ABSTRACT
Farnesoid X receptor (FXR) is a nuclear receptor for bile acids (BAs) that is widely expressed in the intestine, liver and kidney. FXR has important regulatory impacts on a wide variety of metabolic pathways (such as glucose, lipid, and sterol metabolism) and has been recognized to ameliorate obesity, liver damage, cholestasis and chronic inflammatory diseases. The types of BAs are complex and diverse. BAs link the intestine with the liver through the enterohepatic circulation. BAs derivatives have entered clinical trials for liver disease. In addition to the liver, the intestine is also targeted by BAs. This article reviews the effects of different BAs on the intestinal tract through the enterohepatic circulation from the perspective of FXR, aiming to elucidate the effects of different BAs on the intestinal tract and lay a foundation for new treatment methods.
Introduction
Bile acids (BAs) are important signaling molecules involved in glucose metabolism, lipid metabolism and energy consumption.Citation1–3 BAs dysregulation and impaired BAs receptor transduction are associated with liver and intestinal diseases, such as steatohepatitis, hepatocellular carcinoma, enteritis and colorectal cancer.Citation4–6 In 1999, BAs were discovered to be endogenous ligands of farnesoid X receptor (FXR),Citation7,Citation8 which is widely expressed in the intestine, liver, and kidney.Citation9,Citation10 BAs are generated in the liver and stored in the gallbladder. Postprandially, BAs are secreted into the intestine and metabolized by the intestinal flora. However, 95% of the BAs are reabsorbed in the ileum and returns to the liver for entry into the enterohepatic circulation. Another 5% of BAs are excreted in feces.Citation11,Citation12 BAs can maintain their own synthesis, metabolism and homeostasis by regulating FXR and related pathways.Citation13
BAs have different chemical structures, and the conversion between these chemical structures are mediated primarily by the conjugation of amino acids and the metabolism by microorganisms. The four known mechanisms of microbial BA metabolism are dehydroxylation, dehydration and epimerization of cholesterol, and deconjugation of amino acids.Citation14,Citation15 A recently published study systematically analyzed the effects of microorganisms on the mouse metabolome using mass spectrometry analysis and data visualization methods. Researchers have discovered a new type of amino acid-conjugated BA, which represents a fifth and completely different mechanism of microbial-mediated BA transformation: amide conjugation of the cholate backbone with amino acids phenylalanine, tyrosine and leucine. Moreover, researchers confirmed that the resulting BAs are also present in humans and are more common in patients with inflammatory bowel disease and cystic fibrosis. Cells and mouse experiments have shown that these BAs may affect host physiology by acting on the FXR pathway.Citation16 The latest research has revealed the signaling pathway of gut microbe-mediated cholic acid (CA) dehydroxylation. CA can be used to induce of 7α- dehydroxylation, through which it can be converted into deoxycholic acid (DCA) over time in the presence of a mixture of 8 Bai enzymes, β-nicotinamide adenine dinucleotide (NAD+), coenzyme A and ATP,Citation17 without the requirement for additional enzymes. This process provides possible opportunities for controlling BA metabolism and synthesizing related metabolic molecules. Studies have shown that the BA composition varies across species. The main BAs in human bile are CA (30%-40%), DCA (20%-30%) and chenodeoxycholic acid (CDCA) (30%-40%).Citation18,Citation19 In mice, the main BAs are CA and MCA. In rodents, DCA produced by dehydroxylation in the intestine can be rehydroxylated to generate CA. However, rehydroxylate does not occur in humans; therefore, humans have higher levels of secondary BAs such as DCA.Citation20 BAs are not present in invertebrates but are present in all vertebrates.Citation21 The conjugation of BAs varies greatly among species. BAs in mice are mainly conjugated with taurine, while those in humans are conjugated with glycine and taurine at a ratio of 5:1.Citation20
Currently, some BAs have been used in clinical treatment, usually for hepatobiliary diseases.Citation22 A highly effective FXR agonist, 6-ethylchenodeoxycholic acid (6-ECDCA), was recently highlighted for the treatment of primary biliary cholangitis (PBC),Citation23 and is in late-stage clinical trials for nonalcoholic steatohepatitis (NASH).Citation24–27 Obeticholic acid (OCA) is an agonist of FXR.Citation28 Clinical trials have shown that OCA can improve the characteristics of liver tissue, and OCA is the only investigational drug for NASH that has been awarded the designation of breakthrough treatment.Citation29 But the long-term efficacy and safety still need to be further confirmed. OCA is still approved to treat PBC that does not respond to UDCA.Citation30,Citation31 Itching has also been observed in some patients with PBC under treatment with OCA, although the mechanism is unclear. In some PBC patients with obvious liver damage, taking OCA that exceeds the prescription can cause further deterioration of liver function and death, suggesting that the liver toxicity of FXR agonists should not be ignored. Some researchers have speculated whether targeted intestinal transport of BAs or FXR antagonism could improve the therapeutic.Citation31 Recently, some researchers have discovered a new mechanism for OCA to treat NASH by inhibiting the activity of NLRP3 inflammasome in macrophage.Citation32 These findings indicated that liver FXR is a viable therapeutic target for the development of drugs to treat human liver diseases; however, the potential of FXR as a drug target for intestinal diseases remains to be explored, although its prospects are bright. The intestine is a complex ecosystem and intestinal dysfunction is a serious threat to the health of the host. Generally, intestinal dysfunction is related to inflammatory bowel disease, irritable bowel syndrome, necrotizing enterocolitis, and even intestinal cancer. BAs are important emulsifiers that facilitate the absorption of fats and fat-soluble vitamins. Therefore, they are called “cleanser” of the intestine. Studies have demonstrated that FXR is a nuclear receptor that plays an important role in maintaining BA metabolism and lipid and sugar homeostasis.Citation33,Citation34 Moreover, FXR is an important target for treatments related to BA homeostasis. Recently, new functions of FXR have been discovered, including intestinal barrier protection, regulation of innate immunity and regulation of tumorigenesis.Citation3,Citation35–37 Thus, BAs is not only based on FXR acting on the liver and gallbladder through enterohepatic circulation, but also may be based on FXR acting on related molecules to treat intestinal diseases.
The mechanism of action between BAs and intestinal diseases still needs in- depth study. Current research indicates that modulating interaction among the microbiota, BAs and FXR is a promising therapeutic approach for the treatment of metabolic diseases. Therefore, this article reviews the effects of different BAs on the intestinal tract through the enterohepatic circulation via FXR to improve the treatment of intestinal diseases in the future.
BAs and enterohepatic circulation
Classification of BAs
As an endogenous signaling molecules regulating lipid, sterol, glucose and energy metabolism,Citation13 BAs play important roles in many pathological and physiological activities. BAs have different chemical structures (), which are complex and diverse. These complex structures of BAs are the key to their different physiological functions.
Figure1. Structure diagram of bile acids. Abbreviations: Cholic acid (CA), Hyodeoxycholic acid (HCA), α-Muricholic acid (α-MCA), β-Muricholic acid (β-MCA), ω-Muricholic acid (ω-MCA), Ursodeoxycholic acid (UDCA), Chenodeoxycholic acid (CDCA), Deoxycholic acid (DCA), Lithocholic acid (LCA), Murideoxycholic acid (MDCA); Hyodeoxycholic acid (HDCA)
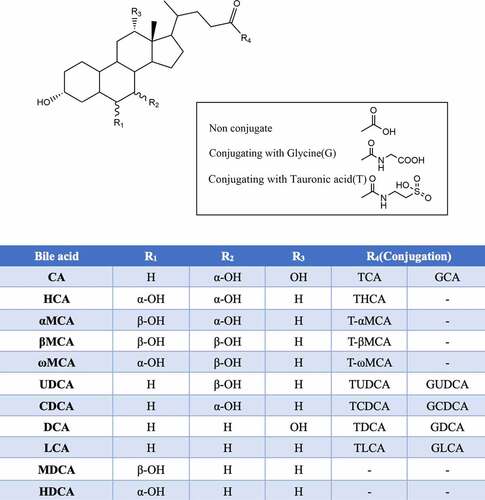
Based on whether they are conjugated with glycine or taurine, BAs are classified as conjugated BAs and unconjugated BAs. Notably, human BAs are conjugated with glycine and taurine, while rodent BAs are conjugated with taurine.Citation7
BAs are also classified as primary BAs and secondary BAs based on their source.Citation17 The primary BAs synthesized in the human liver are CA and CDCA. Notably, CA, CDCA, UDCA, α-MCA and β-MCA are synthesized in mice.Citation38 Primary conjugated BAs are first hydrolyzed by bile salt hydrolase (BSH) to generate unconjugated BAs; UDCA is then generated through epimerization, and secondary BAs are then generated through dehydroxylation catalyzed by 7α-dehydroxylase. In humans, secondary BAs include DCA and LCA. However, in rodents, hyocholic acid (HCA), murideoxycholic acid (MDCA), ω-MCA, hyodeoxycholic acid (HDCA), DCA and LCA can be produced.
BA molecules contains a hydrophilic pole (hydroxyl and carboxyl groups) and a hydrophobic pole (alkyl group). Due to the different modifications of their surface structure, BAs can have both a hydrophilic surface and a hydrophobic surface; that is, BAs have amphipathic properties. Hydrophilic BAs include UDCA and taurodeoxycholic acid (TDCA); hydrophobic BAs include DCA, LCA and CDCA. Generally, different forms of BAs play different roles in organisms.
FXR
BAs are produced in the liver through the breakdown of cholesterol and are then further metabolized by the intestinal flora to produce a set of chemically heterogeneous steroids. These steroids bind and activate members of cell surface and nuclear receptor families, collectively referred to as BA-activated receptors.Citation39 BA-activated receptors include FXR, Takeda G protein-coupled receptor 5 (TGR5), liver X receptor (LXR), pregnane X receptor (PXR), vitamin D receptor (VDR), constitutive androstane receptor (CAR), cholinergic receptor muscarinic 2 (CHRM2), et al.Citation40,Citation41 Among these receptors, FXR is the most important receptor that controls the BA, affects the transport of BA, and regulates the homeostasis of BA.Citation42–44 FXR is the bridge between the liver and intestines to control the level of BAs and regulate the concentration of BAs in the enterohepatic circulation.Citation13 FXR regulates the expression of genes involved in BA synthesis and transport in the liver and intestines and is thus the main modulator of BA homeostasis and enterohepatic circulation. FXR affects the concentration of BAs in various ways, mainly to regulate their synthesis, metabolism, recovery and transport of BAs. FXR regulates BAs through the enterohepatic circulation mainly via 3 main pathways: the small heterodimer partner (SHP) pathway, the mouse fibroblast growth factor 15 or fibroblast growth factor 19 (FGF15/ FGF19) pathway and the c-Jun N-terminal kinase (JNK) pathway. Liver FXR activation increases the expression of the target gene SHP,Citation45 which leads to the inhibition of cholesterol 7α-hydroxylase (CYP7A1) and sterol 12α-hydroxylase (CYP8B1).Citation33,Citation34,Citation46 However, interestingly, in Shp-null mice, BAs were shown to inhibited the expression of CYP7A1.Citation47,Citation48 This finding indicates that transcriptional inhibition of CYP7A1 by BAs involves other pathways that depend on not only SHP. Intestinal FXR activation by BAs can increase the expression of the intestinal FGF15/FGF19,Citation49 induce these proteins to enter the liver through the enterohepatic circulation, act on fibroblast growth factor receptor 4 (FGFR4), activate the JNK signaling pathway, inhibit the expression of CYP7A1, reduce BA synthesis, and improve cholestasis.Citation50–53 However, the expression of CYP7A1 in Fgfr4-null mice was found to be increased, as was the BA pool, further indicating that increased expression of FGF15 and FGFR4 inhibits the synthesis of BAs.Citation54 Intestinal FXR can also reduce the absorption of BAs by epithelial cells of the small intestinal mucosa and inhibit the enterohepatic circulation of BAs to further reduce the BA toxicity in hepatocytes and maintain BA homeostasis.
Numerous studies in mice have shown that Fxr-null is related to the pathological processes of many serious diseases. Liver Fxr-null mice showed elevated serum cholesterol and triglyceride levels and elevated levels of circulating free fatty acids, leading to abnormal glucose metabolism and severe fatty liver.Citation45,Citation55 Studies have indicated that intestinal Fxr-null mice is resistant to HFD-induced obesity, insulin resistance and NAFLD; thus, intestinal FXR has been confirmed to be related to exacerbation metabolic diseases.Citation56,Citation57
FXR has a typical nuclear receptor structure.Citation58 It consists of an N-terminal ligand-independent transcription activation function 1 (AF1) domain, a DNA binding domain (DBD), a ligand binding domain (LBD), a carboxy-terminal ligand-dependent transcription activation function 2 (AF2) domain, and a hinge region ().Citation59 FXR can bind to many endogenous BAs, including CDCA, taurocholic acid (TCA), DCA, LCA, CA and polyphenolic acid.Citation60 However, the pleiotropic downstream effects of FXR vary across BAs, and the metabolic effects of different BA receptors in different organs are also vary.Citation9 The strength of the natural activators of FXR follows the order of CDCA> DCA> CA> LCA,Citation57 and the natural inhibitors of FXR are tauro-α-MCA (T-α-MCA), tauro-β- MCA (T-β-MCA) and UDCA.Citation61–63 Notably, glycodeoxycholic acid (GCDCA), TCA and TDCA are weak activators of FXR.Citation64 Compared with conjugated BAs, unconjugated BAs have a stronger ability to activate FXR. These results may be explained by the different structures of BAs or the different mechanisms of binding to FXR; that is, the type and content of BAs produced in the body may regulate the expression of FXR. Therefore, it is important to consider the different mechanisms of different BAs and to link different types of BAs with specific diseases to provide valuable guidance for the diagnosis and clinical treatment of related diseases.
Enterohepatic circulation of BAs
BAs are the intermediates between the intestine and liver. BAs are the final product of cholesterol catabolism. Primary BAs are synthesized in hepatocytes through the classical or alternative pathway,Citation65,Citation66 conjugated with amino acids and secreted into the bile duct via bile salt export protein (BSEP).Citation67,Citation68 Postprandially, BAs are secreted into the duodenum through the biliary tract. Conjugated BAs are dehydroxylated under the action of the intestinal flora and BSH to generate secondary BAs. Approximately 95% of BAs are reabsorbed by intestinal cells in the terminal ileum through the apical sodium-dependent bile acid transporter (ASBT) and are transported to hepatocytes through the portal vein;Citation38,Citation69–71 this route is referred to as the enterohepatic circulation. However, approximately 5% of the remaining BAs content undergoes oxidation by microbes in the colon for side chain modification and is then excreted in the feces. ()
Figure 3. Synthesis and transformation of BAs in liver and intestine. Abbreviations: cholesterol 7α-hydroxylase (CYP7A1); sterol 27-hydroxylase (CYP27A1); oxysterol 7α-hydroxylase (CYP7B1); sterol 12α-hydroxylase (CYP8B1); acyl-CoA oxidase 2(ACOX2); bile acid–CoA: amino acid N-acyltransferase (BAAT); bile salt hydrolase (BSH); 3β-hydroxy-Δ5-C27-steroid dehydrogenase (HSD3B7); D-bifunctional protein (HSD17B4)
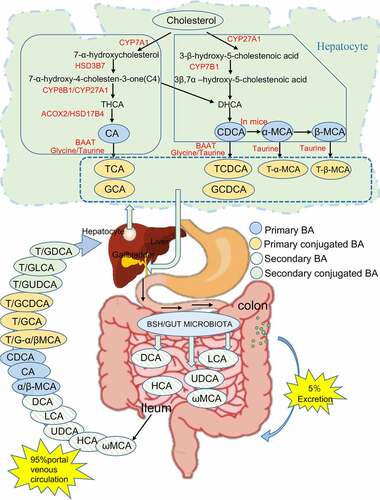
Under physiological conditions, the taurine/glycine conjugates in the side chain of BAs can be removed under the action of intestinal microorganisms.Citation72 Intestinal microorganisms can oxidize or dehydroxylate the hydroxyl groups at C3, C7 and C12 in the BA molecular structure to form unsaturated BAs, and can also convert BAs via carbonyl reduction or epimerization.Citation31,Citation73 CA is converted into DCA; CDCA is coverted to LCA; α-MCA and β-MCA in mice are converted to ω-MCA, HCA, and HDCA; et al.Citation74 Two main mechanisms control the reabsorption of BAs in the intestine. The first is active transport, which occurs mainly in the distal ileum, by which BAs can be effectively recovered by ASBT.Citation75 Almost all types of BAs are transported through this mechanism, but the absorption rates are different; these differences may depend mainly on the number of hydroxyl groups and molecular states of different BAs. The second mechanism is passive transport, which occurs mainly in the small intestine and colon. The rate of passive selective reabsorption depends on the degree and polarity of ionized. Unconjugated BAs and glycine conjugates of dihydroxy BAs (nonionized form) can also be reabsorbed by simple diffusion through the membrane of the small intestine can occur in any part of the small intestine. In summary, different BAs have different physical and chemical properties in the enterohepatic circulation, and the degree of action may be altered accordingly.
Effects of different BAs on the intestine
Roles of BAs in cells
The dynamic balance among intestinal epithelial cells, the intestinal flora and the intestinal mucosa is very important for maintaining intestinal permeability and normal tissue function. BAs have amphiphilic properties, and when combined with polar phospholipids, dietary lipids can be incorporated into the mixed solution in the intestinal lumen. This incorporation results in a micellization process, which is essential for fat absorption and systemic energy balance. In cells, BAs can be inserted into cell membranes, including plasma membranes; supraphysiolgical doses can also damage cell membranes and cause cell lysis. The hydrophobicity of BAs determines their cytotoxicity, which decreases in the following order: UDCA <CA <CDCA <DCA <LCA.Citation76 Hydrophobic BAs have high affinity for lipids and can damage mitochondria. DCA can colocalize with the outer mitochondrial membrane and disrupt its structure. Hydrophobic BAs have been shown to cause HepG2 cell apoptosis through endoplasmic reticulum stress. N-acyl phosphatidylethanolamine D (NAPE-PLD), an enzyme expressed in the brain and intestines, can convert membrane lipids into unique active lipids, and hydrophobic substrates can bind and stabilize NAPE-PLD. LCA has been shown to inhibit NAPE-PLD, while CDCA and DCA have been shown to activate NAPE-PLD; these difference may be attributed to the different hydroxyl sites in different BAs.Citation7
Enteroendocrine L cells are chemical sensors located in the intestinal epithelium that can sense BAs, monosaccharides, fatty acids and their microbial metabolites.Citation77 L cell hormones are secreted according to changes in the intestinal microenvironment, and the steady-state regulation of BAs affects the intestinal microenvironment. Niss Kristoffer et al.Citation78 used proteomic and gene expression data from GLUTag L cells to suggest that FXR is a multifaceted activating factor in L cells, but the mechanism by which FXR regulates intestinal L cell metabolism is unclear.
Hang Saiyu et al.Citation79 screened nearly 30 primary and secondary BA compounds and found two derivatives of LCA: 3-oxoLCA and isoalloLCA. 3-OxoLCA can the differentiation of IL-17a-expressing T helper cells (Th17 cells) by directly binding to its key transcription factor retinoid-related orphan receptor γt (RORγt), while isoalloLCA can enhance Treg cells differentiation through the production of mitochondrial reactive oxygen species (mitoROS). This finding suggests that BA metabolites can directly regulate the balance of Th17 and Treg cells, thereby regulating host immunity. Soon afterward, a new study showed that BA metabolites generated by the intestinal flora can induce the production of Treg cells and that these two components form a complex regulatory network to maintain the balance of the intestinal mucosal immune response. This study showed that in the presence of dendritic cells (DCs), 3-oxoDCA is less effective than isoDCA in promoting Treg cell proliferation and that 6-oxo-MCA is less less successful than ω-MCA in promoting Treg cell proliferation. This patten indicates that epimerization may play an important role in the biotransformation of BAs in the presence of bacterial flora. Ultimately, this study further revealed the effect of secondary BA metabolites of intestinal microorganisms on Treg cells and proved that isoDCA limits the activity of FXR in DCs and exerts an anti-inflammatory effect.Citation80 In addition, intestinal Paneth cells modulate innate immunity. Studies have found that Western diets rely on Clostridium-mediated increase in DCA levels in the ileum, as well as excessive transduction of FXR and type I interferon signals in intestinal epithelial cells, which leads to Paneth cell dysfunction in humans and mice. This study reveals the link between poor diet and innate immunity in the gut.Citation81 These studies clearly reveal the importance of BAs in shaping the host immune system.
Effects of BAs on the intestinal mucosa
The destruction of BA homeostasis plays a key role in intestinal inflammation.Citation82 Indeed, accumulating evidence indicates that BA metabolism disorders are related to malnutrition and mucosal inflammation.Citation11 In addition, studies have shown that a lack of intestinal FXR leads to increased intestinal permeability and decreased intestinal mucosal integrity.Citation83 The enterohepatic circulation regulates BA synthesis and the BA pool size through the joint action of FXR and FGF19.Citation84 In the ileum, BA-dependent FXR activation induces the expression of the intestinal factor FGF19. The first study revealing that FGF19-M52 can protect mice with FXR function against colitis proved that the FXR-FGF19-M52 axis plays a role in regulating the relative content of CDCA and CA by reducing BA synthesis.Citation85 However, interestingly, systemic and local anti-inflammatory activity was abolished in Fxr-null mice, emphasizing the necessity of FXR.Citation86 These results also showed that FXR is closely related to the integrity of the intestinal epithelial barrier and the suppression of inflammatory immune responses. This study assessed preclinical treatment of experimental colitis with FXR-FGF19 and BAs.
Regarding DCA, studies have shown that long-term excessive supplementation with DCA will inhibits the expression of organic solute transporter-β (OST-β) in the ileum,Citation87 significantly increases expression of CYP7A1 and CYP27A, and affects the intestinal FXR-FGF15 signaling pathway. Therefore, the negative feedback on BA synthesis is abolished,Citation88 leading to dysregulation of BAs in the small intestine and an excess concentration of BAs in feces, thereby promoting intestinal inflammation.Citation83,Citation89,Citation90
Barrier dysfunction can increase intestinal microbial abundance and barrier permeability. Destruction of the intestinal vascular barrier causes the translocation of bacteria or bacterial products.Citation91 The key to barrier maintenance is proper regulation of epithelial cell turnover through apoptosis. OCA can activate FXR to control intestinal permeability and play a role in intestinal barrier dysfunction and bacterial translocation.Citation92 In contrast, UDCA is an FXR antagonist,Citation93 but it can inhibit small intestinal inflammation by inducing immunosuppression, reducing bacterial translocation, increasing mucin production, inhibiting lipopolysaccharide production, and inducing intestinal cell apoptosis.Citation91 Some studies have suggested that UDCA and LCA can prevent intestinal inflammation in mouse models of colitis. UDCA and LCA may inhibit the lysis of colonic epithelial cells and expression of caspase-3 through FXR or TGR5 to resis t apoptosis.Citation94,Citation95 Current clinical studies have reported that UDCA and TUDCA can prevent or treat chronic inflammation of the small intestine.Citation96 UDCA has been proven to inhibit the expression of mucosal factors and reduce the severity of disease. CDCA is an effective inducer of epithelial permeability,Citation97 increasing epithelial damage, while CA does not induce epithelial permeability but is highly cytotoxic to intestinal epithelial cells.Citation98 Autophagy and inflammation are regulated bidirectionally.Citation99 Gupta Biki et al.Citation98 showed that both the cecal CDCA content in mice and cell permeability were increased and that sevelamer hydrochloride prevented the loss of barrier function induced by CDCA. CDCA and OCA inhibit autophagy via a mechanism dependent on FXR. UDCA can induce the formation of autophagosomes independent of FXR and enhance autophagic flux.Citation100
Regulation of the intestinal flora
A complex metabolic network connects the intestinal flora and BAs.Citation101 BAs are important metabolites that not only can regulate but also are modified by the intestinal flora. On the one hand, BAs can shape the microbiome through direct antibacterial effects and antimicrobial peptides produced by FXR, which can inhibit the bacteria in the intestinal tract and limit excessive proliferation of the flora.Citation102 On the other hand, the intestinal flora converts conjugated BAs into unconjugated BAs by encoding and expressing related enzymes ();Citation103 primary BAs are thus transformed into secondary BAs, resulting in changes in the composition and concentration of the bile pool.Citation104–106 For example, BSH metabolizes TCA into CA, and CA is metabolized into DCA by Clostridiumscindens 7α-dehydoxylation through the Bai operon. Recently, some researchers have successfully transferred the plasmid related to Bai enzyme into C. sporogenes to produce the MF001 strain. After the MF001 strain is incubated with CA, DCA can be produced with the incubation time. Therefore, the researchers successfully constructed a strain with the core Bai gene family and sufficient to promote the dehydroxylation of CA.Citation17 Chen Ming-liang et al.Citation107 proved that resveratrol can regulate the intestinal flora and reduce atherosclerosis. Specifically, resveratrol can increase the abundances of Lactobacillus and Bifidobacterium by inhibiting the ileal FXR-FGF15 axis, thereby increasing the activity of BSH, promoting the deconjugation of conjugated BAs, increasing the excretion of BAs in feces, and finally reducing the content of BAs in the ileum. BAs not only inhibit the overproduction and harmful colonization of the intestinal flora but also rely on the numerous species of symbiotic bacteria to assist in digestion. FXR can regulate the entry point of bacterial translocation to regulate the intestinal vascular barrier.Citation91 Therefore, the toxic changes in BAs are closely associated with the intestinal flora, and the intestinal flora is reciprocally involved in the production of BAs.Citation108 Studies have shown that OCA activates the FXR receptor, which can inhibit the synthesis of endogenous BAs, reduce the proliferation of bacteria, and thereby affect the composition of the small intestinal flora.Citation109–111 FXR-dependent inhibition of endogenous BA secretion alters the intestinal flora instead of exerting a direct effect on OCA.Citation112,Citation113
Figure 4. Conversion of bile acids in the intestine. (a). Conjugated BAs: T/GCA structural transformation in the intestine. (b). Conjugated BAs: T/GCDCA structural transformation in the intestine. Abbreviations: 7β-hydroxysteroid dehydrogenase (7β-HSDH); (3α-HSDH) 3α-hydroxysteroid dehydrogenase; sterol 6β-hydroxylase (Cyp2c70); bile salt hydrolase (BSH)
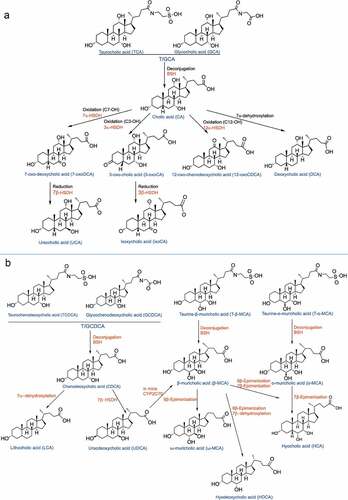
DCA affects the relative abundances of intestinal flora. This molecule is positively correlated with the Clostridium and Eubacteriaceae abundances and negatively correlated with the Bacteroides abundances. Recent studies have shown that chokeberry polyphenols can reduce the relative contents of CA and DCA by extending the treatment time to alter the composition of the intestinal flora and can increase the relative content of CDCA.Citation114 These changes were found to be positively related to the Bacillus abundance and negatively related to the Clostridium abundance.Citation115 This research led to the conclusion that treatment with chokeberry polyphenols is likely to activate FXR or TGR5.Citation109
Unconjugated BAs have stronger antibacterial activity than conjugated BAs, and gram-positive bacteria are more sensitive to BAs than gram-negative bacteria. OCA stimulates FXR, inhibits BA synthesis, and reversibly induces the proliferation of gram-positive bacteria in the small intestine.Citation112 Clostridium difficile is a gram-positive spore-forming bacillus that can damage the intestinal epithelium and cause a strong inflammatory response, which can lead to diarrhea. Clostridium difficile is a gram-positive spore-forming bacillus that damages the intestinal epithelium and causes a strong inflammatory response, which can lead to diarrhea. Studies have found that OCA regulates liver BA synthesis through feedback inhibition of intestinal FXR, thereby improving the severity of Clostridium difficile infection in obese mice induced by a high-fat diet (HFD).Citation116 An increase in LCA can reduce the toxicity to the cecal microbiota and increase the abundance of Clostridia. Clostridia can synthesize DCA and LCA, which can improve metabolism of the host.Citation117 UDCA can inhibit harmful bacteria, increase the proportion of Bacteroides, and play an important role in intestinal bacterial homeostasis. CDCA and DCA can reduce sodium reabsorption and increase chloride ion secretion in the human ileum and colon, while CA cannot. However, through the action of intestinal bacteria, nonsecreted CA can be transformed into DCA, which is secreted in feces. Therefore, supplementation with DCA may be able to alleviate the symptoms of constipation. Many studies have shown that the intestinal flora is related to various metabolic diseases, including obesity, nonalcoholic fatty liver and insulin resistance.Citation110,Citation118 In the future, it may be possible to regulate BA metabolism by identifying specific flora to control and treat corresponding intestinal diseases.
Regulation of tumors
The diversity of BA is receiving more and more attention because it not only affects host metabolism and innate immunity, but also has tumor-promoting properties.Citation7,Citation119,Citation120 Suppression or induction of FXR expression can cause various diseases, such as gastrointestinal diseases, liver hypertrophy, cirrhosis, cholestasis, atherosclerosis, inflammation and cancer.Citation121 Whole-body Fxr-null mice spontaneously developed liver tumors,Citation37 but the lack of mouse hepatocyte-specific FXR did not promote the occurrence of spontaneous liver tumors.Citation86,Citation122,Citation123 Absil et al. pointed out that the high expression of FXR in breast cancer cells promotes bone metastasis of tumor cells.Citation124 In addition, overexpression of FXR may cause non-small cell lung cancer (NSCLC) and esophageal adenocarcinoma. Among the various nuclear receptors, FXR is considered to be a tumor suppressor that can block the initiation of colorectal cancer (CRC) through metabolic or epigenetic mechanisms.Citation104–106 FXR can regulate BAM metabolism and exhibit a tumor-suppressive effect in the colon.Citation125 Intestinal inflammation is a contributing factor to the development of CRC in patients with Irritable bowel disease (IBD).Citation126 Enteral malnutrition is one of the most likely predisposing factors for IBD. However, intestinal malnutrition leads to a decrease in microbial diversity and a decrease in Firmicutes bacteria, resulting in decrease in intestinal secondary BAs levels and increase in conjugated BAs level.Citation127 Hydrophobicity increases the levels of BAs in tumors, while hydrophilicity has the opposite effect. The hydrophobicity of BA is determined by cytotoxicity.Citation128 DCA, LCA, CDCA and TCDCA have been individually proven to have cytotoxic and cancer-promoting properties.Citation129 Among them, DCA affects the signal transduction pathway of epithelial cells, leading to the development of CRC. In addition, studies of CRC mouse models have shown that elevated levels of fecal BAs lead to an increase in the incidence of CRC. In studies of obesity-related CRC, HFD increases the risk of CRC in humans, which may be due to the excretion of CA into the intestine and increase the production of secondary BAs.Citation130,Citation131 Studies have revealed that the convergence of HFD and dysregulated WNT signals changes the BA profile to drive the malignant transformation of Lgr5 expression (Lgr5 +) cancer stem cells and promote the progression of adenoma-adenocarcinoma. In mechanism, FXR controls the proliferation of Lgr5+ intestinal stem cells. In CRC, dietary and genetic risk factors are shown to converge to drive FXR-dependent Lgr5 + CSC proliferation and disease progression. Conversely, the activation of FXR in the intestine reduces the severity of the disease, thereby significantly improving survival.Citation132 Inflammation of the intestine reduces FXR activation. Therefore, FXR can not only inhibit the inflammatory response, but also can be targeted by the inflammatory response itself. This may lead to a vicious circle. Decreased FXR activity leads to decreased inhibition of inflammation, which leads to the occurrence of chronic intestinal inflammation, and may lead to the occurrence of inflammation-induced colorectal cancer.Citation35 FXR maintains the BA concentration in the physiological concentration range, thereby preventing the cytotoxicity induced by BAs. FXR is the main regulator of BA homeostasis. In view of the relationship between abnormal levels of BA and CRC, researchers have presented a large amount of evidence that FXR plays a role in intestinal tumorigenesis. In view of the key role of FXR in maintaining the concentration of BAs within the physiological range, thereby preventing BAs-induced cytotoxicity.
Conclusion and future perspectives
As a signaling molecules regulating glucose, sterol and lipid metabolism, BAs has attracted widespread attention. This article reviews the latest research on different types of BAs on the intestinal tract from the perspective of FXR and will provide a reference for subsequent basic and clinical research. Different BAs have different effects on the intestine and liver, but few clinical studies have focused on the intestine. Thus, the regulation of intestinal microbial metabolism and intestinal mucosal inflammation by BAs needs further research. Different BAs have different structures, act on different regions, and activate different receptors and downstream signal transduction pathways, which may lead to various effects. The final outcome may be the result of competition among several different signaling pathways under the influence of specific environmental factors. In this review, the role of BA-FXR receptor signaling in intestinal regulation and the effects of changes in the BA composition on host metabolism are discussed. BAs may be a new targeted treatment method for metabolic syndrome.
Supplemental Material
Download Zip (162 B)Disclosure Statement
The authors declare no competing interests.
Supplementary material
Supplemental data for this article can be accessed on thepublisher’s website.
Additional information
Funding
References
- Wan YD, Zhu RX, Pan XT, Sun TW. Bile acid supplementation improves murine pancreatitis in association with the gut microbiota. Front Physiol. 2020;11:650. doi:10.3389/fphys.2020.00650.
- Martinot E, Sèdes L, Baptissart M, Lobaccaro JM, Caira F, Beaudoin C, Volle DH. Bile acids and their receptors. Mol Aspects Med. 2017;56:2–17. doi:10.1016/j.mam.2017.01.006.
- Taoka H, Yokoyama Y, Morimoto K, Kitamura N, Tanigaki T, Takashina Y, Tsubota K, Watanabe M. Role of bile acids in the regulation of the metabolic pathways. World J Diabetes. 2016;7(13):260–270. doi:10.4239/wjd.v7.i13.260.
- Arab JP, Karpen SJ, Dawson PA, Arrese M, Trauner M. Bile acids and nonalcoholic fatty liver disease: molecular insights and therapeutic perspectives. Hepatology. 2017;65(1):350–362. doi:10.1002/hep.28709.
- Joyce SA, Gahan CG. Disease-associated changes in bile acid profiles and links to altered gut microbiota. Dig Dis. 2017;35(3):169–177. doi:10.1159/000450907.
- Xie X, Dong J, Lu G, Gao K, Li X, Mao W, Chen F, Tong Z, Li B, Li W. Increased circulating total bile acid levels were associated with organ failure in patients with acute pancreatitis. BMC Gastroenterol. 2020;20(1):222. doi:10.1186/s12876-020-01243-w.
- Ahmad TR, Haeusler RA. Bile acids in glucose metabolism and insulin signalling - mechanisms and research needs. Nat Rev Endocrinol. 2019;15(12):701–712. doi:10.1038/s41574-019-0266-7.
- Wang H, Chen J, Hollister K, Sowers L, Forman B. Endogenous bile acids are ligands for the nuclear receptor FXR/BAR. Mol Cell. 1999;3(5):543–553. doi:10.1016/s1097-2765(00)80348-2.
- Qiu Y, Shen L, Fu L, Yang J, Cui C, Li T, Li X, Fu C, Gao X, Wang W, et al. The glucose-lowering effects of alpha-glucosidase inhibitor require a bile acid signal in mice. Diabetologia. 2020;63(5):1002–1016. doi:10.1007/s00125-020-05095-7.
- Van De Wiel SMW, Bijsmans ITGW, Van Mil SWC, Van De Graaf SFJ. Identification of FDA-approved drugs targeting the Farnesoid X Receptor. Sci Rep. 2019;9(1):2193. doi:10.1038/s41598-019-38668-7.
- Hofmann AF. The enterohepatic circulation of bile acids in mammals: form and functions. Front Biosci. 2009;14(7):2584–2598. doi:10.2741/3399.
- Gonzalez FJ. Nuclear receptor control of enterohepatic circulation. Compr Physiol. 2012;2(4):2811–2828. doi:10.1002/cphy.c120007.
- Matsubara T, Li F, Gonzalez FJ. FXR signaling in the enterohepatic system. Mol Cell Endocrinol. 2013;368:17–29. doi:10.1016/j.mce.2012.05.004.
- Ridlon JM, Kang DJ, Hylemon PB, Bajaj JS. Bile acids and the gut microbiome. Curr Opin Gastroenterol. 2014;30(3):332–338. doi:10.1097/MOG.0000000000000057.
- Gérard P. Metabolism of cholesterol and bile acids by the gut microbiota. Pathogens. 2014;3(1):14–24. doi:10.3390/pathogens3010014.
- Quinn RA, Melnik AV, Vrbanac A, Fu T, Patras KA, Christy MP, Bodai Z, Belda-Ferre P, Tripathi A, Chung LK, et al. Global chemical effects of the microbiome include new bile-acid conjugations. Nature. 2020;579(7797):123–129. doi:10.1038/s41586-020-2047-9.
- Funabashi M, Grove TL, Wang M, Varma Y, Mcfadden ME, Brown LC, Guo C, Higginbottom S, Almo SC, Fischbach MA. A metabolic pathway for bile acid dehydroxylation by the gut microbiome. Nature. 2020;582(7813):566–570. doi:10.1038/s41586-020-2396-4.
- Ellis E, Axelson M, Abrahamsson A, Eggertsen G, Thörne A, Nowak G, Ericzon BG, Björkhem I, Einarsson C. Feedback regulation of bile acid synthesis in primary human hepatocytes: evidence that CDCA is the strongest inhibitor. Hepatology. 2003;38(4):930–938. doi:10.1053/jhep.2003.50394.
- Einarsson C, Ellis E, Abrahamsson A, Ericzon BG, Björkhem I, Axelson M. Bile acid formation in primary human hepatocytes. World J Gastroenterology. 2000;6(4):522–525. doi:10.3748/wjg.v6.i4.522.
- Ellis ECS, Nauglers S, Parini P, Mörk LM, Jorns C, Zemack H, Vgren S, Björkhem I, Ericzon BGR, Wilson EM, et al. Mice with chimeric livers are an improved model for human lipoprotein metabolism. PLoS One. 2013;8(11):e78550. doi:10.1371/journal.pone.0078550.
- Li J, Dawson PA. Animal models to study bile acid metabolism. Biochim Biophys Acta Mol Basis Dis. 2019;1865(5):895–911. doi:10.1016/j.bbadis.2018.05.011.
- Chiang JYL, Ferrell JM. Bile acid biology, pathophysiology, and therapeutics. Clin Liver Dis (Hoboken). 2020;15(3):91–94. doi:10.1002/cld.861.
- Kowdley K, Luketic V, Chapman R, Hirschfield G, Poupon R, Schramm C, Vincent C, Rust C, Parés A, Mason A, et al. A randomized trial of obeticholic acid monotherapy in patients with primary biliary cholangitis. Hepatology. 2018;67(5):1890–1902. doi:10.1002/hep.29569.
- Sanyal AJ. Use of farnesoid X receptor agonists to treat nonalcoholic fatty liver disease. Dig Dis. 2015;33(3):426–432. doi:10.1159/000371698.
- Neuschwander Tetri B, Loomba R, Sanyal A, Lavine J, Van Natta M, Abdelmalek M, Chalasani N, Dasarathy S, Diehl A, Hameed B, et al. Farnesoid X nuclear receptor ligand obeticholic acid for non-cirrhotic, non-alcoholic steatohepatitis (FLINT): a multicentre, randomised, placebo-controlled trial. Lancet. 2015;385(9972):956–965. doi:10.1016/s0140-6736(14)61933-4.
- Copple B, Li T. Pharmacology of bile acid receptors: evolution of bile acids from simple detergents to complex signaling molecules. Pharmacol Res. 2016;104:9–21. doi:10.1016/j.phrs.2015.12.007.
- Deng X, Ye Z, Cao H, Bai Y, Che Q, Guo J, Su Z. Chitosan oligosaccharide ameliorated obesity by reducing endoplasmic reticulum stress in diet-induced obese rats. Food Funct. 2020;11(7):6285–6296. doi:10.1039/d0fo01107j.
- Kjærgaard K, Frisch K, Sørensen M, Munk OL, Hofmann AF, Horsager J, Schacht AC, Erickson M, Shapiro D, Keiding S. Obeticholic acid improves hepatic bile acid excretion in patients with primary biliary cholangitis. J Hepatol. 2021;74(1):58–65. doi:10.1016/j.jhep.2020.07.028.
- Zhu Y, Xu S, Lu Y, Wei Y, Yao B, Guo F, Zheng X, Wang Y, He Y, Jin L, et al. Repositioning an immunomodulatory drug vidofludimus as a farnesoid X receptor modulator with therapeutic effects on NAFLD. Front Pharmacol. 2020;11:590. doi:10.3389/fphar.2020.00590.
- Kowdley KV, Vuppalanchi R, Levy C, Floreani A, Andreone P, Larusso NF, Shrestha R, Trotter J, Goldberg D, Rushbrook S, et al. A randomized, placebo-controlled, phase II study of obeticholic acid for primary sclerosing cholangitis. J Hepatol. 2020;73(1):94–101. doi:10.1016/j.jhep.2020.02.033.
- Li T, Chiang JYL. Bile acid-based therapies for non-alcoholic steatohepatitis and alcoholic liver disease. Hepatobiliary Surg Nutr. 2020;9(2):152–169. doi:10.21037/hbsn.2019.09.03.
- Huang S, Wu Y, Zhao Z, Wu B, Sun K, Wang H, Qin L, Bai F, Leng Y, Tang W. A new mechanism of obeticholic acid on NASH treatment by inhibiting NLRP3 inflammasome activation in macrophage. Metabolism. 2021;120:154797. doi:10.1016/j.metabol.2021.154797.
- Goodwin B, Jones SA, Price RR, Watson MA, Mckee DD, Moore LB, Galardi C, Wilson JG, Lewis MC, Roth ME, et al. A regulatory cascade of the nuclear receptors FXR, SHP-1, and LRH-1 represses bile acid biosynthesis. Mol Cell. 2000;6(3):517–526. doi:10.1016/s1097-2765(00)00051-4.
- Lu TT, Makishima M, Repa JJ, Schoonjans K, Kerr TA, Auwerx J, Mangelsdorf DJ. Molecular basis for feedback regulation of bile acid synthesis by nuclear receptors. Mol Cell. 2000;6(3):507–515. doi:10.1016/s1097-2765(00)00050-2.
- Gadaleta RM, Van Erpecum KJ, Oldenburg B, Willemsen ECL, Renooij W, Murzilli S, Klomp LWJ, Siersema PD, Schipper MEI, Danese S, et al. Farnesoid X receptor activation inhibits inflammation and preserves the intestinal barrier in inflammatory bowel disease. Gut. 2011;60(4):463–472. doi:10.1136/gut.2010.212159.
- Vavassori P, Mencarelli A, Renga B, Distrutti E, Fiorucci S. The bile acid receptor FXR is a modulator of intestinal innate immunity. J Immunol. 2009;183(10):6251–6261. doi:10.4049/jimmunol.0803978.
- Yang F, Huang X, Yi T, Yen Y, Moore DD, Huang W. Spontaneous development of liver tumors in the absence of the bile acid receptor farnesoid X receptor. Cancer Res. 2007;67(3):863–867. doi:10.1158/0008-5472.Can-06-1078.
- Wahlström A, Sayin S, Marschall H, Bäckhed F. Intestinal crosstalk between bile acids and microbiota and its impact on host metabolism. Cell Metab. 2016;24(1):41–50. doi:10.1016/j.cmet.2016.05.005.
- Fiorucci S, Baldoni M, Ricci P, Zampella A, Distrutti E, Biagioli M. Bile acid-activated receptors and the regulation of macrophages function in metabolic disorders. Curr Opin Pharmacol. 2020;53:45–54. doi:10.1016/j.coph.2020.04.008.
- Wan YY, Sheng L. Regulation of bile acid receptor activity. Liver Res. 2018;2(4):180–185. doi:10.1016/j.livres.2018.09.008.
- Cheng J, Shah YM, Ma X, Pang X, Tanaka T, Kodama T, Krausz KW, Gonzalez FJ. Therapeutic role of rifaximin in inflammatory bowel disease: clinical implication of human pregnane X receptor activation. J Pharmacol Exp Ther. 2010;335(1):32–41. doi:10.1124/jpet.110.170225.
- Pols TW, Noriega LG, Nomura M, Auwerx J, Schoonjans K. The bile acid membrane receptor TGR5 as an emerging target in metabolism and inflammation. J Hepatol. 2011;54(6):1263–1272. doi:10.1016/j.jhep.2010.12.004.
- Lee S, Lee K, Yoon S, Lee JW, Lee D. Anomalies in network bridges involved in bile Acid metabolism predict outcomes of colorectal cancer patients. PloS One. 2014;9(9):e107925. doi:10.1371/journal.pone.0107925.
- Nie YF, Hu J, Yan XH. Cross-talk between bile acids and intestinal microbiota in host metabolism and health. J Zhejiang Univ Sci B. 2015;16(6):436–446. doi:10.1631/jzus.B1400327.
- Ma K, Saha PK, Chan L, Moore DD. Farnesoid X receptor is essential for normal glucose homeostasis. J Clin Invest. 2006;116(4):1102–1109. doi:10.1172/jci25604.
- Malhi H, Camilleri M. Modulating bile acid pathways and TGR5 receptors for treating liver and GI diseases. Curr Opin Pharmacol. 2017;37:80–86. doi:10.1016/j.coph.2017.09.008.
- Kerr TA, Saeki S, Schneider M, Schaefer K, Berdy S, Redder T, Shan B, Russell DW, Schwarz M. Loss of nuclear receptor SHP impairs but does not eliminate negative feedback regulation of bile acid synthesis. Dev Cell. 2002;2(6):713–720. doi:10.1016/S1534-5807(02)00154-5.
- Wang L, Lee Y, Bundman D, Han Y, Thevananther S, Kim CS, Chua SS, Wei P, Heyman RA, Karin M, et al. Redundant pathways for negative feedback regulation of bile acid production. Dev Cell. 2002;2(6):721–731. doi:10.1016/s1534-5807(02)00187-9.
- Fang S, Suh JM, Reilly SM, Yu E, Osborn O, Lackey D, Yoshihara E, Perino A, Jacinto S, Lukasheva Y, et al. Intestinal FXR agonism promotes adipose tissue browning and reduces obesity and insulin resistance. Nat Med. 2015;21(2):159–165. doi:10.1038/nm.3760.
- Holt JA, Luo G, Billin AN, Bisi J, Mcneill YY, Kozarsky KF, Donahee M, Wang DY, Mansfield TA, Kliewer SA, et al. Definition of a novel growth factor-dependent signal cascade for the suppression of bile acid biosynthesis. Genes Dev. 2003;17(13):1581–1591. doi:10.1101/gad.1083503.
- Inagaki T, Choi M, Moschetta A, Peng L, Cummins CL, Mcdonald JG, Luo G, Jones SA, Goodwin B, Richardson JA, et al. Fibroblast growth factor 15 functions as an enterohepatic signal to regulate bile acid homeostasis. Cell Metab. 2005;2(4):217–225. doi:10.1016/j.cmet.2005.09.001.
- Zhu Y, Li F, Guo GL. Tissue-specific function of farnesoid X receptor in liver and intestine. Sci Rep. 2011;63(4):259–265. doi:10.1016/j.phrs.2010.12.018.
- Modica S, Petruzzelli M, Bellafante E, Murzilli S, Salvatore L, Celli N, Di Tullio G, Palasciano G, Moustafa T, Halilbasic E, et al. Selective activation of nuclear bile acid receptor FXR in the intestine protects mice against cholestasis. Gastroenterology. 2012;142(2):355–65.e1-4. doi:10.1053/j.gastro.2011.10.028.
- Yu C, Wang F, Kan M, Jin C, Jones RB, Weinstein M, Deng CX, Mckeehan WL. Elevated cholesterol metabolism and bile acid synthesis in mice lacking membrane tyrosine kinase receptor FGFR4. J Biol Chem. 2000;275(20):15482–15489. doi:10.1074/jbc.275.20.15482.
- Sinal CJ, Tohkin M, Miyata M, Ward JM, Lambert G, Gonzalez FJ. Targeted disruption of the nuclear receptor FXR/BAR impairs bile acid and lipid homeostasis. Cell. 2000;102(6):731–744. doi:10.1016/s0092-8674(00)00062-3.
- Gonzalez FJ, Jiang C, Patterson AD. An intestinal microbiota-farnesoid X receptor axis modulates metabolic disease. Gastroenterology. 2016;151(5):845–859. doi:10.1053/j.gastro.2016.08.057.
- Jiang C, Xie C, Li F, Zhang L, Nichols RG, Krausz KW, Cai J, Qi Y, Fang ZZ, Takahashi S, et al. Intestinal farnesoid X receptor signaling promotes nonalcoholic fatty liver disease. J Clin Invest. 2015;125(1):386–402. doi:10.1172/jci76738.
- Emery NJ. Cognitive ornithology: the evolution of avian intelligence. Philos Trans R Soc Lond B Biol Sci. 2006;361(1465):23–43. doi:10.1098/rstb.2005.1736.
- Rastinejad F, Huang P, Chandra V, Khorasanizadeh S. Understanding nuclear receptor form and function using structural biology. J Mol Endocrinol. 2013;51(3):T1–T21. doi:10.1530/JME-13-0173.
- Parks DJ, Blanchard SG, Bledsoe RK, Chandra G, Consler TG, Kliewer SA, Stimmel JB, Willson TM, Zavacki AM, Moore DD, et al. Bile acids: natural ligands for an orphan nuclear receptor. Science. 1999;284(5418):1365–1368. doi:10.1126/science.284.5418.1365.
- Dimarzio M, Rusconi B, Yennawar NH, Eppinger M, Patterson AD, Dudley EG. Identification of a mouse Lactobacillus johnsonii strain with deconjugase activity against the FXR antagonist T-β-MCA. PloS One. 2017;12(9):e0183564. doi:10.1371/journal.pone.0183564.
- Sayin SI, Wahlström A, Felin J, Jäntti S, Marschall HU, Bamberg K, Angelin B, Hyötyläinen T, Orešič M, Bäckhed F. Gut microbiota regulates bile acid metabolism by reducing the levels of tauro-beta-muricholic acid, a naturally occurring FXR antagonist. Cell Metab. 2013;17(2):225–235. doi:10.1016/j.cmet.2013.01.003.
- Jiang C, Xie C, Lv Y, Li J, Krausz KW, Shi J, Brocker CN, Desai D, Amin SG, Bisson WH, et al. Intestine-selective farnesoid X receptor inhibition improves obesity-related metabolic dysfunction. Nat Commun. 2015;6:10166. doi:10.1038/ncomms10166.
- Vaquero J, Monte MJ, Dominguez M, Muntané J, Marin JJ. Differential activation of the human farnesoid X receptor depends on the pattern of expressed isoforms and the bile acid pool composition. Biochem Pharmacol. 2013;86(7):926–939. doi:10.1016/j.bcp.2013.07.022.
- Chiang JYL, Ferrell JM. Bile Acid Metabolism in Liver Pathobiology. Gene Expr. 2018;18(2):71–87. doi:10.3727/105221618x15156018385515.
- Li-Hawkins J, Gåfvels M, Olin M, Lund EG, Andersson U, Schuster G, Björkhem I, Russell DW, Eggertsen G. Cholic acid mediates negative feedback regulation of bile acid synthesis in mice. J Clin Invest. 2002;110(8):1191–1200. doi:10.1172/jci16309.
- Haeusler RA, Camastra S, Nannipieri M, Astiarraga B, Castro-Perez J, Xie D, Wang L, Chakravarthy M, Ferrannini E. Increased Bile Acid Synthesis and Impaired Bile Acid Transport in Human Obesity. J Clin Endocrinol Metab. 2016;101(5):1935–1944. doi:10.1210/jc.2015-2583.
- Reue K, Lee JM, Vergnes L. Regulation of bile acid homeostasis by the intestinal Diet1-FGF15/19 axis. Curr Opin Lipidol. 2014;25(2):140–147. doi:10.1097/mol.0000000000000060.
- Dawson PA, Hubbert ML, Rao A. Getting the mOST from OST: role of organic solute transporter, OSTalpha-OSTbeta, in bile acid and steroid metabolism. Biochim Biophys Acta. 2010;1801(9):994–1004. doi:10.1016/j.bbalip.2010.06.002.
- Döring B, Lütteke T, Geyer J, Petzinger EJCTIM. The SLC10 carrier family: transport functions and molecular structure. Curr Top Membr. 2012;70:105–168. doi:10.1016/b978-0-12-394316-3.00004-1.
- Slijepcevic D, Van De Graaf SF. Bile acid uptake transporters as targets for therapy. Dig Dis. 2017;35(3):251–258. doi:10.1159/000450983.
- Fukui H. Gut-liver axis in liver cirrhosis: how to manage leaky gut and endotoxemia. World J Hepatol. 2015;7(3):425–442. doi:10.4254/wjh.v7.i3.425.
- Rath CM, Alexandrov T, Higginbottom SK, Song J, Milla ME, Fischbach MA, Sonnenburg JL, Dorrestein PC. Molecular analysis of model gut microbiotas by imaging mass spectrometry and nanodesorption electrospray ionization reveals dietary metabolite transformations. Anal Chem. 2012;84(21):9259–9267. doi:10.1021/ac302039u.
- Fickert P, Hirschfield GM, Denk G, Marschall HU, Altorjay I, Färkkilä M, Schramm C, Spengler U, Chapman R, Bergquist A, et al. norUrsodeoxycholic acid improves cholestasis in primary sclerosing cholangitis. J Hepatol. 2017;67(3):549–558. doi:10.1016/j.jhep.2017.05.009.
- Chopyk D, Grakoui A. Contribution of the intestinal microbiome and gut barrier to hepatic disorders. Gastroenterology. 2020;159(3). doi:10.1053/j.gastro.2020.04.077.
- Hegade VS, Speight RA, Etherington RE, Jones DEJ. Novel bile acid therapeutics for the treatment of chronic liver diseases. Therap Adv Gastroenterol. 2016;9(3):376–391. doi:10.1177/1756283x16630712.
- Thomas C, Gioiello A, Noriega L, Strehle A, Oury J, Rizzo G, Macchiarulo A, Yamamoto H, Mataki C, Pruzanski M, et al. TGR5-mediated bile acid sensing controls glucose homeostasis. Cell Metab. 2009;10(3):167–177. doi:10.1016/j.cmet.2009.08.001.
- Niss K, Jakobsson ME, Westergaard D, Belling KG, Olsen JV, Brunak S. Effects of active farnesoid X receptor on GLUTag enteroendocrine L cells. Mol Cell Endocrinol. 2020;517:110923. doi:10.1016/j.mce.2020.110923.
- Hang S, Paik D, Yao L, Kim E, Trinath J, Lu J, Ha S, Nelson BN, Kelly SP, Wu L, et al. Bile acid metabolites control TH17 and Treg cell differentiation. Nature. 2019;576(7785):143–148. doi:10.1038/s41586-019-1785-z.
- Campbell C, Mckenney PT, Konstantinovsky D, Isaeva OI, Schizas M, Verter J, Mai C, Jin WB, Guo CJ, Violante S, et al. Bacterial metabolism of bile acids promotes generation of peripheral regulatory T cells. Nature. 2020;581(7809):475–479. doi:10.1038/s41586-020-2193-0.
- Liu TC, Kern JT, Jain U, Sonnek NM, Xiong S, Simpson KF, Vandussen KL, Winkler ES, Haritunians T, Malique A, et al. Western diet induces Paneth cell defects through microbiome alterations and farnesoid X receptor and type I interferon activation. Cell Host Microbe. 2021. May 12;3. doi:10.1016/j.chom.2021.04.004.
- Tremblay S, Romain G, Roux M, Chen XL, Brown K, Gibson DL, Ramanathan S, Menendez A. Bile acid administration elicits an intestinal antimicrobial program and reduces the bacterial burden in two mouse models of enteric infection. Infect Immun. 2017;85(6). doi:10.1128/iai.00942-16.
- Chen ML, Takeda K, Sundrud MS. Emerging roles of bile acids in mucosal immunity and inflammation. Mucosal Immunol. 2019;12(4):851–861. doi:10.1038/s41385-019-0162-4.
- Wang W, Zhao J, Gui W, Sun D, Dai H, Xiao L, Chu H, Du F, Zhu Q, Schnabl B, et al. Tauroursodeoxycholic acid inhibits intestinal inflammation and barrier disruption in mice with non-alcoholic fatty liver disease. Br J Pharmacol. 2018;175(3):469–484. doi:10.1111/bph.14095.
- Gadaleta RM, Garcia-Irigoyen O, Cariello M, Scialpi N, Peres C, Vetrano S, Fiorino G, Danese S, Ko B, Luo J, et al. Fibroblast Growth Factor 19 modulates intestinal microbiota and inflammation in presence of Farnesoid X Receptor. EBioMedicine. 2020;54:102719. doi:10.1016/j.ebiom.2020.102719.
- Mao J, Chen X, Wang C, Li W, Li J. Effects and mechanism of the bile acid (farnesoid X) receptor on the Wnt/beta-catenin signaling pathway in colon cancer. Oncol Lett. 2020;20(1):337–345. doi:10.3892/ol.2020.11545.
- Xu M, Cen M, Shen Y, Zhu Y, Cheng F, Tang L, Hu W, Dai N. Deoxycholic acid-induced gut dysbiosis disrupts bile acid enterohepatic circulation and promotes intestinal inflammation. Dig Dis Sci. 2020. doi:10.1007/s10620-020-06208-3.
- Ji S, Liu Q, Zhang S, Chen Q, Wang C, Zhang W, Xiao C, Li Y, Nian C, Li J, et al. FGF15 activates hippo signaling to suppress bile acid metabolism and liver tumorigenesis. Dev Cell. 2019;48(4):460–474.e9. doi:10.1016/j.devcel.2018.12.021.
- Du J, Chen Q, Li Y, Xiang X, Xu W, Mai K, Ai Q. Activation of the Farnesoid X receptor (FXR) suppresses linoleic acid-induced inflammation in the large yellow croaker (Larimichthys crocea). J Nutr. 2020. doi:10.1093/jn/nxaa185.
- Fuchs CD, Krivanec S, Steinacher D, Mlitz V, Wahlstrom A, Stahlman M, Claudel T, Scharnagl H, Stojakovic T, Marschall HU, et al. Absence of Bsep/Abcb11 attenuates MCD diet-induced hepatic steatosis but aggravates inflammation in mice. Liver Int. 2020;40(6):1366–1377. doi:10.1111/liv.14423.
- Mouries J, Brescia P, Silvestri A, Spadoni I, Sorribas M, Wiest R, Mileti E, Galbiati M, Invernizzi P, Adorini L, et al. Microbiota-driven gut vascular barrier disruption is a prerequisite for non-alcoholic steatohepatitis development. J Hepatol. 2019;71(6):1216–1228. doi:10.1016/j.jhep.2019.08.005.
- Siddiqui MS, Van Natta ML, Connelly MA, Vuppalanchi R, Neuschwander-Tetri BA, Tonascia J, Guy C, Loomba R, Dasarathy S, Wattacheril J, et al. Impact of obeticholic acid on the lipoprotein profile in patients with non-alcoholic steatohepatitis. J Hepatol. 2020;72(1):25–33. doi:10.1016/j.jhep.2019.10.006.
- Festi D, Montagnani M, Azzaroli F, Lodato F, Mazzella G, Roda A, Di Biase AR, Roda E, Simoni P, Colecchia A. Clinical efficacy and effectiveness of ursodeoxycholic acid in cholestatic liver diseases. Curr Clin Pharmacol. 2007;2(2):155–177. doi:10.2174/157488407780598171.
- Lajczak-Mcginley NK, Porru E, Fallon CM, Smyth J, Curley C, Mccarron PA, Tambuwala MM, Roda A, Keely SJ. The secondary bile acids, ursodeoxycholic acid and lithocholic acid, protect against intestinal inflammation by inhibition of epithelial apoptosis. Physiol Rep. 2020;8(12):e14456. doi:10.14814/phy2.14456.
- Alemi F, Poole DP, Chiu J, Schoonjans K, Cattaruzza F, Grider JR, Bunnett NW, Corvera CU. The receptor TGR5 mediates the prokinetic actions of intestinal bile acids and is required for normal defecation in mice. Gastroenterology. 2013;144(1):145–154. doi:10.1053/j.gastro.2012.09.055.
- Amaral JD, Viana RJ, Ramalho RM, Steer CJ, Rodrigues CM. Bile acids: regulation of apoptosis by ursodeoxycholic acid. J Lipid Res. 2009;50(9):1721–1734. doi:10.1194/jlr.R900011-JLR200.
- Lin Q, Tan X, Wang W, Zeng W, Gui L, Su M, Liu C, Jia W, Xu L, Lan K. Species differences of bile acid redox metabolism: tertiary oxidation of deoxycholate is conserved in preclinical animals. Drug Metab Dispos. 2020;48(6):499–507. doi:10.1124/dmd.120.090464.
- Gupta B, Liu Y, Chopyk DM, Rai RP, Desai C, Kumar P, Farris AB, Nusrat A, Parkos CA, Anania FA, et al. Western diet-induced increase in colonic bile acids compromises epithelial barrier in nonalcoholic steatohepatitis. FASEB J. 2020;34(5):7089–7102. doi:10.1096/fj.201902687R.
- Tan Q, Liu Y, Deng X, Chen J, Tsai PJ, Chen PH, Ye M, Guo J, Su Z. Autophagy: a promising process for the treatment of acetaminophen-induced liver injury. Arch Toxicol. 2020;94(9):2925–2938. doi:10.1007/s00204-020-02780-9.
- Panzitt K, Jungwirth E, Krones E, Lee J, Pollheimer M, Thallinger G, Kolb-Lenz D, Xiao R, Thorell A, Trauner M, et al. FXR-dependent Rubicon induction impairs autophagy in models of human cholestasis. J Hepatol. 2020;72(6):1122–1131. doi:10.1016/j.jhep.2020.01.014.
- Camp JG, Frank CL, Lickwar CR, Guturu H, Rube T, Wenger AM, Chen J, Bejerano G, Crawford GE, Rawls JF. Microbiota modulate transcription in the intestinal epithelium without remodeling the accessible chromatin landscape. Genome Res. 2014;24(9):1504–1516. doi:10.1101/gr.165845.113.
- Fiorucci S, Distrutti E. Bile acid-activated receptors, intestinal microbiota, and the treatment of metabolic disorders. Trends Mol Med. 2015;21(11):702–714. doi:10.1016/j.molmed.2015.09.001.
- Arab JP, Arrese M, Shah VH. Gut microbiota in non-alcoholic fatty liver disease and alcohol-related liver disease: current concepts and perspectives. Hepatol Res. 2020;50(4):407–418. doi:10.1111/hepr.13473.
- Aron-Wisnewsky J, Vigliotti C, Witjes J, Le P, Holleboom AG, Verheij J, Nieuwdorp M, Clément K. Gut microbiota and human NAFLD: disentangling microbial signatures from metabolic disorders. Nat Rev Gastroenterol Hepatol. 2020;17(5):279–297. doi:10.1038/s41575-020-0269-9.
- Ronis MJ, Mercer KE, Shankar K, Pulliam C, Pedersen K, Ingelman-Sundberg M, Friso S, Samuelson D, Del Valle L, Taylor C, et al. Potential role of gut microbiota, the proto-oncogene PIKE (Agap2) and cytochrome P450 CYP2W1 in promotion of liver cancer by alcoholic and nonalcoholic fatty liver disease and protection by dietary soy protein. Chem Biol Interact. 2020;325:109131. doi:10.1016/j.cbi.2020.109131.
- Zhang X, Coker OO, Chu ES, Fu K, Lau HCH, Wang YX, Chan AWH, Wei H, Yang X, Sung JJY, et al. Dietary cholesterol drives fatty liver-associated liver cancer by modulating gut microbiota and metabolites. Gut. 2021;70(4):761–774. doi:10.1136/gutjnl-2019-319664.
- Chen ML, Yi L, Zhang Y, Zhou X, Ran L, Yang J, Zhu JD, Zhang QY, Mi MT. Resveratrol attenuates Trimethylamine-N-Oxide (TMAO)-Induced atherosclerosis by regulating TMAO synthesis and bile acid metabolism via remodeling of the gut microbiota. mBio. 2016;7(2):e02210–15. doi:10.1128/mBio.02210-15.
- Liu Y, Chen J, Tan Q, Deng X, Tsai PJ, Chen PH, Ye M, Guo J, Su Z. Nondigestible oligosaccharides with anti-obesity effects. J Agric Food Chem. 2020;68(1):4–16. doi:10.1021/acs.jafc.9b06079.
- Zhu Y, Zhang JY, Wei YL, Hao JY, Lei YQ, Zhao WB, Xiao YH, Sun AD. The polyphenol-rich extract from chokeberry (Aronia melanocarpa L.) modulates gut microbiota and improves lipid metabolism in diet-induced obese rats. Nutr Metab (Lond). 2020;17:54. doi:10.1186/s12986-020-00473-9.
- Wei M, Huang F, Zhao L, Zhang Y, Yang W, Wang S, Li M, Han X, Ge K, Qu C, et al. A dysregulated bile acid-gut microbiota axis contributes to obesity susceptibility. EBioMedicine. 2020;55:102766. doi:10.1016/j.ebiom.2020.102766.
- Wang J, Wang P, Li D, Hu X, Chen F. Beneficial effects of ginger on prevention of obesity through modulation of gut microbiota in mice. Eur J Nutr. 2019;59(2):699–718. doi:10.1007/s00394-019-01938-1.
- Friedman ES, Li Y, Shen TCD, Jiang J, Chau L, Adorini L, Babakhani F, Edwards J, Shapiro D, Zhao C, et al. FXR-dependent modulation of the human small intestinal microbiome by the bile acid derivative obeticholic acid. Gastroenterology. 2018;155(6):1741–1752.e5. doi:10.1053/j.gastro.2018.08.022.
- Lenicek M, Olde Damink SWM, Schaap FG. Gut microbes take it to the next level? First insights into FXR agonists of microbial origin. Hepatology. 2020;72(4):1483–1485. doi:10.1002/hep.31455.
- Liu L, Liu Z, Li H, Cao Z, Li W, Song Z, Li X, Lu A, Lu C, Liu Y. Naturally occurring TPE-CA maintains gut microbiota and bile acids homeostasis via FXR signaling modulation of the liver-gut axis. Front Pharmacol. 2020;11:12. doi:10.3389/fphar.2020.00012.
- Ovadia C, Perdones-Montero A, Fan HM, Mullish BH, Mcdonald J, Papacleovoulou G, Wahlstrom A, Stahlman M, Tsakmaki A, Clarke LCD, et al. Ursodeoxycholic acid enriches intestinal bile salt hydrolase-expressing Bacteroidetes in cholestatic pregnancy. Sci Rep. 2020;10(1):3895. doi:10.1038/s41598-020-60821-w.
- Jose S, Mukherjee A, Horrigan O, Setchell KDR, Zhang W, Moreno-Fernandez ME, Andersen H, Sharma D, Haslam DB, Divanovic S, et al. Obeticholic acid ameliorates severity of Clostridioides difficile infection in high fat diet-induced obese mice. Mucosal Immunol. 2021;14(2):500–510. doi:10.1038/s41385-020-00338-7.
- Tian Y, Gui W, Koo I, Smith PB, Allman EL, Nichols RG, Rimal B, Cai J, Liu Q, Patterson AD. The microbiome modulating activity of bile acids. Gut Microbes. 2020;11:979–996. doi:10.1080/19490976.2020.1732268.
- Hoofnagle JH. FXR agonists as therapy for liver disease. Hepatology. 2020;72(1):1–3. doi:10.1002/hep.31265.
- Yoshimoto S, Loo TM, Atarashi K, Kanda H, Sato S, Oyadomari S, Iwakura Y, Oshima K, Morita H, Hattori M, et al. Obesity-induced gut microbial metabolite promotes liver cancer through senescence secretome. Nature. 2013;499(7456):97–101. doi:10.1038/nature12347.
- Bernstein C, Holubec H, Bhattacharyya AK, Nguyen H, Payne CM, Zaitlin B, Bernstein H. Carcinogenicity of deoxycholate, a secondary bile acid. Arch Toxicol. 2011;85(8):863–871. doi:10.1007/s00204-011-0648-7.
- Liang LM, Zhou JJ, Xu F, Liu PH, Qin L, Liu L, Liu XD. Diabetes downregulates peptide transporter 1 in the rat jejunum: possible involvement of cholate-induced FXR activation. Acta Pharmacol Sin. 2020. doi:10.1038/s41401-020-0408-4.
- Modica S, Murzilli S, Salvatore L, Schmidt DR, Moschetta A. Nuclear bile acid receptor FXR protects against intestinal tumorigenesis. Cancer Res. 2008;68(23):9589–9594. doi:10.1158/0008-5472.Can-08-1791.
- Kong B, Zhu Y, Li G, Williams JA, Buckley K, Tawfik O, Luyendyk JP, Guo GL. Mice with hepatocyte-specific FXR deficiency are resistant to spontaneous but susceptible to cholic acid-induced hepatocarcinogenesis. Am J Physiol Gastrointest Liver Physiol. 2016;310(5):G295–302. doi:10.1152/ajpgi.00134.2015.
- Absil L, Journe F, Larsimont D, Body JJ, Tafforeau L, Nonclercq D. Farnesoid X receptor as marker of osteotropism of breast cancers through its role in the osteomimetism of tumor cells. BMC Cancer. 2020;20(1):640. doi:10.1186/s12885-020-07106-7.
- Sharma NK, Sarode SC, Sarode GS, Patil S, Pal JK. Dietary choices modulate colorectal cancer stem cells: a role of FXR nuclear receptor. Nutr Cancer. 2020;1–8. doi:10.1080/01635581.2020.1792949.
- Gadaleta RM, Garcia-Irigoyen O, Moschetta A. Bile acids and colon cancer: is FXR the solution of the conundrum? Mol Aspects Med. 2017;56:66–74. doi:10.1016/j.mam.2017.04.002.
- Sokol H, Seksik P, Furet J, Firmesse O, Nion-Larmurier I, Beaugerie L, Cosnes J, Corthier G, Marteau P, Doré J. Low counts of Faecalibacterium prausnitzii in colitis microbiota. Inflamm Bowel Dis. 2009;15(8):1183–1189. doi:10.1002/ibd.20903.
- Thomas C, Pellicciari R, Pruzanski M, Auwerx J, Schoonjans K. Targeting bile-acid signalling for metabolic diseases. Nat Rev Drug Discov. 2008;7(8):678–693. doi:10.1038/nrd2619.
- Bernstein H, Bernstein C, Payne CM, Dvorak K. Bile acids as endogenous etiologic agents in gastrointestinal cancer. World J Gastroenterol. 2009;15(27):3329–3340. doi:10.3748/wjg.15.3329.
- Ajouz H, Mukherji D, Shamseddine A. Secondary bile acids: an underrecognized cause of colon cancer. World J Surg Oncol. 2014;12:164. doi:10.1186/1477-7819-12-164.
- Bingham SA, Day NE, Luben R, Ferrari P, Slimani N, Norat T, Clavel-Chapelon F, Kesse E, Nieters A, Boeing H, et al. Dietary fibre in food and protection against colorectal cancer in the European Prospective Investigation into Cancer and Nutrition (EPIC): an observational study. Lancet. 2003;361(9368):1496–1501. doi:10.1016/s0140-6736(03)13174-1.
- Fu T, Coulter S, Yoshihara E, Oh TG, Fang S, Cayabyab F, Zhu Q, Zhang T, Leblanc M, Liu S, et al. FXR regulates intestinal cancer stem cell proliferation. Cell. 2019;176(5):1098–1112.e18. doi:10.1016/j.cell.2019.01.036.