ABSTRACT
The gut microbiota plays an important role in cardio-metabolic diseases with diet being among the strongest modulators of gut microbiota composition and function. Resistant dietary carbohydrates are fermented to short-chain fatty acids (SCFAs) by the gut bacteria. Fiber and omega-3 rich diets increase SCFAs production and abundance of SCFA-producing bacteria. Likewise, SCFAs can improve gut barrier integrity, glucose, and lipid metabolism, regulate the immune system, the inflammatory response, and blood pressure. Therefore, targeting the gut microbiota with dietary strategies leading to increased SCFA production may benefit cardio-metabolic health. In this review, we provide an overview of the association between diet, SCFAs produced by the gut microbiota and cardio-metabolic diseases. We first discuss the association between the human gut microbiota and cardio-metabolic diseases, then investigate the role of SCFAs and finally explore the beneficial effects of specific dietary interventions that can improve cardio-metabolic outcomes through boosting the SCFA production.
Introduction
Cardio-metabolic diseases (CMD) are the most common cause of morbidity and mortality worldwide, representing a major public health challenge.Citation1; Citation2 Indeed, 25% of the total population is estimated to have CMD, and approximately 30% of all the deaths are caused by CMDs.Citation3
There are many well-established genetic and environmental risk factors associated to CMD including high blood pressure, high cholesterol, smoking, diabetes, abdominal obesity, insulin resistance, glucose intolerance among others. Recent studies suggest that gut microbiota imbalance also plays an important role in the development and progression of CMDs,Citation4–7 including type-2 diabetes (T2D), obesity, atherosclerosis, heart failure, myocardial fibrosis, and atrial fibrillation. Moreover, the gut-brain axis has been implicated in neurogenic hypertension,Citation8 which is particularly relevant for people suffering from obstructive sleep apnea,Citation9 and gut microbiome composition has been linked to sleep architecture in these patients.Citation10 Given the strong link between inflammation and sleep apnea, it is likely that SCFAs/gut microbiome links relating to hypertension may also link to sleep apnea and other disorders.Citation11
Gut microbiota can exert beneficial or detrimental effects in human healthCitation12 through the production of metabolic products and signaling molecules, which influence diverse functions in different organs.Citation13 Among these bacteria-derived metabolites, short-chain fatty acids (SCFA) are gaining attention as a potential focus of CMD.Citation14 SCFAs, namely acetate, propionate, and butyrate, are produced through bacterial fermentation of fibers (e.g., resistant starch, simple sugars, and polysaccharides),Citation15 and present regulatory functions in the lipids, cholesterol and glucose metabolism, anti-inflammatory and immune response and gut barrier integrity.Citation16 SCFAs might protect against CMD as they are able, for instance, to decrease plasma cholesterol and glucose levels and increase fatty acid oxidation.Citation17 Likewise, the type of diet can modulate SCFA production and/or abundance of SCFA-producing bacteria. For instance, a Mediterranean diet, characterized by a high consumption of fiber-rich food, or omega-3-rich diets, has been correlated with higher levels of SCFAs and SCFA-producing bacteria.Citation18,Citation19 Previous reviews addressed SCFAs, CMD or, diet and their links to gut microbiome composition,Citation20–25 however none of them has yet simultaneously covered the role of SCFAs in the interplay between diet, gut microbes, and CMD. Indeed, most of the previous reviews do not discuss in detail SCFAsCitation23 nor their beneficial role in cardio-metabolic health,Citation20 nor how different types of diet can increase SCFAs production to improve the health of patients suffering from CMDs.Citation21,Citation22,Citation24,Citation25 In the present review, on the contrary, we specifically focus on the association between the human gut microbiota and CMDs, the metabolic routes involved in the SCFA production, the benefits exerted by SCFAs in cardio-metabolic health, and the involved mechanisms. Finally, we discuss a number of recent clinical studies supporting the beneficial effects of specific dietary interventions that can improve cardio-metabolic outcomes through boosting SCFAs production.
Gut microbiota
The human intestine contains approximately 1014 microorganisms, collectively known as the gut microbiota.Citation26 The gene repertoire present in these microbes is 100-fold higher than the number of genes present in the human genome.Citation27 In a healthy gut microbiota, the most predominant phyla are Firmicutes and Bacteroidetes (90% of the population), followed by Actinobacteria and Verrucomicrobia,Citation28 although inter-variability between individuals exists. Gut microbiota diversity, richness, and composition vary depending on multiple determinants, either endogenous such as sex, microbial interactions, and host genotype,Citation26,Citation29 or exogenous, such as diet, age, usage of antibiotics, exercise, smoking, and stress.Citation30
Over the last years, advances in bioinformatics tools and next-generation sequencing have increased our knowledge on the relationship between microbiota organisms and humans,Citation31 allowing us to discover the benefits and detriments of the gut bacteria to human health. Bacteria-derived metabolites play important functions in the intestine (e.g., digestion, energy harvest, and barrier integrity)Citation32 and even in other organs when they enter into the systemic circulation (e.g., glucose circulation in the pancreas, lipid metabolism in the liver, and cognitive functions in the brain).Citation13 When there is an intestinal microbial ecosystem balance (eubiosis), the gut microbiota plays important immunological, homeostatic, and metabolic functions that maintain the human host health.Citation33 On the other hand, the imbalance of the gut microbiota, known as dysbiosis, and reduction of bacterial diversity can lead to a variety of metabolic abnormalities, such as inflammation and oxidative stress, impacting negatively on the host pathophysiologic and physiology conditions.Citation34
Gut microbiota in cardio-metabolic health
The role of the gut microbiota has recently been implicated in the development and progression of CMD.Citation35,Citation36 Many studies have shown alterations in the composition and function of the gut bacteria in patients suffering from CMDs. Animal and human obesity has been associated with an increased Firmicutes/Bacteroidetes ratio.Citation37 Moreover, gut dysbiosis can reduce the gut barrier integrity, affecting glucose sensibility and absorption, leading to insulin resistance and T2D.Citation38 Another example is the lipopolysaccharides (LPS) present in the Gram-negative bacteria cell wall, which can trigger the immune system response and potentiate cardiovascular diseases (CVD) pathogenesis.Citation39,Citation40
Our body can functionally interact with bacteria metabolic products,Citation16 and cardio-metabolic health (CMH) is associated with these metabolic products. Trimethylamine (TMA),Citation41–45 bile acidsCitation46–49 are examples of metabolic products that have been negatively associated with CMD, whereas SCFAs,Citation14 anthocyaninsCitation50 and indoleproprionic acidCitation51 might influence positively the host health.
TMA is metabolized from choline-containing compounds (e.g., choline, betaine, and L-carnitine) present in human diet by the gut microbiota.Citation52 Then, TMA enters to the portal circulation, where is oxidized by liver enzymes to produce trimethylamine‑N‑oxide (TMAO).Citation53 TMAO pathway has been associated with atherosclerosis and thrombosis promotion in mouse,Citation41–43 and with CMD in humans such as obesity, chronic kidney disease, and T2D.Citation44,Citation45,Citation54 Detailed therapeutic potential and clinical prognostic of TMAO in CMD can be found in several reviews.Citation55–57
Gut microbiota is responsible for the generation of unconjugated free bile acids and secondary bile acids through deconjugation and dihydroxylation reactions.Citation58 Bile acids can act as signaling molecules involved in inflammation, host metabolism, and energy expenditure, and thus, they might play a role in CMD.Citation46–49 The role of bile acids in metabolic disorders and CVD has been reviewed by.Citation59,Citation60
Anthocyanins are glycosyl-anthocyanidins present in plant vacuoles. Gut bacteria can degrade anthocyanins, generating protocatechuic acid and free anthocyanidins.Citation61 Protocatechuic acid can influence positively atherosclerosis and CVD, thanks to its anti-inflammatory and antioxidant properties.Citation50
Indoleproprionic acid is a compound synthetized from tryptophan by a reduced number of bacterial strains.Citation62 Circulating levels of indoleproprionic acid are negatively correlated with different metabolic syndrome parameters,Citation51 and higher levels of this compound have been also associated with a lower risk of developing T2D.Citation63
SCFAs are the most well-studied gut bacteria-derived metabolites and they have been suggested as potential disease-mitigating factors and/or disease preventing in CMD, including T2D, obesity, and CVD, among others.Citation14,Citation64 SCFAs will be explained in detail in the below sections.
Hence, CMD development might be modulated via specific beneficial bacteria-derived metabolites. Likewise, dietary interventions can have a profound effect on their production,Citation65,Citation66 being very important to investigate the role of these metabolites on human health and their modulation by different diets.
Short-chain fatty acids
Fatty acids are carboxylic acids with an aliphatic chain, which can be saturated or unsaturated.Citation67 Depending on the length of their aliphatic tails, fatty acids can be classified as short (<6 C), medium (6–12 C), or long (>12 C) chain fatty acids. SCFAs include formate (C1), acetate (C2), propionate (C3), butyrate (C4), and valerate (C5), and their chemical properties depend on the number of carbons.Citation68
SCFAs are produced by anaerobic gut bacteria through saccharolytic fermentation of complex resistant carbohydrates (e.g., fructo-oligosaccharides, sugar alcohols, resistant starch, inulin, and polysaccharides from plant cell walls), which escape digestion and absorption in the small intestine.Citation69 As a result of the fermentative reactions, some gases, including hydrogen, methane, and carbon dioxide are generated.Citation70 It is estimated that the fermentation of 50–60 g carbohydrates per day yields to the approximated production of 500–600 mmol of SCFAs in the gut.Citation71 Amino acids can be also fermented to produce SCFAs.Citation72 Although SCFAs are dependent on diet and bacteria present in the gut, there are specific foods containing SCFAs, for instance, vinegar, sourdough bread, and some dairy products such as crème fraiche, butter, and cheese.Citation73
The major SCFAs formed by the gut bacteria are acetate, propionate, and butyrate which account for approximately 80% of all SCFAs and will be the focus of this review. In order to comprehensively understand the effect of these metabolites on human health, it is essential to consider the production site and the gradient along different cells and tissues (). Fermentation takes place in the large intestine, mainly at the right side, and the SCFA absorption occurs rapidly from the human colon.Citation74 Changes in pH vary depending on the SCFA concentration.Citation75 In the cecum, the pH is more acidic and the SCFA concentrations are higher than in the sigmoid/rectum, where the pH is higher. In the colon and stool, butyrate, propionate, and acetate are found in an approximate molar ratio of 20:20:60, respectively,Citation76 although these values vary depending on the microbiota composition, SCFA substrates, and gut transit time.Citation77 Additionally, a strong gradient from the gut lumen to the periphery exists, leading to different cell SCFA exposure.Citation78 Most SCFAs are utilized by colonocytes as an energy source.Citation71 The SCFAs that are not used by these cells can be transported toward the hepatic portal vein. Acetate, propionate, and butyrate concentrations in portal blood (375 µmol/l) are almost 5 times greater than peripheral venous blood (79 µmol/l), suggesting that the gut is a principal SCFA source, whereas SCFA concentrations in the hepatic vein SCFA (148 µmol/l) are 39% of those found in portal blood.Citation76
Figure 1. Overview of the production and absorption sites, and transport of acetate, propionate and butyrate (SCFAs)
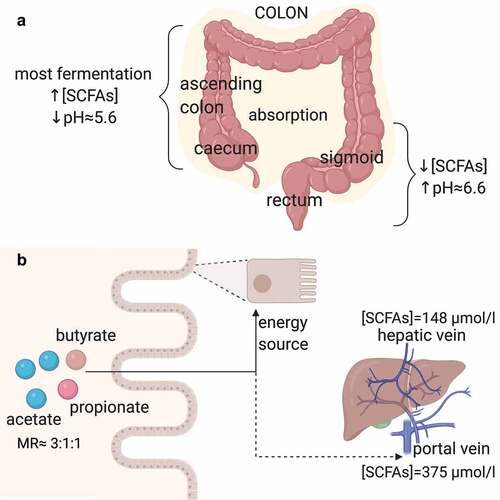
Metabolic routes to produce SCFA and SCFA-producing bacteria
The pathways involved in the SCFA production have been recently described in detail.Citation79 In addition, metagenomic analyses have allowed the characterization of the major SCFA-producing bacteriaCitation21 ().
Figure 2. SCFA biosynthesis pathways from the dietary carbohydrate fermentation and the major SCFA-producing bacteria for each pathway
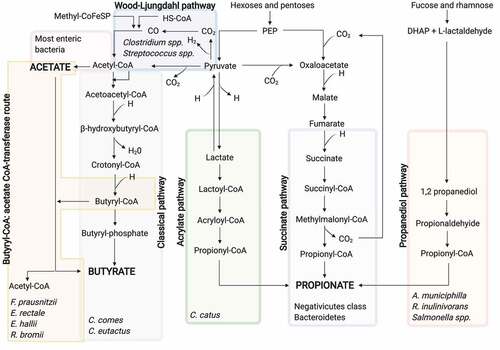
Acetate formation
Acetate can be synthesized through two different pathways. Firstly, acetyl-CoA can be produced by decarboxylation of pyruvate, then, acetyl-CoA is hydrolyzed to acetate by an acetyl-CoA hydrolase.Citation80 Most of the acetate is produced by enteric bacteria, including Prevotella spp., Ruminococcus spp., Bifidobacterium spp., Bacteroides spp., Clostridium spp., Streptococcus spp., A. muciniphila, and B. hydrogenotrophica, using this pathway.Citation81 Secondly, the Wood-Ljungdahl pathway can be also used by acetogenic bacteria to form acetate from acetyl-CoA. Here, the reduction of carbon dioxide generates carbon monoxide, which reacts with a coenzyme A molecule and a methyl group to produce acetyl-CoA. At the same time, acetyl-CoA is the substrate to obtain acetate.Citation82
Propionate formation
Although propionate-producers are distributed across several phyla, only a few bacterial genera are able to form propionate, and unlike acetate, the utilized propionate pathways are more conserved and substrate specific.Citation83
Propionate can be synthesized through three different biochemical pathways, namely succinate, acrylate, and propanediol pathway.Citation83 In the succinate pathway, the primitive electron transfer chain using phosphoenolpyruvate (PEP) can be utilized to generate propionate.Citation84 Specifically, PEP is carboxylated to oxalacetate, and then oxalacetate is sequentially converted into malate and fumarate. The latter accepts electrons from NADH using a fumarate reductase and a NADH dehydrogenase, which form a simple electron-transfer chain. The NADH dehydrogenase transport protons across the cell membrane. These protons are utilized for chemiosmotic ATP synthesis. Likewise, succinate is generated as a result of the fumarate reductase. When the carbon dioxide partial pressure is low, succinate is transformed to methylmalonate, which leads to propionate and carbon dioxide. The latter can be recycled for the PEP carboxylation, repeating the process. BacteroidetesCitation85 and several Firmicutes belonging to the Negativicutes classCitation86 use this pathway for the propionate formation. Besides, acrylate pathway can be used to reduce lactate to propionate by a lactoyl-CoA dehydratase.Citation80 This pathway is only present in a very reduced number of gut bacteria, including Coprococcus catus.Citation83 Lastly, 1,2-propanediol can be formed from deoxy sugars such as rhamnose and fucose in the propanediol pathway. Likewise, 1,2-propanediol is sequentially converted into propionaldehyde and propionyl-CoA, which leads to the propionate formation.Citation87 Salmonella enterica serovar TyphimuriumCitation88 and R. inulinivoransCitation89 are bacteria utilizing this pathway, just as Akkermansia municiphilla which appears to be the major propionate-producing species.Citation90
Butyrate formation
Butyrate production, like propionate, is more conserved and substrate specific.Citation83 Resistant starch fermentation highly contributes to the formation of butyrate in the colon, with Ruminococcus bromii the main producer as its absence has been associated with a reduction in the resistant starch fermentation.Citation91
To form butyrate, first, two acetyl-CoA molecules must be condensed to obtain acetoacetyl-CoA, which is subsequently reduced to β-hydroxybutyryl-CoA, crotonyl-CoA and lastly to butyryl-CoA. In the case of lactate-utilizing bacteria, acetyl-CoA can be produced from lactate.Citation92 From butyryl-CoA, butyrate can be synthesized following two different pathways. In the pathway referred to as classical, phosphotransbutyrylase and butyrate kinase enzymes are responsible for such a conversion.Citation93 In the second pathway, butyryl-CoA: acetate CoA-transferase converts butyryl-CoA into butyrate and acetyl-CoA using exogenously derived acetate. The latter pathway seems to be preferred by the human gut microbiota rather than the classical pathway,Citation94 which is limited to some Coprococcus species.Citation79 F. prausnitzii, E. rectale, E. hallii, and R. bromii present this pathway and appear to be the major butyrate producers.Citation95
Beneficial roles of SCFA in cardio-metabolic health and involved mechanisms
SCFAs act as signaling molecules on both the gut cells and other tissue cells. This is possible due to six receptors to which SCFAs can bind, triggering intracellular signaling cascades: free fatty acid receptor 3 (FFAR3 or GPR41), FFAR2 (also known as GRP43), G-protein coupled receptor 109a (GPR109a or HCAR2), olfactory receptor-78 (Olfr78 in mice or OR51E2 in humans), GPR42 and OR51E1, being the four first the most well-studied.Citation16 Olfr78 mainly binds acetate and propionate, leading to an increase of cyclic adenosine monophosphate (cAMP) and renin releaseCitation96 and is expressed in the vascular smooth muscle cells in the peripheral vasculature and renal afferent arteriole.Citation97 FFAR3, FFAR2, and GPR109a are expressed by different organs and cells: small intestine, colon, liver, spleen, heart, skeletal muscle, neurons, immune cells, and adipose tissues.Citation98 Additionally, depending on the length of their aliphatic tails, the receptors present different affinities for SCFAs. FFAR2 prefers binding to acetate and propionate, whereas FFAR3 binds propionate, butyrate, and acetate with a lower affinity,Citation99 and GPR109a mainly binds butyrate.Citation100 Moreover, butyrate and propionate play an important role in transcriptional regulations and post-translational modifications, as they appear to strongly inhibit lysine and histone deacetylase (K/HDAC) activity.Citation16,Citation101 Such an inhibition leads to histone hyperacetylation, which turns in a higher accessibility of transcription factors to the promoter regions of different genes.Citation102 Likewise, butyrate is a ligand of two transcription factors: peroxisome proliferator-activated receptor γ (PPARγ)Citation103 and aryl hydrocarbon receptor.Citation104 Thanks to these and direct mechanisms, SCFAs can play beneficial roles in human health, such as improvement of gut barrier integrity, regulation of the blood pressure and energy intake and energy use, modulation of glucose and lipid metabolism, and mediation of the immune system and anti-inflammatory response ().
Figure 3. Beneficial roles of SCFA in cardio-metabolic health and the indirect mechanisms involved
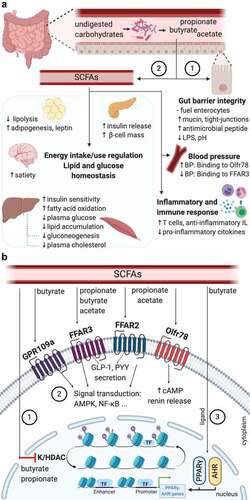
Blood pressure
Several recent studies have analyzed the association between SCFAs and blood pressure. The results seem contradictory, as some studies have reported that SCFAs can cause hypertension,Citation105,Citation106 and others have shown that SCFAs can decrease blood pressure.Citation107,Citation108 For instance, studies with Olfr78 null mice present lower blood pressure compared with the wild-type mice.Citation106 On the other hand, FFAR3 KO mice are hypertensive.Citation107 The potential dual effect of both receptors in the regulation of blood pressure has been analyzed by Pluznick et al. (2013).Citation97 In this study, blood pressure was measure in Olfr78 null mice and wild-type mice before and after an antibiotic treatment. After the treatment, the SCFA levels decreased. Moreover, the wild-type mice presented a slight increase in blood pressure due to the decreased SCFA levels, whereas the Olfr78 null mice had a higher blood pressure increase. Thus, the signaling of both receptors in the wild-type mice can better balance the blood response even with lower SCFA levels, whereas Olfr78 null mice presents only the FFAR3 signaling, which results in a higher blood pressure response. Besides, it is important to note that the ligand affinity of Olfr78 for SCFAs is lower than the one presented in FFAR3.Citation109 Taken together, the SCFA receptors Olfr78 and FFAR3 might play an opposing role in the regulation of blood pressure, balancing each other to have a healthy blood pressure.Citation97 Moreover, using a Mendelian Randomization approach, Sun and coworkersCitation110 reported T2D may causally affect hypertension and particularly higher systolic blood pressure. On the other hand, the relationship from hypertension to T2D is unlikely to be causal, highlighting the importance of keeping an optimal glycemic profile in general populations. Therefore, SCFAs might also be beneficial in hypertension by improving glucose homeostasis.Citation111,Citation112
Gut barrier integrity
It is well recognized that SCFAs are necessary substrates for the colonic epithelium maintenance, with butyrate being the preferred oxidative fuel by colonocytes.Citation113 Butyrate can induce proliferation in normal colonocytes, but also terminal differentiation and apoptosis in neoplastic cells. This dual role is known as the “butyrate paradox” or “Warburg effect”.Citation101,Citation114 Additionally, intestinal epithelial cells (IEC) are connected by transmembrane proteins, namely, tight-junctions, adherent junctions, and desmosomes. SCFAs, in particular butyrate, seem to improve the epithelial barrier integrity by regulating the tight-junction integrity. Several in vitro and experimental animal studies have examined the impact of SCFAs on tight junctions. A study using differentiated IEC observed that butyrate improved the gut barrier integrity through the expression increase of the tight-junctions claudin-1.Citation115 An increased expression of other tight-junctions, including claudin-7, ZO-1, ZO-2, occluding and junctional adhesion molecule A (JAMA), was associated with SCFA production in a mice model study.Citation116 Butyrate also influences the epithelial O2 consumption, contributing to the stabilization of transcription factor hypoxia-inducible factor (HIF), which coordinates the gut barrier protection.Citation117 A proper gut barrier integrity is essential to avoid some pathogenic bacteria (e.g., C. pneumoniae, H. pylori, A. actinomycetemcomitans, and P. gingivalis) entering into the bloodstream and reaching different tissues, in which they can promote CMD through immune system elicitation, host metabolic and inflammatory response regulation.Citation118–120 A correct modulation of the mucus layer thickness is also important for the epithelial barrier function. Butyrate can increase the production of MUC2, a predominant mucin glycoprotein secreted by goblet cells.Citation121,Citation122 Finally, SCFAs can promote the antimicrobial peptide secretion by the IEC. For instance, SCFAs promote the RegIIIγ and defensins production by activating mTOR and STAT3, and thus regulating the epithelial barrier functions.Citation123
Energy intake and energy use
SCFAs might present positive effects on body weight control by regulating the energy intake and energy expenditure. Some insights have been obtained into the mechanisms by which SCFAs regulate appetite. A potential mechanism might be the stimulation of secretion of gut-derived satiety hormones, such as peptide YY (PYY) and glucagon-like peptide 1 (GLP-1), by SCFAs binding to the free fatty acid receptor FFAR2 and FFAR3.Citation124 Both hormones, which are secreted by intestinal enteroendocrine L-cells,Citation125 influence appetite by activating proopiomelanocortin (POMC) neurons in the hypothalamic arcuate nucleus, suppressing neuropeptide Y (NPY), and delaying or inhibiting gastric emptying.Citation126–128 Expression of genes encoding PYY is also regulated by receptor-independent pathways. Indeed, the inhibitory activity of HDAC by butyrate leads to an increased PYY expression in human L-cells.Citation129 Besides, a study using in vivoCitation11C-acetate and PET-CT acetate demonstrated that acetate can cross the blood-brain barrier and is taken up by the hypothalamus, causing an appetite decrease and increase of γ-aminobutyric acid and lactate.Citation130 The secretion of leptin, which is often referred to as the “satiety hormone”, might be also stimulated by SCFAs, resulting in a decreased appetite.Citation131,Citation132 For instance, human adipocytes incubated with a high concentration of propionate appeared to increase the leptin mRNA expression and leptin secretion.Citation133
Glucose homeostasis and insulin resistance
Several studies have suggested that SCFAs can improve glucose homeostasis in vivo by controlling blood glucose levels and increasing glucose uptake mediated by FFAR2 and FFAR3 activation.Citation111,Citation112,Citation134 Although the mechanisms are not completely clear, such effects might happen directly via an AMP-activated protein kinase (AMPK)-dependent co-regulated pathway or indirectly via the PPY and GLP-1 hormones. Indeed, Li et al. (2019)Citation135 have reported that butanoate can affect glucose metabolism through the up-regulation of AMPK-dependent gene expression. Another study has shown that propionate declines hepatic gluconeogenesis via the same mechanism.Citation136 Furthermore, apart from the previously commented functions of PYY and GLP-1, PYY can also contribute to the glucose clearance in adipose tissue and muscle, and GLP-1 can increase insulin secretion and decrease glucagon secretion by the pancreas, regulating blood glucose levels.Citation137 At the same time, it seems that SCFAs can exert anti-diabetic effects in the host. Propionate presents benefits on pancreatic β-cell function in vivo, and enhances glucose-stimulated insulin release via FFAR2 activation and increases β-cell mass.Citation134 Besides, the binding of SCFAs to the FFAR2 receptor might ameliorate insulin resistance by promoting autophagy of skeletal muscle cells.Citation138
Lipid metabolism
SCFAs can regulate lipolysis and adipogenesis. Acetate and propionate may inhibit endogenous lipolysis, whereas propionate can regulate extracellular lipolysis mediated by an increase of lipoprotein lipase expression, both cases resulting in a decrease of the circulating lipid plasma levels and body weight.Citation132,Citation139 As well, SCFAs might play an important role in adipogenic differentiation. Indeed, preadipocytes treated with propionate, and acetate promoted adipocyte differentiation, via an overexpression of FFAR2 and PPARγ.Citation140,Citation141 Finally, acetate, propionate, and butyrate seem to enhance hepatic uptake of cholesterol from the blood, decreasing plasma cholesterol in model animal studies.Citation142,Citation143 Besides, propionate is a potent inhibitor of cholesterol synthesis.Citation144
Immune function and anti-inflammatory response
SCFAs play a role in the immune system regulation. Of note, it has been shown that butyrate can inhibit HDAC and the activation of nuclear factor kappa β (NF-κB) in macrophages.Citation145,Citation146 Both, HDAC and NF-κB, contribute to the immune and inflammatory response.Citation147 SCFAs are also involved in anti-inflammatory response by up-regulating anti-inflammatory cytokines and downregulating pro-inflammatory ones. For example, SCFAs binding to FFAR2 and GPR109A in IEC stimulates K+ efflux and hyperpolarization, leading to the inflammasome-activating protein NLRP3 activation, and thus, inducing the IL-18 release, which helps in the maintenance of integrity, repair, and intestinal homeostasis.Citation148,Citation149 Increased protein acetylation and production of TGFβ1 in IEC by butyrate lead to a decrease of IL-8 production in IECCitation150 and a promotion of anti-inflammatory regulatory T cells (Treg),Citation151 respectively. In human mature dendritic cells, butyrate and propionate appear to reduce the release of pro-inflammatory chemokines, such as CXCL11, CXCL10, CXCL9, CCL5, CCL4, and CCL3, just as inhibiting the expression of LPS-induced cytokines, including IL-6 and IL-12p40.Citation152 Apart from the cytokine production regulation, the luminal pH reduction by SCFA inhibits the growth of pathogenic bacteria.Citation153 Lastly, SCFAs, specifically butyrate, can contribute to host defense by inducing the antimicrobial protein cathelicidin IL-37Citation154,Citation155 and increase the levels of T regulatory cells in the gut.Citation156
Taking all this together, we can deduce that SCFAs can exert benefits in CMD, which are characterized by a deregulation of the blood pressure, glucose, and lipid metabolism, inflammation response, and/or gut barrier integrity. Indeed, several studies have demonstrated the benefits exerted by SCFAs in CMD. shows some of these studies.
Table 1. Summary of studies reporting beneficial effects of SCFAs in cardio-metabolic health through different traits
. Summary of studies reporting beneficial effects of SCFAs in cardio-metabolic health through different traits.
Interplay between diet, gut microbial SCFAs, and CMD
Diets rich in fiber and omega-3 exert beneficial effects in CMDCitation167,Citation168 lowering the risk of CVD by about 30%, for example, in the Mediterranean diet.Citation168 The risk decrease is due to the capability of these diets to reduce risk factors associated with CMH, such as blood pressure, cholesterol, body weight, and systematic inflammation.Citation65,Citation108,Citation169,Citation170 Moreover, short- and long-term diets can induce shifts in gut microbiota activity and composition, and thus, in the SCFA synthesis profile.Citation171–173 The interplay between diet, gut bacteria-derived metabolites, specifically SCFAs, and CMD has only been reported recently. depicts some studies in which this interplay is shown. As some authors have suggested,Citation65,Citation108,Citation186 dietary interventions are a low-risk and cheap strategy to modulate the gut microbiota composition, particularly toward an augmented SCFA production providing benefits to CMH.
Table 2. Studies reporting the modulation by diets of SCFA-producing bacteria, SCFA levels, and risk factors associated with cardio-metabolic health
The modulation of SCFA-producing bacteria and/or SCFA levels by three different diets, namely, fiber-rich diets, omega-3 rich diets, and dairy products fermented/supplemented with beneficial bacteria, just as the exerted benefits in CMH are discussed below.
Fiber-rich diets
Inulin, resistant starch, fructo-oligosaccharides, and polysaccharides, among others, present the food label of fiber in the US.Citation187 It has been suggested that fiber-rich diets, such as the Mediterranean and vegetarian diets, can protect against the development of CMD, including CVD, obesity, and T2D, by modulating the gut microbiota.Citation188
Many studies have shown that SCFA concentrations and/or SCFA producing-bacteria can be enhanced by fiber-rich diets. Indeed, a transversal study with 31 healthy individuals showed that the individuals following a 6-month Mediterranean diet presented higher propionate and butyrate concentration in feces, and higher levels of Bifidobacterium and Faecalibacterium compared to those having a lower fiber intake.Citation179 Another study comparing the fecal microbiota between children from a rural African village with European children observed that African children, who have fiber-rich diets, presented significantly more SCFAs and bacteria belonging to the Bacteroidetes phylum.Citation178 More studies in which fiber-rich diets positively influence SCFA production can be found in the review conducted by Dreher (2017).Citation189
Other human and murine studies have reported that dietary fiber can improve the risk factors associated with CMD by modulating the SCFA-producing gut bacteria. For instance, after following a 3-month fiber-rich diet, patients with T2D showed an increase of the SCFA producing-bacteria F. prausnitzii and A. muciniphila, as well as a decrease in glucose, total and LDL cholesterol, free fatty acids and hemoglobin A1c (HbA1c),Citation65 suggesting that a long-term adherence to a high-fiber diet might improve dyslipidemia, glycemic control, and inflammation by increasing SCFA-producing bacteria. In another study, a vegetarian diet assigned to patients with ischemic heart disease caused an increase of the families Ruminococcaceae, Lachnospiraceae, and Akkermansiaceae, and a decrease of LDL and total cholesterol and body weight compared to the patients following a meat diet.Citation169 Therefore, it was suggested that the improvement of these cardiometabolic risk factors might be caused by the capability of these bacterial families to produce SCFAs. Finally, a murine model study showed that Roseburia (butyrate-producing bacterial genus) can interact with dietary plant polysaccharides, promoting fatty acid utilization and reducing systematic inflammation, which turns in the amelioration of atherosclerosis development.Citation174
Omega-3 rich diets
Omega-3 rich diets have been also associated with an increase of SCFA-producing bacteria. Indeed, in a randomized trial, 2-month of treatment with omega-3 polyunsaturated fatty acids (PUFA) capsules or drinks was given to 22 healthy middle-aged subjects to analyze the effect of omega-3 PUFA on the human gut microbiota.Citation66 An increased abundance of SCFA-producing bacteria belonging to the Lachnospira, Roseburia, Lactobacillus, and Bifidobacterium genus was reported in the subjects taken one or both formulations (capsules and drinks). Noriega et colleagues (2016)Citation182 showed a significant increase of the butyrate-producing bacteria Eubacterium, Roseburia, Anaerostipes, Coprococcus, Subdoligranulum, and Pseudobutyrivibrio after 2 weeks of an omega-3 rich diet.
Other studies have also reported this association and suggested the potential beneficial role of this type of diet in CMD. For instance, a recent human study showed a significant increase in Coprococcus spp. and Bacteroides spp., and in isobutyrate and isovalerate levels after a daily supplementation of 500 mg of omega-3 fatty acid for 6 weeks.Citation190 Likewise, Coprococcus was positively associated with the branched-chain fatty acid isobutyric acid and negatively associated with the triglyceride-rich lipoproteins VLDL and VLDL-TG, suggesting that dietary omega-3 influences the gut microbiota composition and its benefits in CVD might be mediated by gut fermentation products.Citation190 In another study, three different diets were fed to female mice, namely control, n-3 PUFA supplemented and n-3 PUFA deficient, during gestation, and then, the male offspring continued with the same diet for 3 months.Citation170 Deficiency of n3-PUFA in diet reduced the levels of acetate and butyrate in feces, and the Clostridiaceae family, which can produce SCFAs, was not detected in this group. Likewise, the metabolic results revealed an increase of cecal metabolites involved in energy metabolism in n-3 PUFA supplemented mice. Thus, it was suggested that the observed impairment of SCFA production might disrupt the metabolism homeostasis and thus, having an impact on metabolic diseases. In a study conducted by Balfegó and coworkers,Citation181 gut bacterial species were determined in drug-naïve patients with T2D before and after following either a standard diet (control) or the same diet but enriched with sardines for 6 months. Individuals following the latter diet presented an increase of Bacteroides and Prevotella (acetate-producing bacteria) in comparison with the baseline. It was indicated that this diet might present benefits for cardiovascular risk.
Dairy products fermented or supplemented with beneficial bacteria
A few studies have reported the capability of dairy products fermented or supplemented with beneficial bacteria to increase SCFA-producing bacteria. Bifidobacterium animalis subsp. lactis fermented milk product resulted in an increase of butyrate-producing bacteria and cecal SCFA in a mouse model, and an inflammation reduction.Citation183 This statement was further validated in humans, where the same fermented milk product potentiated colonic SCFA production and increased two previously uncharacterized butyrate producers, namely MGS126 and MGS203.Citation184 Another study reported that skimmed milk supplemented with Lactobacillus paracasei subsp. Paracasei caused in healthy young adults a significant increase in Lactobacillus, Bifidobacterium, and Roseburia and in the acetic acid and butyrate acid levels, compared with the control (only skimmed milk).Citation185
However, more research is required in the CMD field as no study has focused on investigating the effect of these dairy products in these diseases yet.
. Studies reporting the modulation by diets of SCFA-producing bacteria, SCFA levels, and risk factors associated with cardio-metabolic health.
Conclusions and future directions
The composition, diversity, and activity of the gut microbiota can be easily modified by dietary patterns or specific nutritional components. Indeed, several studies have demonstrated that certain diet types can increase the abundance of SCFA-producing bacteria, leading to an augmented SCFA level. Likewise, SCFAs have shown promising results in the modulation of CMD, as they can improve different risk factors associated to these diseases, such as dyslipidemia, cholesterol, insulin resistance, hyperglycemia, and inflammation, thanks mainly to their capability to inhibit HDAC and activate the different FFAR receptors. However, long-term human intervention studies analyzing the effect of different diets in the SCFA production and the benefits exerted in patients suffering from CMD are clearly needed. These types of studies would contribute to deeply understand the interplay between diet, SCFAs, and CMD, enabling us to design dietary strategies that prevent/improve the CMD development by enhancing the SCFA production. Thus, the major public health challenge that CMD represents might be addressed with these low-risk and cheap strategies. Additionally, a limitation of some human studies is the lack of consistency in their results due to the variability in the baseline microbiota of the participants. Therefore, future work will focus on how to create personalized dietary strategies based on the microbiota of each individual. As well it would be interesting to determine the synergic effect of different diets (e.g., a diet rich in omega-3 and fiber) in the gut microbiota and CMH.
Abbreviations
AMPK, AMP-activated protein kinase; cardio-metabolic diseases; cAMP, cyclic adenosine monophosphate; CMH, cardio-metabolic health; CVD, cardiovascular diseases; FFAR, free fatty acid receptor; GLP-1, glucagon-like peptide 1; GPR, G-protein coupled receptor; HDAC, histone deacetylase; IEC, intestinal epithelial cells; LPS, lipopolysaccharides; NF-κB, nuclear factor kappa β; PUFA, polyunsaturated fatty acids; PYY, peptide YY; PPARγ, peroxisome proliferator-activated receptor γ; SCFA, short-chain fatty acid; TMA, trimethylamine; TMAO, trimethylamine‑N‑oxide; T2D, type-2 diabetes; Olfr72, olfactory receptor-78.
Disclosure statement
Ana M. Valdes is a consultant for Zoe Global Ltd. No potential conflict of interest was reported by the authors.
Additional information
Funding
References
- Rudd KE, Johnson SC, Agesa KM, Shackelford KA, Tsoi D, Kievlan DR, Colombara DV, Ikuta KS, Kissoon N, Finfer S. Global, regional, and national sepsis incidence and mortality, 1990–2017: analysis for the global burden of disease study. The Lancet. 2020;395(10219):200–24. doi:10.1016/S0140-6736(19)32989-7.
- Ounpuu S, Anand S, Yusuf S. The global burden of cardiovascular disease. Medsc Cardiol. 2002;4(1):S5-S13.
- Mathers CD, Loncar D. Projections of global mortality and burden of disease from 2002 to 2030. PLoS Med. 2006;3(11):e442. doi:10.1371/journal.pmed.0030442.
- Woting A, Blaut M. The intestinal microbiota in metabolic disease. Nutrients. 2016;8(4):202. doi:10.3390/nu8040202.
- Meyer KA, Bennett BJ. Diet and gut microbial function in metabolic and cardiovascular disease risk. Current Diabetes Reports. 2016;16(10):93. doi:10.1007/s11892-016-0791-x.
- Goodrich JK, Waters JL, Poole AC, Sutter JL, Koren O, Blekhman R, Beaumont M, Van Treuren W, Knight R, Bell JT. Human genetics shape the gut microbiome. Cell. 2014;159(4):789–799. doi:10.1016/j.cell.2014.09.053.
- Fan Y, Pedersen O. 2020. Gut microbiota in human metabolic health and disease. Nature Reviews Microbiology. 1–17.
- Zubcevic J, Baker A, Martyniuk CJ. Transcriptional networks in rodent models support a role for gut-brain communication in neurogenic hypertension: a review of the evidence. Physiol Genomics. 2017;49(7):327–338. doi:10.1152/physiolgenomics.00010.2017.
- Mashaqi S, Gozal D. Obstructive sleep apnea and systemic hypertension: gut dysbiosis as the mediator?. J Clin Sleep Med. 2019;15(10):1517–1527. doi:10.5664/jcsm.7990.
- Ko CY, Fan JM, Hu AK, Su HZ, Yang JH, Huang LM, Yan FR, Zhang HP, Zeng YM. Disruption of sleep architecture in prevotella enterotype of patients with obstructive sleep apnea-hypopnea syndrome. Brain Behav. 2019;9(5):e01287. doi:10.1002/brb3.1287.
- Fernández-Bello I, Monzón Manzano E, García Río F, Justo Sanz R, Cubillos-Zapata C, Casitas R, Sánchez B, Jaureguizar A, Acuña P, Alonso-Fernández A. 2020. Procoagulant state of sleep apnea depends on systemic inflammation and endothelial damage. Arch Bronconeumol.
- Sekirov I, Russell SL, Antunes LCM, Finlay BB. Gut microbiota in health and disease. Physiol Reviews. 2010;90(3):859–904. doi:10.1152/physrev.00045.2009.
- Korecka A, Arulampalam V. The gut microbiome: scourge, sentinel or spectator?. J Oral Microbiol. 2012;4(1):9367. doi:10.3402/jom.v4i0.9367.
- Brown JM, Hazen SL. Microbial modulation of cardiovascular disease. Nat Reviews Microbiol. 2018;16(3):171–181. doi:10.1038/nrmicro.2017.149.
- Topping DL, Clifton PM. 2001. Short-chain fatty acids and human colonic function: roles leof resistant starch and nonstarch polysaccharides. Physiological reviews.
- Martin-Gallausiaux C, Marinelli L, Blottière HM, Larraufie P, Lapaque N. 2020. SCFA: mechanisms and functional importance in the gut. Proceedings of the Nutrition Society. 1–13.
- Den Besten G, Van Eunen K, Groen AK, Venema K, Reijngoud D-J, Bakker BM. The role of short-chain fatty acids in the interplay between diet, gut microbiota, and host energy metabolism. J Lipid Res. 2013;54(9):2325–2340. doi:10.1194/jlr.R036012.
- Garcia-Mantrana I, Selma-Royo M, Alcantara C, Collado MC. Shifts on gut microbiota associated to mediterranean diet adherence and specific dietary intakes on general adult population. Front Microbiol. 2018;9:890. doi:10.3389/fmicb.2018.00890.
- Costantini L, Molinari R, Farinon B, Merendino N. Impact of omega-3 fatty acids on the gut microbiota. Int J Mol Sci. 2017;18(12):2645. doi:10.3390/ijms18122645.
- Ríos-Covián D, Ruas-Madiedo P, Margolles A, Gueimonde M, De Los Reyes-gavilán CG, Salazar N. Intestinal short chain fatty acids and their link with diet and human health. Front Microbiol. 2016;7:185. doi:10.3389/fmicb.2016.00185.
- Morrison DJ, Preston T. Formation of short chain fatty acids by the gut microbiota and their impact on human metabolism. Gut Microbes. 2016;7(3):189–200. doi:10.1080/19490976.2015.1134082.
- Chambers ES, Preston T, Frost G, Morrison DJ. Role of gut microbiota-generated short-chain fatty acids in metabolic and cardiovascular health. Curr Nutr Rep. 2018;7(4):198–206. doi:10.1007/s13668-018-0248-8.
- Wong JM. Gut microbiota and cardiometabolic outcomes: influence of dietary patterns and their associated components. The Am J Clin Nutr. 2014;100(suppl_1):369S–377S. doi:10.3945/ajcn.113.071639.
- Wan Y, Tang J, Li J, Li J, Yuan J, Wang F, Li D. Contribution of diet to gut microbiota and related host cardiometabolic health: diet-gut interaction in human health. Gut Microbes. 2020;11(3):603–609. doi:10.1080/19490976.2019.1697149.
- Hansen TH, Gøbel RJ, Hansen T, Pedersen O. The gut microbiome in cardio-metabolic health. Genome Med. 2015;7(1):1–16. doi:10.1186/s13073-015-0157-z.
- Savage DC. Microbial ecology of the gastrointestinal tract. Annu Review Microbiol. 1977;3(12):107–133. doi:10.1146/annurev.mi.31.100177.000543.
- Hooper LV, Gordon JI. Commensal host-bacterial relationships in the gut. Sci. 2001;292(5519):1115–1118. doi:10.1126/science.1058709.
- Eckburg PB, Bik EM, Bernstein CN, Purdom E, Dethlefsen L, Sargent M, Gill SR, Nelson KE, Relman DA. Diversity of the human intestinal microbial flora. Sci. 2005;308:1635–1638.
- Rakoff-Nahoum S, Paglino J, Eslami-Varzaneh F, Edberg S, Medzhitov R. Recognition of commensal microflora by toll-like receptors is required for intestinal homeostasis. Cell. 2004;118(2):229–241. doi:10.1016/j.cell.2004.07.002.
- Falony G, Joossens M, Vieira-Silva S, Wang J, Darzi Y, Faust K, Kurilshikov A, Bonder MJ, Valles-Colomer M, Vandeputte D. Population-level analysis of gut microbiome variation. Sci. 2016;352(6285):560–564. doi:10.1126/science.aad3503.
- Malla MA, Dubey A, Kumar A, Yadav S, Hashem A, Abd_Allah EF. Exploring the human microbiome: the potential future role of next-generation sequencing in disease diagnosis and treatment. Front Immunol. 2019;9:2868. doi:10.3389/fimmu.2018.02868.
- Montalto M, D’onofrio F, Gallo A, Cazzato A, Gasbarrini G. Intestinal microbiota and its functions. Digestive Liver Dis Suppl. 2009;3(2):30–34. doi:10.1016/S1594-5804(09)60016-4.
- Iebba V, Totino V, Gagliardi A, Santangelo F, Cacciotti F, Trancassini M, Mancini C, Cicerone C, Corazziari E, Pantanella F. Eubiosis and dysbiosis: the two sides of the microbiota. New Microbiol. 2016;39:1–12.
- Schippa S, Conte MP. Dysbiotic events in gut microbiota: impact on human health. Nutr. 2014;6(12):5786–5805. doi:10.3390/nu6125786.
- Katsimichas T, Antonopoulos AS, Katsimichas A, Ohtani T, Sakata Y, Tousoulis D. The intestinal microbiota and cardiovascular disease. Cardiovasc Res. 2019;115(10):1471–1486. doi:10.1093/cvr/cvz135.
- Tang WW, Hazen SL. Microbiome, trimethylamine N-oxide, and cardiometabolic disease. Transl Res. 2017;179:108–115. doi:10.1016/j.trsl.2016.07.007.
- Ley RE, Turnbaugh PJ, Klein S, Gordon JI. Human gut microbes associated with obesity. Nat. 2006;444(7122):1022–1023. doi:10.1038/4441022a.
- Tanti J-F, Ceppo F, Jager J, Berthou F. Implication of inflammatory signaling pathways in obesity-induced insulin resistance. Front Endocrinol. 2013;3:181. doi:10.3389/fendo.2012.00181.
- Philpott DJ, Sorbara MT, Robertson SJ, Croitoru K, Girardin SE. NOD proteins: regulators of inflammation in health and disease. Nat Reviews Immunol. 2014;14(1):9–23. doi:10.1038/nri3565.
- Curtiss LK, Tobias PS. Emerging role of Toll-like receptors in atherosclerosis. J Lipid Res. 2009;50(Supplement):S340–S345. doi:10.1194/jlr.R800056-JLR200.
- Zhu W, Gregory JC, Org E, Buffa JA, Gupta N, Wang Z, Li L, Fu X, Wu Y, Mehrabian M. Gut microbial metabolite TMAO enhances platelet hyperreactivity and thrombosis risk. Cell. 2016;165(1):111–124. doi:10.1016/j.cell.2016.02.011.
- Wang Z, Roberts AB, Buffa JA, Levison BS, Zhu W, Org E, Gu X, Huang Y, Zamanian-Daryoush M, Culley MK. Non-lethal inhibition of gut microbial trimethylamine production for the treatment of atherosclerosis. Cell. 2015;163(7):1585–1595. doi:10.1016/j.cell.2015.11.055.
- Randrianarisoa E, Lehn-Stefan A, Wang X, Hoene M, Peter A, Heinzmann SS, Zhao X, Königsrainer I, Königsrainer A, Balletshofer B. Relationship of serum trimethylamine N-oxide (TMAO) levels with early atherosclerosis in humans. Scientific Reports. 2016;6(1):1–9. doi:10.1038/srep26745.
- Dambrova M, Latkovskis G, Kuka J, Strele I, Konrade I, Grinberga S, Hartmane D, Pugovics O, Erglis A, Liepinsh E. Diabetes is associated with higher trimethylamine N-oxide plasma levels. Exp Clin Endocrinol Diabetes. 2016;124(04):251–256. doi:10.1055/s-0035-1569330.
- Schugar RC, Shih DM, Warrier M, Helsley RN, Burrows A, Ferguson D, Brown AL, Gromovsky AD, Heine M, Chatterjee A. The TMAO-producing enzyme flavin-containing monooxygenase 3 regulates obesity and the beiging of white adipose tissue. Cell Reports. 2017;19(12):2451–2461. doi:10.1016/j.celrep.2017.05.077.
- Wahlström A, Sayin SI, Marschall H-U, Bäckhed F. Intestinal crosstalk between bile acids and microbiota and its impact on host metabolism. Cell Metab. 2016;24(1):41–50. doi:10.1016/j.cmet.2016.05.005.
- Hylemon PB, Zhou H, Pandak WM, Ren S, Gil G, Dent P. Bile acids as regulatory molecules. J Lipid Res. 2009;50(8):1509–1520. doi:10.1194/jlr.R900007-JLR200.
- Joyce SA, Gahan CG. Disease-associated changes in bile acid profiles and links to altered gut microbiota. Digestive Dis. 2017;35(3):169–177. doi:10.1159/000450907.
- Vítek L. Bile acids in the treatment of cardiometabolic diseases. Annals Hepatol. 2017;16(1):43–52. doi:10.5604/01.3001.0010.5496.
- Masella R, Santangelo C, D’archivio M, LiVolti G, Giovannini C, Galvano F. Protocatechuic acid and human disease prevention: biological activities and molecular mechanisms. Curr Med Chem. 2012;19(18):2901–2917. doi:10.2174/092986712800672102.
- Menni C, Hernandez MM, Vital M, Mohney RP, Spector TD, Valdes AM. Circulating levels of the anti-oxidant indoleproprionic acid are associated with higher gut microbiome diversity. Gut Microbes. 2019;16(10):688–695. doi:10.1080/19490976.2019.1586038.
- Al-Waiz M, Mikov M, Mitchell S, Smith R. The exogenous origin of trimethylamine in the mouse. Metab. 1992;41(2):135–136. doi:10.1016/0026-0495(92)90140-6.
- Zhu W, Buffa J, Wang Z, Warrier M, Schugar R, Shih D, Gupta N, Gregory J, Org E, Fu X. Flavin monooxygenase 3, the host hepatic enzyme in the metaorganismal trimethylamine n‐oxide‐generating pathway, modulates platelet responsiveness and thrombosis risk. J Thrombosis and Haemostasis. 2018;16(9):1857–1872. doi:10.1111/jth.14234.
- Tang WW, Wang Z, Kennedy DJ, Wu Y, Buffa JA, Agatisa-Boyle B, Li XS, Levison BS, Hazen SL. Gut microbiota-dependent trimethylamine n-oxide (TMAO) pathway contributes to both development of renal insufficiency and mortality risk in chronic kidney disease. Circulation Res. 2015;116(3):448–455. doi:10.1161/CIRCRESAHA.116.305360.
- Brown JM, Hazen SL. The gut microbial endocrine organ: bacterially derived signals driving cardiometabolic diseases. Annu Review Med. 2015;66(1):343–359. doi:10.1146/annurev-med-060513-093205.
- Heianza Y, Ma W, Manson JE, Rexrode KM, Qi L. Gut microbiota metabolites and risk of major adverse cardiovascular disease events and death: a systematic review and meta‐analysis of prospective studies. J Am Heart Association. 2017;6(7):e004947. doi:10.1161/JAHA.116.004947.
- Yang S, Li X, Yang F, Zhao R, Pan X, Liang J, Tian L, Li X, Liu L, Xing Y. Gut microbiota-dependent marker TMAO in promoting cardiovascular disease: inflammation mechanism, clinical prognostic, and potential as a therapeutic target. Front Pharmacol. 2019;10. doi:10.3389/fphar.2019.00010.
- Keitel V, Kubitz R, Häussinger D. Endocrine and paracrine role of bile acids. WJG. 2008;14(37):5620. doi:10.3748/wjg.14.5620.
- Fiorucci S, Distrutti E. Bile acid-activated receptors, intestinal microbiota, and the treatment of metabolic disorders. Trends in Molecular Medicine. 2015;21(11):702–714. doi:10.1016/j.molmed.2015.09.001.
- Khurana S, Raufman JP, Pallone TL. Bile acids regulate cardiovascular function. Clinical and Translational Science. 2011;4(3):210–218. doi:10.1111/j.1752-8062.2011.00272.x.
- Aura A-M, Martin-Lopez P, O’Leary KA, Williamson G, Oksman-Caldentey K-M, Poutanen K, Santos-Buelga C. In vitro metabolism of anthocyanins by human gut microflora. European Journal of Nutrition. 2005;44(3):133–142. doi:10.1007/s00394-004-0502-2.
- Dodd D, Spitzer MH, Van Treuren W, Merrill BD, Hryckowian AJ, Higginbottom SK, Le A, Cowan TM, Nolan GP, Fischbach MA. A gut bacterial pathway metabolizes aromatic amino acids into nine circulating metabolites. Nature. 2017;551(7682):648–652. doi:10.1038/nature24661.
- De Mello VD, Paananen J, Lindström J, Lankinen MA, Shi L, Kuusisto J, Pihlajamäki J, Auriola S, Lehtonen M, Rolandsson O. Indolepropionic acid and novel lipid metabolites are associated with a lower risk of type 2 diabetes in the finnish diabetes prevention study. Scientific Reports. 2017;7(1):46337. doi:10.1038/srep46337.
- Sanna S, Van Zuydam NR, Mahajan A, Kurilshikov A, Vich Vila A, Võsa U, Mujagic Z, Masclee AAM, Jonkers D, Oosting M. Causal relationships among the gut microbiome, short-chain fatty acids and metabolic diseases. Nat Genet. 2019;51(4):600–605. doi:10.1038/s41588-019-0350-x.
- Medina-Vera I, Sanchez-Tapia M, Noriega-Lopez L, Granados-Portillo O, Guevara-Cruz M, Flores-López A, Avila-Nava A, Fernández M, Tovar A, Torres N. A dietary intervention with functional foods reduces metabolic endotoxaemia and attenuates biochemical abnormalities by modifying faecal microbiota in people with type 2 diabetes. Diabetes & Metabolism. 2019;45(2):122–131. doi:10.1016/j.diabet.2018.09.004.
- Watson H, Mitra S, Croden FC, Taylor M, Wood HM, Perry SL, Spencer JA, Quirke P, Toogood GJ, Lawton CL. A randomised trial of the effect of omega-3 polyunsaturated fatty acid supplements on the human intestinal microbiota. Gut. 2018;67(11):1974–1983. doi:10.1136/gutjnl-2017-314968.
- Moss G, Smith P, Tavernier D. Glossary of class names of organic compounds and reactivity intermediates based on structure (IUPAC recommendations 1995). Pure and Applied Chemistry. 1995;67(8–9):1307–1375. doi:10.1351/pac199567081307.
- Layden BT, Angueira AR, Brodsky M, Durai V, Lowe JWL. Short chain fatty acids and their receptors: new metabolic targets. Translational Research. 2013;161(3):131–140. doi:10.1016/j.trsl.2012.10.007.
- Richards LB, Li M, Van Esch BC, Garssen J, Folkerts G. The effects of short-chain fatty acids on the cardiovascular system. PharmaNutrition. 2016;4:68–111.
- AsM H, Bjorck IM, Nyman EMG. Combinations of indigestible carbohydrates affect short-chain fatty acid formation in the hindgut of rats. The Journal of Nutrition. 2002;132(10):3098–3104. doi:10.1093/jn/131.10.3098.
- Bergman E. Energy contributions of volatile fatty acids from the gastrointestinal tract in various species. Physiological Reviews. 1990;70(2):567–590. doi:10.1152/physrev.1990.70.2.567.
- Macfarlane J. 1995. Proteolysis and amino acid fermentation. Human colonic bacteria. 75–100.
- Darzi J, Frost GS, Robertson MD. Do SCFA have a role in appetite regulation?. Proceedings of the Nutrition Society. 2011;70(1):119–128. doi:10.1017/S0029665110004039.
- McNeil NI, Cummings J, James W. Short chain fatty acid absorption by the human large intestine. Gut. 1978;19(9):819–822. doi:10.1136/gut.19.9.819.
- Holtug K, Clausen M, Hove H, Christiansen J, Mortensen P. The colon in carbohydrate malabsorption: short-chain fatty acids, pH, and osmotic diarrhoea. Scandinavian Journal of Gastroenterology. 1992;27(7):545–552. doi:10.3109/00365529209000118.
- Cummings J, Pomare E, Branch W, Naylor C, Macfarlane G. Short chain fatty acids in human large intestine, portal, hepatic and venous blood. Gut. 1987;28(10):1221–1227. doi:10.1136/gut.28.10.1221.
- Macfarlane S, Macfarlane GT. Regulation of short-chain fatty acid production. Proceedings of the Nutrition Society. 2003;62(1):67–72. doi:10.1079/PNS2002207.
- Macfarlane G, Gibson G, Cummings J. Comparison of fermentation reactions in different regions of the human colon. J Applied Bacteriology. 1992;72(1):57–64. doi:10.1111/j.1365-2672.1992.tb04882.x.
- Flint HJ, Duncan SH, Scott KP, Louis P. Links between diet, gut microbiota composition and gut metabolism. Proceedings of the Nutrition Society. 2015;74(1):13–22. doi:10.1017/S0029665114001463.
- Miller TL, Wolin MJ. Pathways of acetate, propionate, and butyrate formation by the human fecal microbial flora. Applied and Environmental Microbiology. 1996;62(5):1589–1592. doi:10.1128/AEM.62.5.1589-1592.1996.
- Rey FE, Faith JJ, Bain J, Muehlbauer MJ, Stevens RD, Newgard CB, Gordon JI. Dissecting the in vivo metabolic potential of two human gut acetogens. Journal of Biological Chemistry. 2010;285(29):22082–22090. doi:10.1074/jbc.M110.117713.
- Ragsdale SW, Pierce E. Acetogenesis and the wood–ljungdahl pathway of CO2 fixation. Biochim Biophys Acta Proteins Proteomics. 2008;1784:1873–1898.
- Reichardt N, Duncan SH, Young P, Belenguer A, Leitch CM, Scott KP, Flint HJ, Louis P. Phylogenetic distribution of three pathways for propionate production within the human gut microbiota. The ISME Journal. 2014;8(6):1323–1335. doi:10.1038/ismej.2014.14.
- Macy JM, Ljungdahl LG, Gottschalk G. Pathway of succinate and propionate formation in Bacteroides fragilis. Journal of Bacteriology. 1978;134(1):84–91. doi:10.1128/JB.134.1.84-91.1978.
- Macy JM, Probst I. The biology of gastrointestinal bacteroides. Annual Reviews in Microbiology. 1979;33(1):561–594. doi:10.1146/annurev.mi.33.100179.003021.
- Marchandin H, Teyssier C, Campos J, Jean-Pierre H, Roger F, Gay B, Carlier J-P, Jumas-Bilak E. Negativicoccus succinicivorans gen. Nov., sp. Nov., isolated from human clinical samples, emended description of the family veillonellaceae and description of negativicutes classis nov., selenomonadales ord. Nov. And acidaminococcaceae fam. Nov. in the bacterial phylum firmicutes. International Journal of Systematic and Evolutionary Microbiology. 2010;60(Pt 6):1271–1279. doi:10.1099/ijs.0.013102-0.
- Saxena R, Anand P, Saran S, Isar J, Agarwal L. Microbial production and applications of 1, 2-propanediol. Indian Journal of Microbiology. 2010;50(1):2–11. doi:10.1007/s12088-010-0017-x.
- Bobik TA, Havemann GD, Busch RJ, Williams DS, Aldrich HC. The propanediol utilization (PDU) operon of Salmonella enterica serovar typhimurium lt2 includes genes necessary for formation of polyhedral organelles involved in coenzyme B12-dependent 1, 2-propanediol degradation. Journal of Bacteriology. 1999;181(19):5967–5975. doi:10.1128/JB.181.19.5967-5975.1999.
- Scott KP, Martin JC, Campbell G, Mayer C-D, Flint HJ. Whole-genome transcription profiling reveals genes up-regulated by growth on fucose in the human gut bacterium “Roseburia inulinivorans”. Journal of Bacteriology. 2006;188(12):4340–4349. doi:10.1128/JB.00137-06.
- Belzer C, Chia LW, Aalvink S, Chamlagain B, Piironen V, Knol J, De Vos WM. Microbial metabolic networks at the mucus layer lead to diet-independent butyrate and vitamin B12 production by intestinal symbionts. MBio. 2017;8(5). doi:10.1128/mBio.00770-17.
- Ze X, Le Mougen F, Duncan SH, Louis P, Flint HJ. Some are more equal than others: the role of “keystone” species in the degradation of recalcitrant substrates. Gut Microbes. 2013;4(3):236–240. doi:10.4161/gmic.23998.
- Duncan SH, Louis P, Flint HJ. Lactate-utilizing bacteria, isolated from human feces, that produce butyrate as a major fermentation product. Applied and Environmental Microbiology. 2004;70:5810–5817.
- Louis P, Flint HJ. Diversity, metabolism and microbial ecology of butyrate-producing bacteria from the human large intestine. FEMS Microbiology Letters. 2009;294(1):1–8. doi:10.1111/j.1574-6968.2009.01514.x.
- Duncan SH, Barcenilla A, Stewart CS, Pryde SE, Flint HJ. Acetate utilization and butyryl coenzyme A (CoA): acetate-CoA transferase in butyrate-producing bacteria from the human large intestine. Applied and Environmental Microbiology. 2002;68(10):5186–5190. doi:10.1128/AEM.68.10.5186-5190.2002.
- Louis P, Young P, Holtrop G, Flint HJ. Diversity of human colonic butyrate‐producing bacteria revealed by analysis of the butyryl‐CoA: acetate CoA‐transferase gene. Environmental Microbiology 2010;12(2):304–314. doi:10.1111/j.1462-2920.2009.02066.x.
- Pluznick JL. Gut microbiota in renal physiology: focus on short-chain fatty acids and their receptors. Kidney International. 2016;90(6):1191–1198. doi:10.1016/j.kint.2016.06.033.
- Pluznick JL, Protzko RJ, Gevorgyan H, Peterlin Z, Sipos A, Han J, Brunet I, Wan L-X, Rey F, Wang T. Olfactory receptor responding to gut microbiota-derived signals plays a role in renin secretion and blood pressure regulation. Proceedings of the National Academy of Sciences. 2013;110(11):4410–4415. doi:10.1073/pnas.1215927110.
- Dalile B, Van Oudenhove L, Vervliet B, Verbeke K. 2019. The role of short-chain fatty acids in microbiota–gut–brain communication. Nature Reviews Gastroenterology & Hepatology. 1.
- Milligan G, Stoddart LA, Smith NJ. Agonism and allosterism: the pharmacology of the free fatty acid receptors FFA2 and FFA3. British Journal of Pharmacology. 2009;158(1):146–153. doi:10.1111/j.1476-5381.2009.00421.x.
- Thangaraju M, Cresci GA, Liu K, Ananth S, Gnanaprakasam JP, Browning DD, Mellinger JD, Smith SB, Digby GJ, Lambert NA. GPR109a is a G-protein–coupled receptor for the bacterial fermentation product butyrate and functions as a tumor suppressor in colon. Cancer Research. 2009;69(7):2826–2832. doi:10.1158/0008-5472.CAN-08-4466.
- Donohoe DR, Collins LB, Wali A, Bigler R, Sun W, Bultman SJ. The warburg effect dictates the mechanism of butyrate-mediated histone acetylation and cell proliferation. Molecular Cell. 2012;48(4):612–626. doi:10.1016/j.molcel.2012.08.033.
- Bose P, Dai Y, Grant S. Histone deacetylase inhibitor (hdaci) mechanisms of action: emerging insights. Pharmacol Ther. 2014;143:323–336.
- Alex S, Lange K, Amolo T, Grinstead JS, Haakonsson AK, Szalowska E, Koppen A, Mudde K, Haenen D, Roelofsen H. Short-chain fatty acids stimulate angiopoietin-like 4 synthesis in human colon adenocarcinoma cells by activating peroxisome proliferator-activated receptor γ. Molecular and Cellular Biology. 2013;33(7):1303–1316. doi:10.1128/MCB.00858-12.
- Marinelli L, Martin-Gallausiaux C, Bourhis J-M, Béguet-Crespel F, Blottière HM, Lapaque N. Identification of the novel role of butyrate as ahr ligand in human intestinal epithelial cells. Scientific Reports. 2019;9(1):1–14. doi:10.1038/s41598-018-37019-2.
- Huart J, Leenders J, Taminiau B, Descy J, Saint-Remy A, Daube G, Krzesinski J-M, Melin P, De Tullio P, Jouret F. Gut microbiota and fecal levels of short-chain fatty acids differ upon 24-hour blood pressure levels in men. Hypertension. 2019;74(4):1005–1013. doi:10.1161/HYPERTENSIONAHA.118.12588.
- Pluznick JL. Microbial short-chain fatty acids and blood pressure regulation. Current Hypertension Reports. 2017;19(4):25. doi:10.1007/s11906-017-0722-5.
- Natarajan N, Hori D, Flavahan S, Steppan J, Flavahan NA, Berkowitz DE, Pluznick JL. Microbial short chain fatty acid metabolites lower blood pressure via endothelial G protein-coupled receptor 41. Physiological Genomics. 2016;48(11):826–834. doi:10.1152/physiolgenomics.00089.2016.
- Marques FZ, Nelson E, Chu P-Y, Horlock D, Fiedler A, Ziemann M, Tan JK, Kuruppu S, Rajapakse NW, El-Osta A. High-fiber diet and acetate supplementation change the gut microbiota and prevent the development of hypertension and heart failure in hypertensive mice. Circulation. 2017;135(10):964–977. doi:10.1161/CIRCULATIONAHA.116.024545.
- Miyamoto J, Kasubuchi M, Nakajima A, Irie J, Itoh H, Kimura I. The role of short-chain fatty acid on blood pressure regulation. Current Opinion in Nephrology and Hypertension. 2016;25(5):379–383. doi:10.1097/MNH.0000000000000246.
- Sun D, Zhou T, Heianza Y, Li X, Fan M, Fonseca VA, Qi L. Type 2 diabetes and hypertension: a study on bidirectional causality. Circulation Research. 2019;124(6):930–937. doi:10.1161/CIRCRESAHA.118.314487.
- Canfora EE, Jocken JW, Blaak EE. Short-chain fatty acids in control of body weight and insulin sensitivity. Nature Reviews Endocrinology. 2015;11(10):577. doi:10.1038/nrendo.2015.128.
- Zadeh-Tahmasebi M, Duca FA, Rasmussen BA, Bauer PV, Côté CD, Filippi BM, Lam TK. Activation of short and long chain fatty acid sensing machinery in the ileum lowers glucose production in vivo. Journal of Biological Chemistry. 2016;291(16):8816–8824. doi:10.1074/jbc.M116.718460.
- Velazquez OC, Lederer HM, Rombeau JL. 1997. Butyrate and the colonocyte. Dietary fiber in health and disease. Springer. p. 123–134.
- Lupton JR. Microbial degradation products influence colon cancer risk: the butyrate controversy. The Journal of Nutrition. 2004;134(2):479–482. doi:10.1093/jn/134.2.479.
- Wang H, Shi P, Zuo L, Dong J, Zhao J, Liu Q, Zhu W. Dietary non-digestible polysaccharides ameliorate intestinal epithelial barrier dysfunction in IL-10 knockout mice. Journal of Crohn’s and Colitis. 2016;10(9):1076–1086. doi:10.1093/ecco-jcc/jjw065.
- Hung TV, Suzuki T. Dietary fermentable fibers attenuate chronic kidney disease in mice by protecting the intestinal barrier. The Journal of Nutrition. 2018;148(4):552–561. doi:10.1093/jn/nxy008.
- Kelly CJ, Zheng L, Campbell EL, Saeedi B, Scholz CC, Bayless AJ, Wilson KE, Glover LE, Kominsky DJ, Magnuson A. Crosstalk between microbiota-derived short-chain fatty acids and intestinal epithelial HIF augments tissue barrier function. Cell Host & Microbe. 2015;17(5):662–671. doi:10.1016/j.chom.2015.03.005.
- Munford RS. Endotoxemia—menace, marker, or mistake?. Journal of Leukocyte Biology. 2016;100(4):687–698. doi:10.1189/jlb.3RU0316-151R.
- Filardo S, Di Pietro M, Farcomeni A, Schiavoni G, Sessa R. 2015. Chlamydia pneumoniae-mediated inflammation in atherosclerosis: a meta-analysis. Mediators of inflammation. doi:10.1155/2015/378658.
- Koren O, Spor A, Felin J, Fåk F, Stombaugh J, Tremaroli V, Behre CJ, Knight R, Fagerberg B, Ley RE. Human oral, gut, and plaque microbiota in patients with atherosclerosis. Proceedings of the National Academy of Sciences. 2011;108(Supplement_1):4592–4598. doi:10.1073/pnas.1011383107.
- Hamer HM, Jonkers D, Venema K, Vanhoutvin S, Troost F, Brummer RJ. The role of butyrate on colonic function. Alimentary Pharmacology & Therapeutics. 2008;27(2):104–119. doi:10.1111/j.1365-2036.2007.03562.x.
- Gaudier E, Jarry A, Blottiere H, De Coppet P, Buisine M, Aubert J, Laboisse C, Cherbut C, Hoebler C. Butyrate specifically modulates MUC gene expression in intestinal epithelial goblet cells deprived of glucose. Am J Physiol Gastrointestinal Liver Physiol. 2004;287(6):G1168–G1174. doi:10.1152/ajpgi.00219.2004.
- Zhao Y, Chen F, Wu W, Sun M, Bilotta AJ, Yao S, Xiao Y, Huang X, Eaves-Pyles TD, Golovko G. GPR43 mediates microbiota metabolite SCFA regulation of antimicrobial peptide expression in intestinal epithelial cells via activation of mTOR and STAT3. Mucosal Immunology. 2018;11(3):752–762. doi:10.1038/mi.2017.118.
- Puddu A, Sanguineti R, Montecucco F, Viviani GL. 2014. Evidence for the gut microbiota short-chain fatty acids as key pathophysiological molecules improving diabetes. Mediators of Inflammation.
- Theodorakis MJ, Carlson O, Michopoulos S, Doyle ME, Juhaszova M, Petraki K, Egan JM. Human duodenal enteroendocrine cells: source of both incretin peptides, GLP-1 and GIP. American Journal of Physiology-Endocrinology and Metabolism. 2006;290(3):E550–E559. doi:10.1152/ajpendo.00326.2004.
- De Silva A, Bloom SR. Gut hormones and appetite control: a focus on PYY and GLP-1 as therapeutic targets in obesity. Gut and Liver. 2012;6(1):10. doi:10.5009/gnl.2012.6.1.10.
- Savage A, Adrian T, Carolan G, Chatterjee V, Bloom S. Effects of peptide YY (PYY) on mouth to caecum intestinal transit time and on the rate of gastric emptying in healthy volunteers. Gut. 1987;28(2):166–170. doi:10.1136/gut.28.2.166.
- Naslund E, Bogefors J, Skogar S, Gryback P, Jacobsson H, Holst JJ, Hellstrom PM. GLP-1 slows solid gastric emptying and inhibits insulin, glucagon, and PYY release in humans. American Journal of Physiology-Regulatory, Integrative and Comparative Physiology. 1999;277(3):R910–R916. doi:10.1152/ajpregu.1999.277.3.R910.
- Larraufie P, Martin-Gallausiaux C, Lapaque N, Dore J, Gribble F, Reimann F, Blottiere H. SCFAs strongly stimulate pyy production in human enteroendocrine cells. Scientific Reports. 2018;8(1):1–9. doi:10.1038/s41598-017-18259-0.
- Frost G, Sleeth ML, Sahuri-Arisoylu M, Lizarbe B, Cerdan S, Brody L, Anastasovska J, Ghourab S, Hankir M, Zhang S. The short-chain fatty acid acetate reduces appetite via a central homeostatic mechanism. Nature Communications. 2014;5(1):1–11. doi:10.1038/ncomms4611.
- Soliman M, Kimura K, Ahmed M, Yamaji D, Matsushita Y, Okamatsu-Ogura Y, Makondo K, Saito M. Inverse regulation of leptin mRNA expression by short-and long-chain fatty acids in cultured bovine adipocytes. Domestic Animal Endocrinology. 2007;33(4):400–409. doi:10.1016/j.domaniend.2006.08.005.
- Lee S, Hossner K. Coordinate regulation of ovine adipose tissue gene expression by propionate. Journal of Animal Science. 2002;80(11):2840–2849. doi:10.2527/2002.80112840x.
- Al-Lahham SH, Roelofsen H, Priebe M, Weening D, Dijkstra M, Hoek A, Rezaee F, Venema K, Vonk RJ. Regulation of adipokine production in human adipose tissue by propionic acid. Eur J Clin Invest. 2010;40(5):401–407. doi:10.1111/j.1365-2362.2010.02278.x.
- Pingitore A, Chambers ES, Hill T, Maldonado IR, Liu B, Bewick G, Morrison DJ, Preston T, Wallis GA, Tedford C. The diet‐derived short chain fatty acid propionate improves beta‐cell function in humans and stimulates insulin secretion from human islets in vitro. Diabetes, Obesity and Metabolism. 2017;19(2):257–265. doi:10.1111/dom.12811.
- Li Q, Chen H, Zhang M, Wu T, Liu R. Altered short chain fatty acid profiles induced by dietary fiber intervention regulate ampk levels and intestinal homeostasis. Food & Function. 2019;10(11):7174–7187. doi:10.1039/C9FO01465A.
- Yoshida H, Ishii M, Akagawa M. Propionate suppresses hepatic gluconeogenesis via GPR43/AMPK signaling pathway. Archives of Biochemistry and Biophysics. 2019;672:108057. doi:10.1016/j.abb.2019.07.022.
- Chambers ES, Viardot A, Psichas A, Morrison DJ, Murphy KG, Zac-Varghese SE, MacDougall K, Preston T, Tedford C, Finlayson GS. Effects of targeted delivery of propionate to the human colon on appetite regulation, body weight maintenance and adiposity in overweight adults. Gut. 2015;64(11):1744–1754. doi:10.1136/gutjnl-2014-307913.
- Yang L, Lin H, Lin W, Xu X. Exercise ameliorates insulin resistance of type 2 diabetes through motivating short-chain fatty acid-mediated skeletal muscle cell autophagy. Biology. 2020;9(8):203. doi:10.3390/biology9080203.
- Sa A, Roelofsen H, Rezaee F, Weening D, Hoek A, Vonk R, Venema K. Propionic acid affects immune status and metabolism in adipose tissue from overweight subjects. European Journal of Clinical Investigation. 2012;42(4):357–364. doi:10.1111/j.1365-2362.2011.02590.x.
- Li G, Yao W, Jiang H. Short-chain fatty acids enhance adipocyte differentiation in the stromal vascular fraction of porcine adipose tissue. The Journal of Nutrition. 2014;144(12):1887–1895. doi:10.3945/jn.114.198531.
- Hong Y-H, Nishimura Y, Hishikawa D, Tsuzuki H, Miyahara H, Gotoh C, Choi K-C, Feng DD, Chen C, Lee H-G. Acetate and propionate short chain fatty acids stimulate adipogenesis via GPCR43. Endocrinology. 2005;146(12):5092–5099. doi:10.1210/en.2005-0545.
- Zhao Y, Liu J, Hao W, Zhu H, Liang N, He Z, Ma KY, Chen Z-Y. Structure-specific effects of short-chain fatty acids on plasma cholesterol concentration in male Syrian hamsters. Journal of Agricultural and Food Chemistry. 2017;65(50):10984–10992. doi:10.1021/acs.jafc.7b04666.
- Nguyen TD, Prykhodko O, Hållenius FF, Nyman M. Monobutyrin reduces liver cholesterol and improves intestinal barrier function in rats fed high-fat diets. Nutrients. 2019;11(2):308. doi:10.3390/nu11020308.
- Demigné C, Morand C, Levrat M-A, Besson C, Moundras C, Rémésy C. Effect of propionate on fatty acid and cholesterol synthesis and on acetate metabolism in isolated rat hepatocytes. British Journal of Nutrition. 1995;74(2):209–219. doi:10.1079/BJN19950124.
- Lührs H, Gerke T, Müller J, Melcher R, Schauber J, Boxberger F, Scheppach W, Menzel T. Butyrate inhibits NF-κB activation in lamina propria macrophages of patients with ulcerative colitis. Scandinavian Journal of Gastroenterology. 2002;37(4):458–466. doi:10.1080/003655202317316105.
- Maeda T, Towatari M, Kosugi H, Saito H. Up-regulation of costimulatory/adhesion molecules by histone deacetylase inhibitors in acute myeloid leukemia cells. Blood, the Journal of the American Society of Hematology. 2000;96:3847–3856.
- Glauben R, Siegmund B. Inhibition of histone deacetylases in inflammatory bowel diseases. Molecular Medicine. 2011;17(5–6):426–433. doi:10.2119/molmed.2011.00069.
- Zaki MH, Boyd KL, Vogel P, Kastan MB, Lamkanfi M, Kanneganti T-D. The nlrp3 inflammasome protects against loss of epithelial integrity and mortality during experimental colitis. Immunity. 2010;32(3):379–391. doi:10.1016/j.immuni.2010.03.003.
- Macia L, Tan J, Vieira AT, Leach K, Stanley D, Luong S, Maruya M, McKenzie CI, Hijikata A, Wong C. Metabolite-sensing receptors GPR43 and GPR109a facilitate dietary fibre-induced gut homeostasis through regulation of the inflammasome. Nature Communications. 2015;6(1):6734. doi:10.1038/ncomms7734.
- Huang N, Katz JP, Martin DR, Wu GD. Inhibition of IL-8 gene expression in Caco-2 cells by compounds which induce histone hyperacetylation. Cytokine. 1997;9(1):27–36. doi:10.1006/cyto.1996.0132.
- Atarashi K, Tanoue T, Oshima K, Suda W, Nagano Y, Nishikawa H, Fukuda S, Saito T, Narushima S, Hase K. T reg induction by a rationally selected mixture of clostridia strains from the human microbiota. Nature. 2013;500(7461):232–236. doi:10.1038/nature12331.
- Nastasi C, Candela M, Bonefeld CM, Geisler C, Hansen M, Krejsgaard T, Biagi E, Andersen MH, Brigidi P, Ødum N. The effect of short-chain fatty acids on human monocyte-derived dendritic cells. Scientific Reports. 2015;5(1):1–10. doi:10.1038/srep16148.
- Slavin J. Fiber and prebiotics: mechanisms and health benefits. Nutrients. 2013;5(4):1417–1435. doi:10.3390/nu5041417.
- Cobo ER, Kissoon-Singh V, Moreau F, Holani R, Chadee K. MUC2 mucin and butyrate contribute to the synthesis of the antimicrobial peptide cathelicidin in response to entamoeba histolytica-and dextran sodium sulfate-induced colitis. Infection and Immunity. 2017;85(3). doi:10.1128/IAI.00905-16.
- Schauber J, Dorschner RA, Yamasaki K, Brouha B, Gallo RL. Control of the innate epithelial antimicrobial response is cell‐type specific and dependent on relevant microenvironmental stimuli. Immunology. 2006;118(4):509–519. doi:10.1111/j.1365-2567.2006.02399.x.
- Furusawa Y, Obata Y, Fukuda S, Endo TA, Nakato G, Takahashi D, Nakanishi Y, Uetake C, Kato K, Kato T. Commensal microbe-derived butyrate induces the differentiation of colonic regulatory T cells. Nature. 2013;504(7480):446–450. doi:10.1038/nature12721.
- Li M, van Esch BC, Henricks PA, Folkerts G, Garssen J. 2018. The anti-inflammatory effects of short chain fatty acids on lipopolysaccharide-or tumor necrosis factor α-stimulated endothelial cells via activation of GPR41/43 and inhibition of HDACs. Frontiers in pharmacology. 9:533.
- Bartolomaeus H, Balogh A, Yakoub M, Homann S, Markó L, Höges S, Tsvetkov D, Krannich A, Wundersitz S, Avery EG. Short-chain fatty acid propionate protects from hypertensive cardiovascular damage. Circulation. 2019;139(11):1407–1421. doi:10.1161/CIRCULATIONAHA.118.036652.
- Kang C, Wang B, Kaliannan K, Wang X, Lang H, Hui S, Huang L, Zhang Y, Zhou M, Chen M. Gut microbiota mediates the protective effects of dietary capsaicin against chronic low-grade inflammation and associated obesity induced by high-fat diet. MBio. 2017;8(3):e00470-17.
- Cani PD, Neyrinck AM, Fava F, Knauf C, Burcelin RG, Tuohy KM, Gibson G, Delzenne NM. Selective increases of bifidobacteria in gut microflora improve high-fat-diet-induced diabetes in mice through a mechanism associated with endotoxaemia. Diabetologia. 2007;50(11):2374–2383. doi:10.1007/s00125-007-0791-0.
- Dao MC, Everard A, Aron-Wisnewsky J, Sokolovska N, Prifti E, Verger EO, Kayser BD, Levenez F, Chilloux J, Hoyles L. Akkermansia muciniphila and improved metabolic health during a dietary intervention in obesity: relationship with gut microbiome richness and ecology. Gut. 2016;65(3):426–436. doi:10.1136/gutjnl-2014-308778.
- Kimura I, Ozawa K, Inoue D, Imamura T, Kimura K, Maeda T, Terasawa K, Kashihara D, Hirano K, Tani T. The gut microbiota suppresses insulin-mediated fat accumulation via the short-chain fatty acid receptor GPR43. Nature Communications. 2013;4(1):1–12. doi:10.1038/ncomms2852.
- Lin HV, Frassetto A, Kowalik JEJ, Nawrocki AR, Lu MM, Kosinski JR, Hubert JA, Szeto D, Yao X, Forrest G. Butyrate and propionate protect against diet-induced obesity and regulate gut hormones via free fatty acid receptor 3-independent mechanisms. PloS One. 2012;7(4):e35240. doi:10.1371/journal.pone.0035240.
- Gao Z, Yin J, Zhang J, Ward RE, Martin RJ, Lefevre M, Cefalu WT, Ye J. Butyrate improves insulin sensitivity and increases energy expenditure in mice. Diabetes. 2009;58(7):1509–1517. doi:10.2337/db08-1637.
- Sakakibara S, Yamauchi T, Oshima Y, Tsukamoto Y, Kadowaki T. Acetic acid activates hepatic AMPK and reduces hyperglycemia in diabetic kk-a (y) mice. Biochemical and Biophysical Research Communications. 2006;344(2):597–604. doi:10.1016/j.bbrc.2006.03.176.
- Roshanravan N, Mahdavi R, Alizadeh E, Jafarabadi MA, Hedayati M, Ghavami A, Alipour S, Alamdari NM, Barati M, Ostadrahimi A. Effect of butyrate and inulin supplementation on glycemic status, lipid profile and glucagon-like peptide 1 level in patients with type 2 diabetes: a randomized double-blind, placebo-controlled trial. Hormone and Metabolic Research. 2017;49(11):886–891. doi:10.1055/s-0043-119089.
- Liu S-H, Chiu C-Y, Wang L-P, Chiang M-T. Omega-3 fatty acids-enriched fish oil activates AMPK/PGC-1α signaling and prevents obesity-related skeletal muscle wasting. Marine Drugs. 2019;17(6):380. doi:10.3390/md17060380.
- Estruch R, Ros E, Salas-Salvadó J, Covas M-I, Corella D, Arós F, Gómez-Gracia E, Ruiz-Gutiérrez V, Fiol M, Lapetra J. Primary prevention of cardiovascular disease with a mediterranean diet. New England Journal of Medicine. 2013;368(14):1279–1290. doi:10.1056/NEJMoa1200303.
- Djekic D, Shi L, Brolin H, Carlsson F, Särnqvist C, Savolainen O, Cao Y, Bäckhed F, Tremaroli V, Landberg R. Effects of a vegetarian diet on cardiometabolic risk factors, gut microbiota, and plasma metabolome in subjects with ischemic heart disease: a randomized, crossover study. Journal of the American Heart Association. 2020;9(18):e016518. doi:10.1161/JAHA.120.016518.
- Robertson RC, Oriach CS, Murphy K, Moloney GM, Cryan JF, Dinan TG, Ross RP, Stanton C. Deficiency of essential dietary n-3 PUFA disrupts the caecal microbiome and metabolome in mice. British Journal of Nutrition. 2017;118(11):959–970. doi:10.1017/S0007114517002999.
- Brüssow H, Parkinson SJ. You are what you eat. Nature Biotechnology. 2014;32(3):243–245. doi:10.1038/nbt.2845.
- Wu GD, Chen J, Hoffmann C, Bittinger K, Chen -Y-Y, Keilbaugh SA, Bewtra M, Knights D, Walters WA, Knight R. Linking long-term dietary patterns with gut microbial enterotypes. Science. 2011;334(6052):105–108. doi:10.1126/science.1208344.
- David LA, Maurice CF, Carmody RN, Gootenberg DB, Button JE, Wolfe BE, Ling AV, Devlin AS, Varma Y, Fischbach MA. Diet rapidly and reproducibly alters the human gut microbiome. Nature. 2014;505(7484):559–563. doi:10.1038/nature12820.
- Kasahara K, Krautkramer KA, Org E, Romano KA, Kerby RL, Vivas EI, Mehrabian M, Denu JM, Bäckhed F, Lusis AJ. Interactions between roseburia intestinalis and diet modulate atherogenesis in a murine model. Nature Microbiology. 2018;3(12):1461–1471. doi:10.1038/s41564-018-0272-x.
- Zhao L, Zhang F, Ding X, Wu G, Lam YY, Wang X, Fu H, Xue X, Lu C, Ma J. Gut bacteria selectively promoted by dietary fibers alleviate type 2 diabetes. Science. 2018;359:1151–1156.
- Weitkunat K, Schumann S, Petzke KJ, Blaut M, Loh G, Klaus S. Effects of dietary inulin on bacterial growth, short-chain fatty acid production and hepatic lipid metabolism in gnotobiotic mice. The Journal of Nutritional Biochemistry. 2015;26(9):929–937. doi:10.1016/j.jnutbio.2015.03.010.
- Kim MS, Hwang SS, Park EJ, Bae JW. Strict vegetarian diet improves the risk factors associated with metabolic diseases by modulating gut microbiota and reducing intestinal inflammation. Environmental Microbiology Reports. 2013;5(5):765–775. doi:10.1111/1758-2229.12079.
- De Filippo C, Cavalieri D, Di Paola M, Ramazzotti M, Poullet JB, Massart S, Collini S, Pieraccini G, Lionetti P. Impact of diet in shaping gut microbiota revealed by a comparative study in children from Europe and rural Africa. Proceedings of the National Academy of Sciences. 2010;107(33):14691–14696. doi:10.1073/pnas.1005963107.
- Gutiérrez-Díaz I, Fernández-Navarro T, Sánchez B, Margolles A, González S. Mediterranean diet and faecal microbiota: a transversal study. Food & Function. 2016;7(5):2347–2356. doi:10.1039/C6FO00105J.
- Menni C, Zierer J, Pallister T, Jackson MA, Long T, Mohney RP, Steves CJ, Spector TD, Valdes AM. Omega-3 fatty acids correlate with gut microbiome diversity and production of n-carbamylglutamate in middle aged and elderly women. Scientific Reports. 2017;7(1):1–11. doi:10.1038/s41598-017-10382-2.
- Balfegó M, Canivell S, Hanzu FA, Sala-Vila A, Martínez-Medina M, Murillo S, Mur T, Ruano EG, Linares F, Porras N. Effects of sardine-enriched diet on metabolic control, inflammation and gut microbiota in drug-naïve patients with type 2 diabetes: a pilot randomized trial. Lipids in Health and Disease. 2016;15(1):78. doi:10.1186/s12944-016-0245-0.
- Noriega BS, Sanchez-Gonzalez MA, Salyakina D, Coffman J. 2016. Understanding the impact of omega-3 rich diet on the gut microbiota. Case reports in medicine. doi:10.1155/2016/3089303.
- Veiga P, Gallini CA, Beal C, Michaud M, Delaney ML, DuBois A, Khlebnikov A, Van Hylckama Vlieg JE, Punit S, Glickman JN. 2010. Bifidobacterium animalis subsp. lactis fermented milk product reduces inflammation by altering a niche for colitogenic microbes. Proceedings of the National Academy of Sciences. 107( 42):18132–18137.
- Veiga P, Pons N, Agrawal A, Oozeer R, Guyonnet D, Brazeilles R, Faurie J-M, Van Hylckama Vlieg JE, Houghton LA, Whorwell PJ. Changes of the human gut microbiome induced by a fermented milk product. Scientific Reports. 2015;4(1):6328. doi:10.1038/srep06328.
- Zhang H, Sun J, Liu X, Hong C, Zhu Y, Liu A, Li S, Guo H, Ren F. Lactobacillus paracasei subsp. paracasei lc01 positively modulates intestinal microflora in healthy young adults. Journal of Microbiology. 2013;51(6):777–782. doi:10.1007/s12275-013-3279-2.
- Jayachandran M, Chung SSM, Xu B. A critical review on diet-induced microbiota changes and cardiovascular diseases. Critical Reviews in Food Science and Nutrition. 2020;60(17):2914–2925. doi:10.1080/10408398.2019.1666792.
- Jones JM. Codex-aligned dietary fiber definitions help to bridge the ‘fiber gap’. Nutrition Journal. 2014;13(1):34. doi:10.1186/1475-2891-13-34.
- Slavin JL. Dietary fiber: classification, chemical analyses, and food sources. Journal of the American Dietetic Association. 1987;87:1164–1171.
- Dreher ML. Connection between fiber, colonic microbiota, and health across the human life cycle. Dietary Fiber in Health and Disease. Springer. 2018;67–93.
- Vijay A, Astbury S, Le Roy C, Spector TD, Valdes AM. The prebiotic effects of omega-3 fatty acid supplementation: a six-week randomised intervention trial. Gut Microbes. 2021;13(1):1–11. doi:10.1080/19490976.2020.1863133.