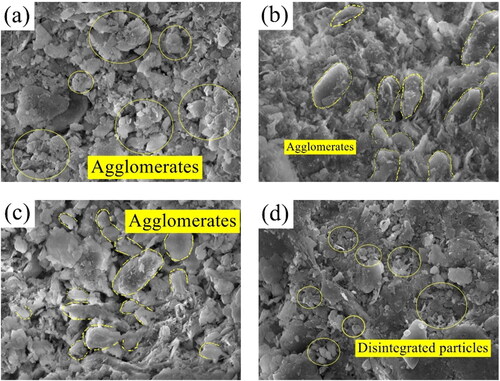
As shown in , undisturbed samples exhibit a distinct agglomerate structure, indicating the presence of colloid materials and well-connected particle connections. In contrast, shows the reconstituted samples, where the distinctive breakage of soil structure characteristics leads to noticeable fragmentation of reconstituted samples and loose particle connections. This finding is consistent with the observations of Li et al. (Citation2022) in their study of undisturbed and reconstituted clay in the Zhanjiang region. Additionally, during the sedimentation process, soil is directionally distributed in layers, with isotropy within the horizontal plane and anisotropy in other directions (Li et al. Citation2010; Gao et al. Citation2020). Comparing the microscopic images of undisturbed samples at different angles reveals that the undisturbed sample at θ = 0° exhibits clear isotropic characteristics, while the soil particles at the other two angles show anisotropy, indicating the anisotropic of high liquid limit clay.
3.2. The influence of D-W cycles on the stress-strain curves of structural high liquid limit clay
3.2.1. Stress-strain curve of structural high liquid limit clay with 0 D-W cycles
shows the stress-strain curves and porewater pressure-strain curves for the reconstituted and undisturbed samples (θ = 0°) of high liquid limit clay with 0 D-W cycles. The stress-strain curve of the undisturbed sample exhibits variations from that of the reconstituted sample due to the influence of its inherent structure. As shown in , both the reconstituted and undisturbed samples exhibit hardening-type stress-strain curves. However, when subjected to cell pressures of 50 kPa and 100 kPa, the stress peak in undisturbed samples is notably greater than that in reconstituted samples, with the undisturbed samples exhibiting an approximately 15 kPa and 50 kPa higher stress peak, respectively, compared to the reconstituted samples. This difference in stress peak is primarily caused by its structural nature. After undergoing long-term natural consolidation, the undisturbed sample acquires a structural nature, and when the cell pressure is lower than the structural yield stress (Wang et al. Citation2004), the structural nature enhances the strength of the soil.
Figure 9. Stress-strain and porewater pressure-strain curves of reconstituted and undisturbed samples (θ = 0°) of high liquid limit clay with 0 D-W cycles (a)reconstituted samples stress-strain curves; (b) reconstituted samples porewater pressure-strain curves; (c) undisturbed samples stress-strain curves; (d) undisturbed samples porewater pressure-strain curves.
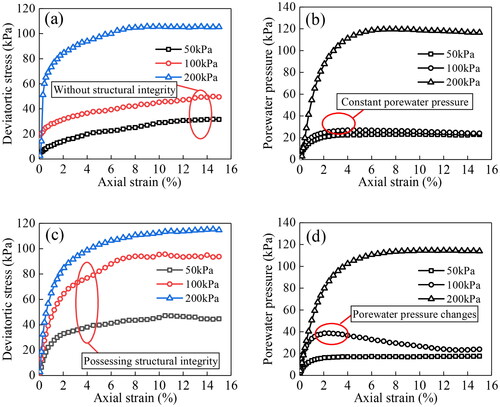
In terms of porewater pressure, due to the influence of its structure, the porewater pressure-strain curve of the undisturbed sample is not entirely consistent with that of the reconstituted sample. under cell pressures of 50 kPa and 100 kPa, the undisturbed sample shows an initial increase followed by a decrease in porewater pressure, as shown in . This indicates that shear dilation occurs during the shearing process. This primarily occurs because the undisturbed sample possesses structural characteristics, making it more susceptible to shear dilation compared to the reconstituted sample, resulting in an initial increase and subsequent decrease in porewater pressure.
shows the stress-strain curves for three undisturbed samples (θ = 0°, 45°, and 90°) with 0 D-W cycles, and stress-angle variation curves. The stress peak values of undisturbed samples, taken at various angles, exhibit differences due to the effects of anisotropy. Based on SEM images, it is evident that the Huai River high liquid limit clay exhibits significant anisotropy. Furthermore, as indicated in , the mechanical properties of high liquid limit clay with anisotropic strength have the following characteristics: under the same cell pressure conditions, the stress peak values of samples taken at different angles vary. With increasing sampling angles, the stress peak initially decreases and then increases. The maximum stress peak occurs in the vertical direction, the minimum near 45°, and the difference in stress peak between the maximum and minimum values is up to 40 kPa.
3.2.2. Stress-strain curves of structural high liquid limit clay after 1 cycle of D-W
shows stress-strain curves and porewater pressure-strain curves of reconstituted samples and undisturbed samples (θ = 0°, 45°, and 90°) of high liquid limit clay after 1 D-W cycle. Due to the impact of D-W cycles, the stress-strain and porewater pressure-strain curves of undisturbed samples experience alterations. As shown in , the stress-strain curves of reconstituted samples remain of the hardening type after D-W cycles, while the stress-strain curves of undisturbed samples taken from three different sampling angles change from hardening to softening, This phenomenon indicates that, under the same D-W cycle conditions, the mechanical property changes of high liquid limit clay with a structural nature are more pronounced compared to reconstituted samples. In practical engineering, this aspect is crucial for evaluating the mechanical properties of undisturbed clay under the influence of D-W cycles, and engineers should take note of this phenomenon.
Figure 11. Stress-strain curves and porewater pressure-strain curves of reconstituted samples and undisturbed samples (θ = 0°, 45°, and 90°) of high liquid limit clay after 1 D-W cycle (a)reconstituted samples stress-strain curves; (b) reconstituted samples porewater pressure-strain curves; (c) undisturbed sample in 0° direction stress-strain curves; (d) undisturbed sample in 0° direction porewater pressure-strain curves; (e) undisturbed sample in 45° direction stress-strain curves; (f)undisturbed sample in 45° direction porewater pressure-strain curves; (g) undisturbed sample in 90° direction stress-strain curves; (h)undisturbed sample in 90° direction porewater pressure-strain curves.
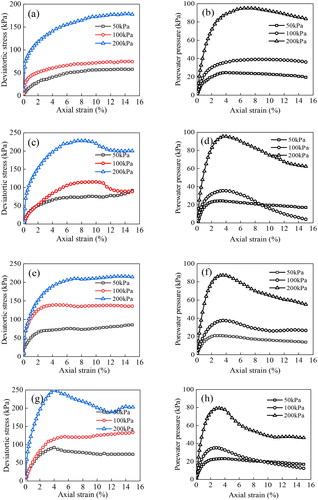
Regarding porewater pressure, as shown in , unlike undisturbed samples with 0 D-W cycles where a rare occurrence of an initial increase followed by a decrease in porewater pressure is observed in a few samples, both reconstituted and undisturbed samples display an initial increase followed by a decrease in porewater pressure after 1 cycle of D-W. This suggests that after undergoing 1 cycle of D-W, the soil’s compactness increases, making the sample more prone to shear dilation during the shearing process.
3.3. The effect of D-W cycles on the shear strength of structurally high liquid limit clay
3.3.1. The strength of structural high liquid limit clay with 0 D-W cycles
shows the shear strength envelopes for reconstituted and undisturbed samples (θ = 0°, 45°, and 90°) of high liquid limit clay with 0 D-W cycles. shows the shear strength parameters for reconstituted and undisturbed samples with 0 D-W cycles.
Figure 12. Shear strength envelope for reconstituted and undisturbed samples (θ = 0°, 45°, and 90°) of high liquid limit clay with 0 D-W cycles.
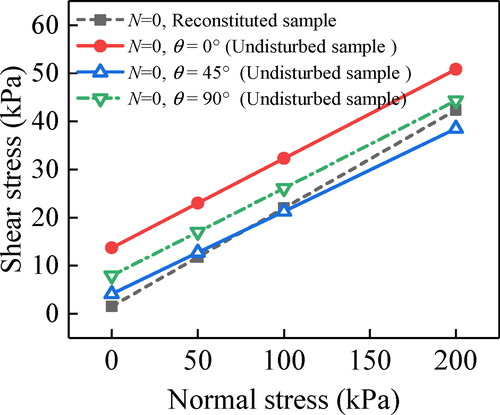
Table 5. Shear strength parameters for reconstituted samples and undisturbed samples (θ = 0°, 45°, and 90°) of high liquid limit clay with 0 D-W cycles.
Utilizing the Mohr-Coulomb Criterion, the shear strength envelopes and their associated parameters for reconstituted and undisturbed samples with 0 D-W cycles were obtained. Due to the influence of structure, the undisturbed samples and reconstituted samples taken at different angles exhibit different shear strengths and related parameters. As shown in , undisturbed samples exhibit higher shear strength compared to reconstituted samples, and the shear strength of undisturbed samples varies with different sampling angles. The primary reason for this is the presence of structural strength in undisturbed samples due to long-term consolidation, making the undisturbed high liquid limit clay exhibit higher shear strength than reconstituted samples.
Furthermore, during the natural consolidation process of the soil due to factors like deviator stress (gravity) and particle arrangement, the soil particles form a directional arrangement on a microscopic level, which manifests as anisotropy in undisturbed samples on a macroscopic level (Huang et al. Citation2016). This strength variation caused by natural sedimentation is noteworthy in slope, excavation, or tunnel engineering calculations (Liang et al. Citation2012). Based on the experimental results, it becomes apparent that high liquid limit clay formed through natural sedimentation typically exhibits higher strength in the vertical direction compared to other orientations. However, it’s essential to note that soil failure occurs along the weakest plane. In practical engineering applications, relying solely on the strength parameters of high plasticity clay in the vertical direction as a basis can pose substantial safety risks.
3.3.2. Structural high liquid limit clay strength after 1 cycle of D-W
shows the shear strength envelopes of reconstituted and undisturbed high liquid limit clay samples (θ = 0°, 45°, and 90°) after 1 cycle of D-W, and presents the shear strength parameters of these samples. Due to the impact of D-W cycles, the shear strength of structured high liquid limit clay experiences strengthening. As shown in , the shear strength envelope of high liquid limit clay after 1 D-W cycle is consistently positioned above that of 0 D-W cycles. Furthermore, as shown in , the internal friction angle of high liquid limit clay after 1 D-W cycle is approximately twice as high as that with 0 D-W cycles. This indicates that the shear strength of high liquid limit clay is enhanced after 1 D-W cycle, highlighting the influence of D-W cycles on the shear strength of high liquid limit clay.
Figure 13. Shear strength envelope for reconstituted samples and undisturbed samples (θ = 0°, 45°, and 90°) of high liquid limit clay after 1 D-W cycle.
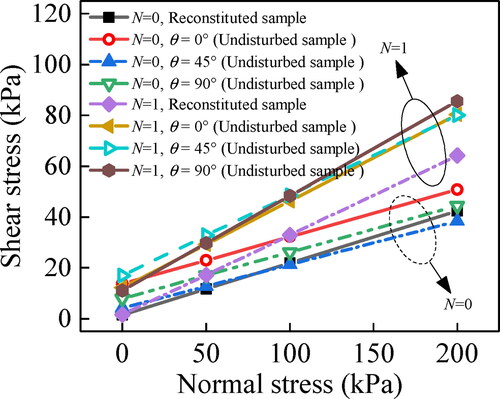
Table 6. Shear strength parameters for reconstituted samples and undisturbed samples (θ = 0°, 45°, and 90°) of high liquid limit clay after 1 D-W cycle.
3.3.3. Self-healing capability of high liquid limit clay
Contrary to previous literature conclusions regarding the deterioration of soil strength due to D-W cycles (Lü et al. Citation2009; Deng et al. Citation2017; Liu et al. Citation2021), the results for high liquid limit clays indicate an increase in soil strength. Some researchers have pointed out that during dehydration, samples are affected by matric suction, leading to particle aggregation and increased soil compaction. Simultaneously, the aggregation of particles leads to the creation of soil cracks, structural damage and degradation of mechanical properties (Mu et al. Citation2016; Liu et al. Citation2017; Tu et al. Citation2017). We observed cracks in the samples after D-W cycles, as shown in the . However, in recent years, research has found that for clay, the clay has a certain degree of self-healing behavior under the action of external forces. The higher the moisture content and clay content, the more obvious the self-healing effect (Lee Citation2020; El-Zein et al. Citation2021; Zhu et al. Citation2022). As shown in and , the clay content of high liquid limit clay is 42.6%, and the colloid content is 30%. It shows that high liquid limit clay has good self-healing ability. Overall, the increase in shear strength is mainly attributed to two factors: firstly, high liquid limit clay is significantly influenced by matrix suction under maximum dry stress conditions, leading to a substantial increase in the compactness of the non-cracked portion of the soil. Secondly, high liquid limit clay has a good self-healing ability, and some cracks are closed under the action of isotropic stress. The combined effect of these two factors results in an increase in shear strength in the samples.
Figure 14. Undisturbed high liquid limit clay samples (θ = 0°, 45°, and 90°) after 1 D-W cycle (a) θ = 0°; (b) θ = 45°; (c) θ = 90°.
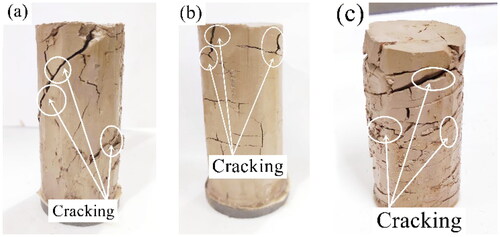
Based on the above analysis, it is preliminarily speculated that the failure of the Huaihe River slope is due to the long-term drought that causes the soil to experience large matric suction, and micro-cracks are formed during the aggregation of particles. Heavy rain causes wedging of the soil-bound water solvent film, rapid expansion of cracks, and destruction of the original structure (Deng et al. Citation2017; Yuan et al. Citation2017). In the subsequent period, the river side slope lacks K0 stress in the horizontal direction, making it difficult to self-heal. Consequently, collapse and damage occur under the hydraulic action of the river.
3.4. The impact of D-W cycles on the failure mode of structured high liquid limit clay
3.4.1. The failure mode of structured high liquid limit clay with 0 cycles of D-W
shows the failure modes of undisturbed samples of high liquid limit clay (θ = 0°, 45°, and 90°) under 100 kPa cell pressure with 0 D-W cycles. As shown in , the three types of failure modes for the samples are as follows: the samples undergo bulging deformation, and no clear shear bands are observed.
Figure 15. Failure mode of undisturbed samples of high liquid limit clay (θ = 0°, 45°, and 90°) under 100 kPa cell pressure with 0 D-W cycles (a) θ = 0°; (b) θ = 45°; (c) θ = 90°.
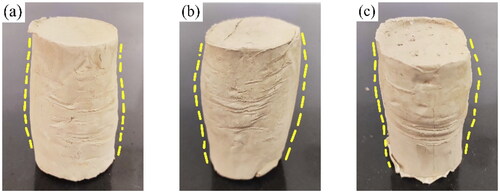
Yoshimine and Ishihara (Citation1998) categorized the undrained shear behavior of saturated sandy soils into four deformation and failure modes, as shown in . Ultimate steady state (UUS) refers to the final stable state of shear in sandy soil. Phase-transformation state (PTS) represents the state where there is a transition between shear contraction and shear dilation. Peak-stress state (PSS) is the state associated with the peak stress. Quasi-steady state (QSS) signifies the lowest stress level during the strain softening process, which is a quasi-stable state.
Figure 16. General undrained shear behavior of sand under large deformation (Yoshimine and Ishihara Citation1998).
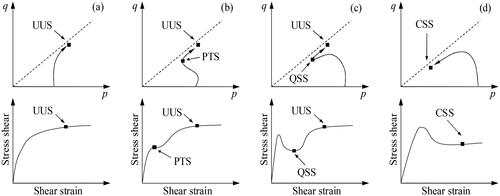
shows the effective mean principal stress paths of undisturbed samples of high liquid limit clay (θ = 0°, 45°, and 90°) with 0 D-W cycles. Previous research studies have indicated that the failure mode of most reconstituted clays is characterized by shear contraction (Guo and Wang Citation2011; Yao et al. Citation2012; Luo et al. Citation2013; Huang et al. Citation2016). As shown in , the effective mean principal stress paths of undisturbed samples under 50 kPa and 100 kPa cell pressures reveal a hardening-shear dilation behavior, setting them apart from conventional reconstituted clays. This suggests that the structural properties have a notable influence on the failure mode of high liquid limit clay. More precisely, the samples experience bulging deformation without the formation of well-defined shear bands.
3.4.2. The failure mode of structured high liquid limit clay after 1 cycle of D-W
shows the failure modes of undisturbed high liquid limit clay samples (θ = 0°, 45°, and 90°) under 100 kPa cell pressure after 1 D-W cycle. The failure mode of high liquid limit clay undergoes notable transformations due to the influence of D-W cycles. As shown in , the three types of failure modes for the samples are as follows: the sample exhibits minimal shear dilation, but distinct shear bands become evident.
Figure 18. Failure modes of undisturbed high liquid limit clay samples (θ = 0°, 45°, and 90°) under a 100 kPa cell pressure after 1 D-W cycle. (a) θ = 0°; (b) θ = 45°; (c) θ = 90°.
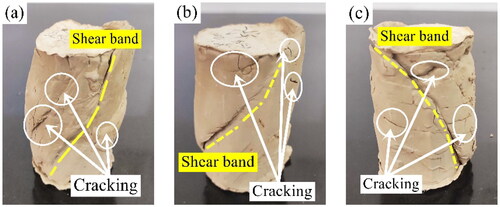
shows the effective mean principal stress paths of undisturbed samples of high liquid limit clay (θ = 0°, 45°, and 90°) after 1 D-W cycle. Due to the influence of D-W cycles, the effective mean principal stress paths of high liquid limit clay undergo notable alterations. As shown in , after 1 cycle of D-W cycle, the effective mean principal stress paths of undisturbed samples transition from a hardening-shear dilation mode to a softening-partial shear contraction-partial shear dilation mode under both 100 kPa and 200 kPa cell pressures. There are clear phase-transformation state (PTS) points in the effective mean principal stress paths of undisturbed samples. The analysis indicates that the shear contraction occurs due to the initial effective stress acting on the soil in the early stages of shearing, leading to the partial closure of cracks within the sample. Specifically, the structural damage caused by D-W cycles in undisturbed samples generates cracks. In the early stages of shearing, some of the effective stress acts on these cracks, resulting in gap closure and shear contraction. Subsequently, the D-W cycles cause the soil skeleton to contract, increasing its density and exhibiting characteristics of over-consolidated soil. As the deviator stress continues to increase, shear dilation occurs. Therefore, the effective stress path transitions from a hardening-shear dilation mode to a softening-partial shear contraction-partial shear dilation mode, indicating that D-W cycles influence the failure mode of structured high liquid limit clay. This is characterized by a less pronounced shear dilation phenomenon in the failed samples and the presence of distinct shear bands.
3.5. The influence of D-W cycles on the anisotropic structure of high liquid limit clay
shows the stress peak variation with cell pressure for undisturbed samples (θ = 0°, 45°, and 90°) of high liquid limit clay under 0 and 1 D-W cycle.
Figure 20. Stress peak variation with cell pressure for undisturbed samples (θ = 0°, 45°, and 90°) of high liquid limit clay under 0 and 1 D-W cycle (a) high liquid limit clay with 0 cycles of D-W; (b) high liquid limit clay after 1 cycle of D-W.
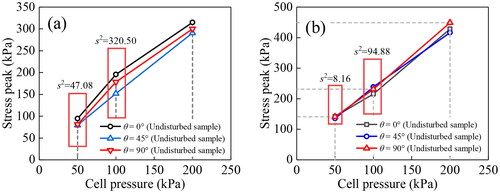
To investigate the impact of D-W cycles on the anisotropy of high liquid limit clay, the degree of dispersion of stress peak values for samples at different angles under the same cell pressure is used as a measure of anisotropy. That is, the greater the degree of dispersion, the more pronounced the anisotropy. EquationEquation (2)(2)
(2) represents the formula for calculating the variance of stress peak values for undisturbed samples after different numbers of cycles under a specific cell pressure, and the magnitude of the variance indicates the degree of dispersion.
(2)
(2)
where s2 is the variance of the stress peak of undisturbed samples at a specific cell pressure, n is the number of different sampling angles, Xi is the stress peak at different angles (i = θ), and
is the mean stress peak at various angles under a specific cell pressure.
shows the variance of stress peak values for undisturbed samples (θ = 0°, 45°, and 90°) under 0 and 1 D-W cycles. To eliminate the influence of excessively high cell pressures and weaken or eliminate the effects of anisotropy, cell pressures of 50 kPa and 100 kPa were chosen. The variance of the peak stress was calculated using EquationEquation (2)(2)
(2) .
Table 7. Variance of stress peak values for undisturbed samples (θ = 0°, 45°, and 90°) under 0 and 1 D-W cycle.
As shown in , after 1 cycle of D-W cycles, the variance values for undisturbed samples are lower than those with 0 cycles. Under a cell pressure of 50 kPa, the variance decreased by a factor of 5.8, and under a cell pressure of 100 kPa, it decreased by a factor of 3.4. This reduction in variance indicates a decrease in the differences in strength among the three undisturbed samples, suggesting that D-W cycles weaken the anisotropy of structured high liquid limit clay. From a mechanical perspective, deviatoric stresses can cause variations in the arrangement and compaction of particles in different directions, leading to different forms of anisotropy. An initial stress state in which there is isotropic stress (spherical stress) will not exhibit anisotropy (Wheeler et al. Citation2003; Luo et al. Citation2013; Li et al. Citation2016). Natural high liquid limit clay experiences initial directional alignment (K0 consolidation) due to gravitational factors, resulting in differences in the peak stress when shear occurs in different directions in the undisturbed soil. Consequently, under 0 cycles of D-W cycles, the stress peaks of the three undisturbed samples are different, and the degree of dispersion is higher, resulting in larger variance values. After undergoing the D-W cycles, phase, undisturbed samples experience significant crack development, causing structural damage and weakening of anisotropy. During the isotropic consolidation phase, the additional stress is applied in the form of spherical stress to the damaged samples, enhancing the isotropic particle arrangement characteristics (Gao et al. Citation2020). Therefore, structured undisturbed high liquid limit clay transitions from an anisotropic state to an isotropic state after experiencing D-W cycles and isotropic consolidation.
There are some limitations in the application of the results from our research, The high liquid limit clay of the Huaihe River is a typical regional soil, due to the differences in the physicomechanical properties of structured clay in different regions, and the results in the manuscript may not be applied to other structured clays.
4. Conclusions
Based on the failure of the Huaihe River slope, this paper studied the effects of D-W cycles on the structure and mechanical properties of undisturbed high liquid limit clay through unconfined compression tests, SEM tests and triaxial shear tests. the conclusions are drawn as follows:
After undergoing D-W cycles, the changes in the mechanical properties of undisturbed high liquid limit clay with good structural characteristics are more pronounced compared to reconstituted samples. Specifically, the stress-strain curves of reconstituted samples still exhibit hardening behavior after undergoing D-W cycles, whereas the stress-strain curves of undisturbed samples transition from hardening to softening.
D-W cycles have a significant impact on the mechanical properties of structured high liquid limit clay. It manifests as a transition in the stress-strain curve from hardening to softening behavior, a shift in the porewater pressure-strain curve from an initial increase followed by stability to an initial increase followed by a decrease, and the fact that structured high liquid limit clay exhibits higher shear strength after undergoing D-W cycles compared to clay that hasn’t undergone any cycles.
D-W cycles alter the effective stress path of structured high liquid limit clay, thereby significantly influencing the failure mode. The failure mode shifts from a hardening-shear dilation mode to a softening-partial shear contraction-partial shear dilation mode. The specific manifestation of the failed samples changes from bulging deformation without distinct shear bands to less significant shear dilation with distinct shear bands.
PTS points emerge due to the fact that during the initial stages of shearing, a fraction of the effective stress is exerted on the cracks within the sample, causing these cracks to partially close and consequently inducing shear contraction.
A method for assessing the degree of anisotropy based on the variance of stress peak values is proposed. The results indicate that D-W cycles weaken the anisotropy of high liquid limit clay. Under 50kPa and 100kPa cell pressure conditions, the variance of stress peak values for high liquid limit clay samples taken from different orientations after 1 cycle of D-W is smaller than that with 0 cycles, indicating that D-W cycles reduce the directional nature (anisotropy) of structured high liquid limit clay.
Data availability statement
All data used during the study are available from the corresponding author by request.
Disclosure statement
The authors declared that there is no conflict of interest to this work.
Additional information
Funding
References
- AASHTO M. 1991. Standard specification for classification of soils and soil-aggregate mixtures for highway construction purposes. Washington, DC: Aashto.
- Bradford J. 1981. The shear strength of a moderately well‐structured soil in its natural and remolded states. Soil Sci Soc Am J. 45(1):9–12. doi: 10.2136/sssaj1981.03615995004500010002x.
- Cai ZY, Chen H, Huang YH, Zhang C. 2019. Failure mechanism of canal slopes of expansive soils considering action of wetting-drying cycles. Chin J Geotech Eng. 41:1977–1982.
- Deng HF, Xiao Y, Fang JC, Zhang HB, Wang CXJ, Cao Y. 2017. Shear strength degradation and slope stability of soils at hydro-fluctuation belt of river bank slope during drying-wetting cycle. Rock Soil Mech. 38:2629–2638.
- El-Zein A, Airey D, Yu B, Esgandani GA, Proust G, Dias-da-Costa D, Gao Y, Gan Y, Chen S. 2021. Self-repair of cracks and defects in clay: a review of evidence, mechanisms, theories and nomenclature. Acta Geotech. 16(12):3741–3760. doi: 10.1007/s11440-021-01382-8.
- Gao Q-F, Hattab M, Jrad M, Fleureau J-M, Hicher P-Y. 2020. Microstructural organization of remoulded clays in relation with dilatancy/contractancy phenomena. Acta Geotech. 15(1):223–243. doi: 10.1007/s11440-019-00876-w.
- Guo Y, Wang YX. 2011. Comparative test study on consolidation undrained shear characteristics of undisturbed and remolding silt. J Hydraul Eng. 42:68–75.
- Hao YZ, Wang TH, Zhao W, Xin J. 2021. Experimental study on triaxial shear characteristics of compacted loess under drying and wetting cycles. J Hydraul Eng. 52:359–368.
- Huang MS, Yao YP, Yin ZY, Liu EL, Lei HY. 2016. An overview on elementary mechanical behaviors, constitutive modeling and failure criterion of soils. Tumu Gongcheng Xuebao/China Civil Eng J. 49:9–35.
- Jiang JJ, Cui ZD. 2022. Instability of high liquid limit soil slope for the expressway induced by rainfall. Appl Sci. 12(21):10857. doi: 10.3390/app122110857.
- JTGF10. 2006. 公路路基施工技术规范 [S].
- Lee S. 2020. Drying cracks network in soils: remedial solutions and 3-D ERT monitoring [D].
- Li L, Zang M, Zhang RT, Lu HJ. 2022. Deformation and strength characteristics of structured clay under different stress paths. Math Prob Eng. 2022:1–16. doi: 10.1155/2022/9266206.
- Li SQ, Xia JH, Zhang PY. 2016. THE INITIAL STRESS LINE AND MODIFIED MOHR-COULOMB CRITERIONS FOR UNDISTURBED SOIL. 工程力学. 33:116–122.
- Li SQ, Zhang LD, Xia JH, Kong DZ. 2014. Initial stress state for undisturbed soil and ameliorated structural model based on mohr-coulomb criterion. China J Highway Trans. 27:1.
- Li SQ, Zheng G, Cui CY, Liu SJ. 2010. Pedigree cluster method to evaluate geometrica anisotropy of soil micro-structure [J]. Chin J Geotech Eng. 32:109–114.
- Liang QG, Zhao L, An YF, Zhang YJ. 2012. Preliminary study of anisotropy of Q 4 loess in Lanzhou. Rock Soil Mech. 33:17–23.
- Liang ZX, Sun H, Huang ZX, Niu FJ. 2023. The influences of natural structure damage and stress path on mechanical behaviors of soft clay. Bull Eng Geol Environ. 82(6):1–15. doi: 10.1007/s10064-023-03237-w.
- Liu WH, Yang Q, Sun XL, Hua Y. 2017. Influence of drying stress history on the mechanical behaviors of silty clay under saturated condition. J Hydraul Eng. 48:203–209.
- Liu Y, Chen DX, Wang H, Yu JJ. 2021. Response analysis of residual soil slope considering crack development under drying − wetting cycles. Rock Soil Mech. 42:6.
- Lü HB, Zeng ZT, Zhao YL, Lu H. 2009. Experimental studies of strength of expansive soil in drying and wetting cycle. Rock Soil Mech. 30:3797–3802.
- Luo KT, Nie Q, Zhang SY, Liu EL. 2013. Investigation on artificiallystructured soils with initial stress-induced anisotropy. Rock Soil Mech. 34:2815–2820.
- Ma Q, Hu ZL, Hu Z, Li JH. 2022. Strength characteristics and micro-scale mechanism of high liquid limit clay treated by recycled construction and demolition wastes (CDW) aggregates. Constr Build Mater. 332:127367. doi: 10.1016/j.conbuildmat.2022.127367.
- Mu K, Kong LW, Zhang XW, Yin S. 2016. Experimental investigation on engineering behaviors of red clay under effect of wetting-drying cycles. Rock Soil Mech. 37:2247–2253.
- Newson T, Dyer T, Adam C, Sharp S. 2006. Effect of structure on the geotechnical properties of bauxite residue. J Geotech Geoenviron Eng. 132(2):143–151. doi: 10.1061/(ASCE)1090-0241(2006)132:2(143).
- Ng CWW, Mu Q, Zhou C. 2017. Effects of soil structure on the shear behaviour of an unsaturated loess at different suctions and temperatures. Can Geotech J. 54(2):270–279. doi: 10.1139/cgj-2016-0272.
- Standardization Administration of China (SAC), Ministry of Construction. 2019. China National Standards GB/T 50123–2019: standard for geotechnical testing method. Beijing: China Planning Press.
- Shao SJ, Zhou FF, Song CX. 2006. Analysis of moistening and compression deformation of loess considering soil structure variations. Tumu Gongcheng Xuebao (China Civil Eng J). 39:94–99.
- Sun D, Sun WJ, Xiang L. 2010. Effect of degree of saturation on mechanical behaviour of unsaturated soils and its elastoplastic simulation. Comput Geotech. 37(5):678–688. doi: 10.1016/j.compgeo.2010.04.006.
- Tu YL, Liu XR, Zhong ZL, Wang S, Wang ZJ, Ke W. 2017. Experimental study on strength and deformation characteristics of silty clay during wetting-drying cycles. Rock Soil Mech. 38:3582–3589.
- Wang LZ, Ding L, Chen YM, Li LL. 2004. Study on compressibility of structured soft soil. China Civil Eng J. 37:46–53.
- Wang LZ, Shen KL. 2007. A constitutive model of K 0 consolided structured soft clays. Chin J Geotech Eng. 29:496–504.
- Wheeler SJ, Näätänen A, Karstunen M, Lojander M. 2003. An anisotropic elastoplastic model for soft clays. Can Geotech J. 40(2):403–418. doi: 10.1139/t02-119.
- Xiao J, Yang HP, Li HF, Tang XY. 2013. Shear strength test of Nanning expansive soil with various dry densities and low stresses. China J Highway Transport. 26:15.
- Xie DY, Qi JL. 1999. Soil structure characteristics and new approach in research on its quantitative parameter. Chin J Geotech Eng. 21:651–656.
- Yang HP, Tang XY, Wang XZ, Xiao H, Ni X. 2018. Shear strength of expansive soils under wet-dry cycles with loading. Rock and Soil Mechanics. 39:2311–2317.
- Yao YP, Liu L, Luo T, Tian Y, Zhang JM. 2019. Unified hardening (UH) model for clays and sands. Comput Geotech. 110:326–343. doi: 10.1016/j.compgeo.2019.02.024.
- Yao YP, Zhang BY, Zhu JG. 2012. Behaviors, constitutive models and numerical simulation of soils. China Civil Eng J. 45:127–150.
- Yoshimine M, Ishihara K. 1998. Flow potential of sand during liquefaction. Soils Found. 38(3):189–198. doi: 10.3208/sandf.38.3_189.
- Yuan ZH, Ni WK, Tang C, Hu SM, Gan JJ. 2017. Experimental study of structure strength and strength attenuation of loess under wetting-drying cycle. Rock Soil Mech. 38:1894. +
- Zhang JM, Zhou Z, Lin F, Yang QG, Luo Y. 2021. Failure mechanism of a slow-moving landslide on September 27, 2020, in Chang Nong Village, Guangxi, China. Landslides. 18(7):2575–2592. doi: 10.1007/s10346-021-01688-4.
- Zhang XW, Wang CM, Li JX. 2010. Structural characteristics and the damage mechanism of soft clay. J China Univ Mining Technol. 39:373–379.
- Zhu QY, Yin ZY, Wang JH, Xia XH. 2015. One dimension compression model for natural clays considering structure disturbance. J Civil Architect Environ Eng. 34(3):28–33.
- Zhu YB, Zheng HH, Lan HX, Liu YW, Li LP, Fu BY, Du CC. 2022. Effect of initial water content and dry density on the self-healing of desiccation cracks in compacted hipparion red clay. Front Earth Sci. 10:963086. doi: 10.3389/feart.2022.963086.