ABSTRACT
The gastrointestinal (GI) tract is the reservoir for multidrug resistant (MDR) pathogens, specifically carbapenem-resistant (CR) Klebsiella pneumoniae and other Enterobacteriaceae, which often lead to the spread of antimicrobial resistance genes, severe extraintestinal infections, and lethal outcomes. Selective GI decolonization has been proposed as a new strategy for preventing transmission to other body sites and minimizing spreading to susceptible individuals. Here, we purify the to-date uncharacterized class IIb microcin I47 (MccI47) and demonstrate potent inhibition of numerous Enterobacteriaceae, including multidrug-resistant clinical isolates, in vitro at concentrations resembling those of commonly prescribed antibiotics. We then genetically modify the probiotic bacterium Escherichia coli Nissle 1917 (EcN) to produce MccI47 from a stable multicopy plasmid by using MccI47 toxin production in a counterselection mechanism to engineer one of the native EcN plasmids, which renders provisions for inducible expression and plasmid selection unnecessary. We then test the clinical relevance of the MccI47-producing engineered EcN in a murine CR K. pneumoniae colonization model and demonstrate significant MccI47-dependent reduction of CR K. pneumoniae abundance after seven days of daily oral live biotherapeutic administration without disruption of the resident microbiota. This study provides the first demonstration of MccI47 as a potent antimicrobial against certain Enterobacteriaceae, and its ability to significantly reduce the abundance of CR K. pneumoniae in a preclinical animal model, when delivered from an engineered live biotherapeutic product. This study serves as the foundational step toward the use of engineered live biotherapeutic products aimed at the selective removal of MDR pathogens from the GI tract.
Introduction
Modern health care is challenged by the increasing emergence of multidrug resistant (MDR) pathogens, including carbapenem-resistant (CR) Enterobacteriaceae, which are responsible for millions of infections, tens of thousands of deaths, and billions of dollars in health-care costs every year.Citation1–3 In 2017, the World Health Organization (WHO) released a list of bacteria, for which new antibiotics are urgently needed, and considered CR Enterobacteriaceae including Klebsiella pneumoniae, Escherichia coli, Salmonella enterica, or Enterobacter cloaceae of the highest priority.Citation4 While K. pneumoniae is not known to cause gastrointestinal (GI) disease, its stable colonization of the intestine is the main reservoir for infections and transmission.Citation5 MDR Klebsiella species are responsible for outbreaks in nursing homes and long-term care facilitiesCitation6 and for spreading antibiotic resistance among other members of the GI microbiota.Citation7,Citation8 Moreover, GI colonization by certain K. pneumoniae contributes to the development of inflammatory bowel diseaseCitation9 and fatty acid liver diseaseCitation10, making its selective removal a desireable milestone in modern medicine.Citation11 However, the paucity of treatment options for the elimination of CR K. pneumoniae begs for the development of new strategies to eradicate it from the GI tract.
The human gut microbiome is a densely populated ecosystem, where our bacterial symbionts are in constant competition for survival.Citation12 A major strategy employed by bacteria to outcompete their neighbors is the production of highly specific antimicrobial peptides, so called bacteriocins, that target closely related species or strains.Citation13 While small molecule antimicrobials, such as penicillinCitation14, have been exploited for decades as traditional antibiotics, antimicrobial peptides as therapeutic agents have just recently gained widespread interest as potential treatments of MDR pathogens.Citation15,Citation16 Siderophore antimicrobial peptides (sAMPs), including class IIb microcins, are ribosomally synthesized and post-translationally modified peptides (RiPPs) consisting of an antimicrobial peptide (AMP) linked to an iron-chelating molecule, a siderophore (e.g., enterobactin).Citation17 Several studies have pointed to class IIb microcins as possible players capable of modulating the colonization dynamics of pathogenic Enterobacteriaceae in the GI tractCitation18–21, however, to date only three of them (MccM, MccH47, MccE492) have been characterized in detail.Citation22–25 MccI47 from E. coli strains H47 and CA46 has been identified based on sequence homology to known sAMPs, but it has never been overexpressed heterologously, purified, and no data demonstrating antimicrobial activity have been presented before.Citation26 In this study, we first leverage our recently established pipeline for sAMPs purificationCitation20,Citation21 to provide the first ever characterization of MccI47 as a potent antimicrobial against multiple Enterobacteriaceae. We then perform rational genetic manipulation of the probiotic E. coli Nissle 1917 (EcN) by engineering one of its two native plasmids to produce MccI47 without the need of any selection and demonstrate the ability of this new EcN strain in reducing CR K. pneumoniae abundance in vivo.
Results
To expand the library of potent antimicrobials against MDR Enterobacteriaceae, we overexpressed MccI47 heterologously in E. coli and tested its inhibitory activity against a collection of enteric bacteria, including MDR clinical isolates. Specifically, we created a plasmid that contained the structural microcin (mciA) and immunity (mciI) gene under arabinose induction, followed by the genes necessary for posttranslational modification with monoglycosylated enterobactin (MGE) mchCDEFA (as inCitation20) (). Highlighting the differences in activity spectrum and efficacy, we directly compared the potency of MccI47 with MccH47 (Figure S1), a well-characterized sAMP that has been used in previous proof-of-concept engineered biotherapeutics against Salmonella.Citation20,Citation21 Using static inhibition assays, we demonstrate that both microcins have strong inhibitory activity against extended-spectrum beta-lactamase (ESBL) producing E. coli (BAA 196). Intriguingly, MccI47 also inhibits the growth of CR K. pneumoniae (BAA 1705), while MccH47 has no effect (). We purified MccI47 with a maltose-binding protein (MBP)-microcin fusion proteinCitation20 and direct comparison of MccH47 and MccI47 in equal quantities reproduced the results obtained using the live-producing bacteria, highlighting MccI47ʹs efficacy against CR K. pneumoniae (BAA 1705) (). We next assessed the potency of MccI47 in minimum inhibitory concentration (MIC) assays against a panel of 20 different target strains (). Overall, MccI47ʹs potency is comparable to that of commonly prescribed broad-spectrum antibiotics, while being highly specific for members of the Enterobacteriaceae family.Citation27 Compared to MccH47, MccI47 is approximately three-times more effective against various E. coli strains (0.2–0.6 µM), four-times more effective against Shigella flexneri (0.06 µM), and seven-times more effective against Salmonella Typhimurium (0.9–1.7 µM). More importantly, MccI47 but not MccH47 demonstrates activity against Enterobacter cloacae (19.5 µM), Serratia marcescens (15.1 µM), and Klebsiella pneumoniae (2.0–5.1 µM). Interestingly, neither of the two toxins showed activity against Klebsiella oxytoca or any tested bacteria outside of the Enterobacteriaceae family. We did not detect any difference in potency in targets carrying MDR genes, which is likely because the mode of entry of MccI47 into the target cells is independent of the resistance mechanisms of commonly used antibiotics.Citation28 Taken together, these data demonstrate the discovery of MccI47 as a new potent AMP with selective activity against several Enterobacteriaceae members, including a strong inhibitory effect against CR K. pneumoniae representatives.
Table 1. Results of minimum inhibitory concentration (MIC) assays of purified MccI47 against bacterial isolates, including MDR Enterobacteriaceae in comparison to MccH47.Citation20 Asterisks indicate MDR strains.
Figure 1. MccI47 is a potent inhibitor of MDR Klebsiella pneumoniae. (a) Plasmid map of pBBAD-I47, a pUC19-based plasmid producing MccI47-MGE from mciA, the immunity peptide mciI as well as the genes needed for post-translational modification mchCDEFA. (b) Static inhibition assays comparing MccH47 and MccI47 activity from single colony production against multidrug-resistant Escherichia coli (top) and Klebsiella pneumoniae (bottom). The control strain (ctrl) harbors the same backbone plasmid (pUC19) without microcin production. (c) Static inhibition assays comparing purified MccH47 and MccI47 activity against multidrug-resistant Escherichia coli (top) and Klebsiella pneumoniae (bottom). After purification, 3.5 µg of each microcin were dried on the media before the overlay with the target bacteria. Scale bars: 1 cm.
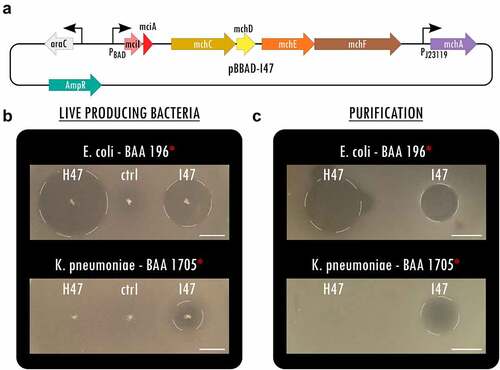
Due to the potent inhibitory effect against K. pneumoniae observed in vitro, we hypothesized that MccI47 could be used as a novel narrow-spectrum antimicrobial to reduce CR K. pneumoniae colonization in the GI tract in a murine model. We therefore developed an engineered EcN strain with MccI47-production from a native, modified multicopy plasmid, allowing for long-term plasmid retention without the need of selection. We chose EcN as vehicle strain because it has been used in its wildtype form as a live probiotic supplement for several human diseases for over a centuryCitation29, carries genomic islands that promote anti-inflammatory responsesCitation30, and was found to be highly refractory to exogenous plasmid acquisition.Citation31 EcN is used for the construction of genetically engineered bacteriotherapeutic agents, which are being tested in multiple ongoing clinical trials.Citation32
While recent studies relied on chromosomal integrationCitation33,Citation34, we sought to maximize MccI47 output using a multicopy plasmidCitation21,Citation35,Citation36 and developed an expression system using the native, self-retaining EcN plasmid pMut2 as backbone for constitutive heterologous protein expression.Citation36 We first cured EcN from pMut2 by utilizing MccI47 (mciA) in a counterselection approach (). For the curing plasmid pCure2-I47 (Figure S2A), we employed pMut2 as backbone for plasmid competition, while harboring a chloramphenicol resistance, and placing mciA without its signal peptide for secretion and the protective immunity gene under isopropyl β-d-1-thiogalactopyranoside (IPTG) induction. Strong antibiotic selection allowed us to first displace pMut2, before inducing mciA expression and thus cell death in cells harboring pCure2-I47 ().
Figure 2. Stable MccI47 production from a native, self-retaining EcN plasmid. (a) Curing of the native EcN plasmid pMut2 via utilizing inducible MccI47 expression as a counterselection tool. Counterselection plasmid pCure2-I47 with pMut2 as backbone was introduced into EcN by electroporation and chloramphenicol (Cm) supplementation. High concentrations of Cm led to complete displacement of pMut2 in the cells. Without further antibiotic selection, mciA expression was induced, leading to selection against pCure2-I47, since the immunity peptide mciI was omitted in the plasmid design. (b) Plasmid map of pMut2-I47, an MccI47-producing plasmid with pMut2 as backbone vector. (c) Serial passage experiment to assess pMut2-I47 plasmid retention in EcNΔH ΔM-I47. Cells were initially grown with the supplementation of ampicillin and then subjected to daily subculturing for ten days without selection pressure, resulting in a total of approximately 200 bacterial generations. n = 5. (d) Assessment of plasmid copy number of pMut2 and the pMut2-based pMut2-I47 using quantitative PCR. Note that EcNΔH ΔM-I47 cells harboring pMut2-I47 exhibit a significantly higher copy number mean compared to EcN harboring native pMut2. Additionally, both plasmids are stably retained for the ten days of the serial passage experiment. ***: p < .001. n = 5. (e) Static inhibition assay comparing inhibitory activity of (1) EcN, (2) EcNΔH ΔM, and (3) EcNΔH ΔM-I47, harboring pMut2-I47, against carbapenem-resistant Klebsiella pneumoniae. Inhibitory activity of EcNΔH ΔM-I47 is independent ampicillin (Amp) for plasmid selection. Scale bar: 1 cm.
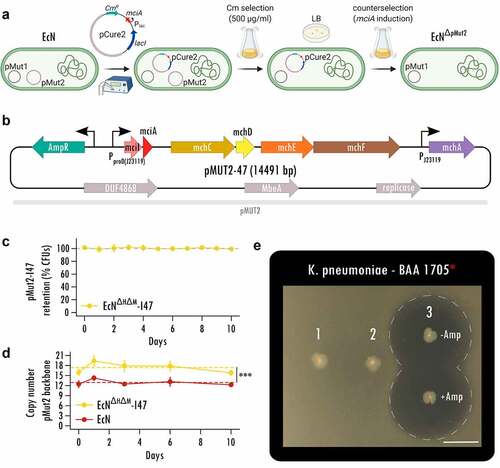
The resulting cells cured of pMut2 and pCure2-I47 (Figure S2B, Table S1) were then transformed with the MccI47-producing plasmid (pMut2-I47) for in vivo applicability (). pMut2-I47 contains the same genetic machinery for MccI47 production and export as pBBAD-I47 (); however, it uses a proD-likeCitation37 insulated strong constitutive promoter (J23119) for mciA/mciI expression for maximum microcin output in vivo. Further, the pMut2 backbone allows plasmid retention without selection pressure. Since wildtype EcN harbors genes for the microcins MccM (mcmIA) and MccH47 (mchIB), we generated an EcN MccH47/MccM knockout strain (EcNΔH ΔM) to exclude potential interference by these microcins in the downstream experiments (Figure S3, Figure S4). Therefore, the following strains were used in the remaining set of experiments: (i) EcN, (ii) EcNΔH ΔM, and (iii) EcNΔH ΔM-I47, with the latter harboring pMut2-I47.
Since pMut2-I47 (14491 bp) is 2.6-times larger than the native pMut2 (5514 bp), we evaluated pMut2-I47ʹs retention in a serial passage experiment without antibiotic selection for 10 consecutive days and approximately 200 generations in total. We did not observe a reduction in colony forming units (CFUs) carrying the plasmid (linear mixed effect modeling, p value > .05; ) but intriguingly record a higher plasmid copy number for EcNΔH ΔM-I47 (mean ± SD, 17.4 ± 1.5) compared to EcN (12.9 ± 0.8) as estimated by quantitative PCR (linear mixed effect modeling, p value < .001; ), possibly caused by MccI47 accumulation during the growth phase. This suggests that even though we increased the plasmid size 2.6-fold, pMut2-I47 is retained stably long-term without the need of selection. We then confirmed EcNΔH ΔM-I47 killing activity using static inhibition assays. EcNΔH ΔM-I47 produced a zone of inhibition of more than 2.3 cm in diameter against CR K. pneumoniae (BAA 1705) irrespective of the addition of ampicillin for antibiotic plasmid retention (). Simultaneously, the two respective controls EcN (1) and EcNΔH ΔM (2) were unable to produce any halo of inhibition. Taken together, these data provide the evidence for a novel EcN strain engineered to constitutively produce MccI47 from a self-retaining plasmid in vitro, which is suitable for application in a murine model to challenge CR K. pneumoniae colonization.
We then evaluated MccI47ʹs ability to reduce gastrointestinal carriage of CR K. pneumoniae by administration of EcNΔH ΔM-I47 in vivo and performed a decolonization experiment in a specific-pathogen-free mouse model, as described in.Citation38–40 Briefly, we treated C57BL/6J mice with ampicillin for 7 days to disrupt the resident microbiome, before challenging them with 108 cells of CR K. pneumoniae (BAA 1705), for which we had demonstrated MccI47-mediated inhibitory effects in , , and . One week after CR K. pneumoniae application, each mouse was orally administered PBS or 108 cells of either EcN, EcNΔH ΔM or EcNΔH ΔM-I47 daily for 7 days (). Fecal samples were collected throughout the experiment and plated to quantify absolute abundances of CR K. pneumoniae. The decolonization efficacy was tested for differences between the treatment groups by running linear mixed effect modeling as described in detail in the Methods section. Daily administration of EcNΔH ΔM-I47 causes a significantly higher decay in CR K. pneumoniae abundance (measured by fecal shedding) compared to treatment with PBS alone (p = .0005) as well as treatment with the control strain EcNΔH ΔM (p = .042) (, Figure S5). Moreover, treatment with the wildtype EcN also causes a significant decline in CR K. pneumoniae abundance compared to PBS (p = .009) but not EcNΔH ΔM (p = .26) or EcNΔH ΔM-I47 (p = .37), supporting the previously described role of class IIb microcins in niche competition.Citation18 Importantly, when compared to PBS, EcNΔH ΔM-I47 (time:treatment interaction coefficient = −0.22) exhibits a stronger decolonization effect than EcN (time:treatment interaction coefficient = −0.16). To determine whether the treatments resulted in alterations of the resident microbiota, we analyzed the relative bacterial abundances before and after treatment through 16S rRNA profiling. Principal coordinates analysis of pre and post-treatment groups revealed significant community changes for all samples (PERMANOVA, p < .0001) likely caused by the time passed since the antibiotic treatment and the recovery of the resident microbiota (). However, we could not find any live biotherapeutic product-dependent changes in the community caused by the different EcN strains (PERMANOVA, p = .81). Differential analysis using LimmaVoom resulted in only three significantly different bacterial species, when comparing EcNΔH ΔM-I47 with the PBS group (Acetivibrio ethanolgignens, Mammaliicoccus lentus, Mammaliicoccus sciuri), which may be due to the use of sensitive statistical testing, since none of them displays consistent signal over most samples across the treatment groups (Figure S6). Therefore, we can conclude that in contrast to commonly prescribed antibiotics, treatment with the engineered strain has minimal effects on the GI microbiota, with the addition that our engineered biotherapeutic strain EcNΔH ΔM-I47 selectively targets CR K. pneumoniae. Taken together, these data demonstrate that the oral delivery of an engineered probiotic E. coli strain overproducing MccI47 while passing through the GI tract is a viable approach to reduce CR K. pneumoniae colonization in vivo.
Figure 3. Engineered probiotic expressing MccI47 is effectively reducing colonization of carbapenem-resistant Klebsiella pneumoniae in vivo. (a) Schematic representation of the experimental design. Mice were treated with ampicillin in the drinking water for seven days and infected with 108 cells of carbapenem-resistant K. pneumoniae (BAA 1705) . After one week of engraftment, the mice were treated daily with either PBS, or with 108 cells of the different EcN strains (EcN, EcNΔH ΔM and EcNΔH ΔM-I47) for seven days by oral administration. (b) Log2 fold change of K. pneumoniae colonization throughout the treatment with PBS or the respective EcN strain (EcN, EcNΔH ΔM and EcNΔH ΔM-I47). Linear mixed effect modeling was used to assess the change in K. pneumoniae (BAA 1705) colonization. *: p < .05, ***: p < .001. n = 16. (c) Principal coordinate analysis (PCoA) of the bacterial communities before and after treatment. Note that the experimental time introduces significant differences between the samples pre and post treatment. Ellipses are grouping samples according to time point pre and post treatment. (n = 8).
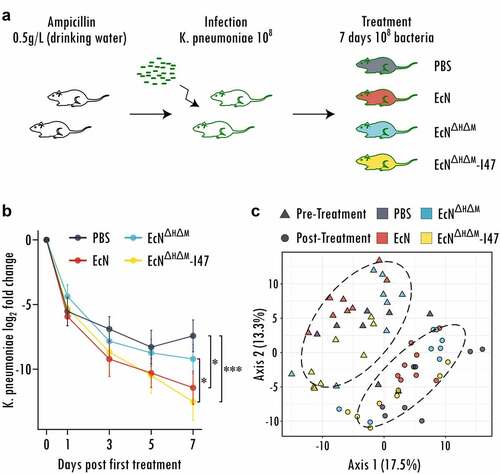
Discussion
Under the global societal challenge of moving toward more personalized medicine, biomedical research is currently exploring the use of engineered bacteria as vehicles for the targeted administration of therapeutic compounds at the location of disease and with the goal of reducing systemic side effects.Citation32,Citation33,Citation41 This is particularly relevant when the therapeutic objective is the delivery of protein-based agents (i.e., AMPs) to the GI tract as host digestive enzymes likely inactivate them before they reach their site of action.Citation42 Furthermore, embedding the proteins into a lipid matrix within enteric capsules to prevent host-mediated degradationCitation43, needs to account for the agent’s dilution effect that naturally occurs downstream of the point of capsule’s opening. These issues are circumvented with the use of live, engineered bacteria as they can be designed to ensure continuous production of the therapeutic compound while passing through the GI tract.Citation44
Current antibiotics are often applied systemically to treat bacterial pathogens, yet increasingly fail to clear infections caused by MDR organisms.Citation45 Additionally, these drugs also cause profound gastrointestinal dysbiosis of the protective microbiota, leaving treated individuals with often dangerous secondary outcomes.Citation46 Selective decolonization of pathogenic bacteria as a way to minimize their translocation and horizontal transmission to other patients is currently being explored in the pharmaceutical industry by assembling consortia of isolated bacteria that display decolonizing properties against certain species, including K. pneumoniae.Citation47,Citation48 However, to our knowledge, there is no live biotherapeutic for pathogen decolonization that has reached the stage of human trials.
Inspired by recent research on the role of siderophore microcins in modulating the dynamics of gastrointestinal bacteriaCitation49, we here provide the very first characterization of the previously putative class IIb microcin MccI47. We show that MccI47 is a potent antimicrobial against multiple MDR Enterobacteriaceae strains and displays strong inhibitory effect against CR K. pneumoniae. Based on this, we then develop a rationally engineered biotherapeutic E. coli Nissle 1917 that is capable of MccI47 overproduction. Compared to previous studies that either rely on genomic integrationCitation32 (which would lead to a single copy of the operon and thus suboptimal production) or rely on the use of plasmids that require a strong selective pressure for their maintenanceCitation50, we devised a novel constitutive expression system from a stably retained multicopy plasmid that allows for high MccI47 output, while rendering provisions for plasmid selection unnecessary. Finally, we demonstrate possible clinical relevance of our engineered MccI47-producing strain in an in vivo model of CR K. pneumoniae colonization. Specifically, we observe that MccI47 production provides a significant decline in intestinal CR K. pneumoniae abundance compared to the mock treatment or the control strain without microcin production after 7 days of daily oral probiotic administration, with no major effect on the resident microbiota. Selective reduction in gastrointestinal CR K. pneumoniae colonization, including hypervirulent strains, in symptomatic and asymptomatic carriers may decrease host-to-host transmission and thus ameliorate host pathogenesis in affected populations.
Overall, this study serves as the foundational step toward the use of engineered probiotics and engineered live biotherapeutic products aimed at the selective removal of MDR pathogens from the GI tract. We believe that this study will open the door to investigations into the optimization, scale-up and manufacturing of these next-generation therapeutic agents and possibly human trials.
Methods
Bacterial strains and plasmids
This study used the Escherichia coli strains NEB10β (New England Biolabs, Ipswich, MA), Nissle 1917 as well as all strains listed in . Strains in were purchased from ATCC (Manassas, VA). All plasmids in this study have been transformed into cells using electroporation with the Bio-Rad MicropulserTM (Bio-Rad Laboratories, Hercules, CA) and were created using Gibson AssemblyCitation51 using the Gibson Assembly Master Mix (New England Biolabs, Ipswich, MA) and custom DNA oligonucleotides purchased from Integrated DNA Technologies (Coralville, IA). For pBBAD-H47 and pBBAD-I47, four fragments were amplified by PCR and assembled: (1) linearized pUC19, (2) araC and PBAD from pTARACitation52 (Addgene #39491), (3) the microcin and immunity genes for MccH47 (mchXIB) or MccI47 (mciIA) originating from pEX2000Citation53 as well as (4) the genes mchCDEFA originating from pPP2000.Citation20 Plasmid pHMT-H47 has been described and used in.Citation20 For plasmid pHMT-I47 a total of seven fragments was assembled: (1) linearized pUC19, (2) chloramphenicol resistance cassette from pTARACitation52 (Addgene #39491), (3) lacI and tac promoter from pMAL-c5X (New England Biolabs, Ipswich, MA), (4) MBP, amplified using primers to add a 6× Histidine N-terminal tag, from pMAL-c5X, (5) mciA from pEX2000,Citation53 (6) mciI from pEX2000, and (7) mchCDEFA from pPP2000. To cure the native pMut2 plasmid from E. coli Nissle 1917, pCure2-I47 was assembled from four fragment: (1) linearized pMut2, (2) chloramphenicol resistance cassette from pTARACitation52 (Addgene #39491), (3) mciA from pEX2000,Citation53 (4) lacI and tac promoter from pMAL-c5X (New England Biolabs, Ipswich, MA). The modified replacement plasmid for pMut2, pMut2-I47, was created from four fragments: (1) linearized pMut2, (2) ampicillin resistance cassette from pUC19, (3) insulated promoter proD,Citation37 where the promoter region was replaced with the strong constitutive promoter J23119 (BBa_J23119), (4) mciIA to mchCDEFA from pBBAD-I47. All plasmid sequences and maps have been deposited as .dna files at: https://gitlab.com/vanni-bucci/2021_MccI47_paper. Chromosomal modifications were obtained through lambda Red recombination using pKD46 and FLP-FRT recombination using pCP20 as described by Datsenko and Wanner.Citation54 Briefly, a kanamycin resistance cassette flanked by flippase recognition target (FRT) sites was amplified by PCR from pKD4 and then transformed into the respective electrocompetent EcN strain harboring plasmid pKD46. For strain EcNΔH ΔM, the kanamycin resistance was removed after the knockout of mchIB by inducing the flippase from pKD20, before it was reintroduced for the mcmIA knockout. All modifications were confirmed using Sanger sequencing (Figure S3).
Plasmid curing and assessment of retention
To cure the native plasmid pMut2 from E. coli Nissle 1917, the inducible plasmid pCure2-I47 was created for counterselection. After transformation of pCure2-I47 into E. coli Nissle 1917, plasmid presence was confirmed by selective plating on chloramphenicol (25 µg/ml) and PCR. Positive clones were individually grown over night at 37°C under strong antibiotic selection (500 µg/ml) in liquid LB medium to skew plasmid competition toward pCure2-I47 and to displace pMut2. Resulting cultures were plated on LB agar, and the loss of pMut2 was confirmed by PCR (Table S1). Resulting clones were again individually grown over night at 37°C in LB with the addition of 0.5 mM IPTG. Since pCure2-I47 expresses the MccI47 toxin (mciA) without the immunity peptide (mciI) under IPTG induction, cells harboring pCure2-I47 are killed in response to the addition of IPTG and therefore forced to lose the plasmid to survive. Resulting cultures were plated on LB agar and loss of pMut2 and pCure2-I47 was confirmed by PCR (Figure S2B). Subsequently, pMut2-I47 was transformed into cured cells and retention without antibiotic selection was assessed by a serial passage experiment. Five independent replicates of EcNΔH ΔM-I47 were initially grown under ampicillin selection (100 µg/ml) overnight at 37°C in liquid LB medium (to day 0) and then subcultured 10−6 daily for 10 days (until day 10), allowing for the growth of approximately 20 bacterial generations per passage. Each biological replicate was plated daily on LB agar using three technical replicates with and without ampicillin (100 µg/ml) to quantify the percentage of CFUs retaining the plasmid over time. To test for the effect of time on the number of CFUs of EcNΔH ΔM-I47 retaining pMut2-I47 we ran linear mixed effect modeling in ‘R’ using the lmer from the lmerTest package. Specifically, we fit models predicting the percentage of CFUs having the pMut2-I47 plasmid as a function of time and using replicate ID as random effect. To assess the average plasmid’s copy number per bacterium, cells contained in 1 ml from each daily passed culture were harvested and frozen at −80°C until qPCR. To compare pMut2-I47 copy number with the copy number of the native pMut2, EcN was also subjected to the same serial passage assay and related procedures as control. Plasmid copy numbers have been assessed using qPCR by comparing the signal from the plasmid pMut2 to that from the chromosomal gene DNA gyrase subunit B (Table S1).Citation55 The frozen bacterial pellet was resuspended in 1 ml of sterile PBS, incubated for 5 min at 95°C and used as template in the qPCR reaction in a final 10−4 dilution with the iTaq Universal SYBR Green Supermix (Bio-Rad Laboratories, Hercules, CA). The qPCR was run in a StepOnePlus Real-Time PCR System (Applied Biosystems, Waltham, MA) and plasmid copy number was calculated using the ∆∆Ct, assuming an efficiency of 100%. To test for differences in the number of copies of pMut2-I47 (carried by EcNΔH ΔM-I47) versus the number of copies of pMut2 (carried by EcN) and the effect of time, we ran linear mixed effect modeling in ‘R’ using the lmer from the lmerTest package. Here, we fit models where the copy number was predicted as a function of the strain (i.e., EcNΔH ΔM-I47 vs. EcN), time and the interaction of the two and using replicate ID as random effect.
MccI47 purification
The MBP-MccI47 fusion protein was expressed from pHMT-I47 and purified as described in.Citation20 Briefly, overnight cultures of the E. coli NEB10β strain harboring pHMT-I47 were grown in LB under antibiotic selection (100 µg/ml ampicillin, 25 µg/ml chloramphenicol). The culture was diluted 1:50 in prewarmed LB medium and incubated at 200 rpm and 37°C. After 90 min 2,2-dipyridyl was added to a final concentration of 0.2 mM. After two more hours (approximate optical density at 600 nm OD600 = 0.2), MccI47 production was induced with 0.5 mM IPTG for 5 h. The cells were then harvested by centrifugation, resuspended in column buffer (200 mM NaCl, 20 mM Tris-HCl, pH 7.5) and frozen until purification at −20°C. The cells were slowly thawed in cold water, sonicated, and centrifuged at 4°C with 15,000xg for 10 min to remove the debris. The lysate was then passed through an amylose resin (New England Biolabs, Ipswich, MA) column and finally the MBP-MccI47 fusion proteins were eluted into 30 ml using 10 mM maltose in the column buffer. The MBP-MccI47-containing solution was concentrated using MilliporeSigma (Burlington, MA) MWCO 10,000 filters and then digested with 10 µl Tobacco etch virus nuclear-inclusion-a endopeptidase (TEV) (New England Biolabs, Ipswich, MA) overnight at 4°C. The next day, the solution was brought to room temperature, another 5 µl TEV were added, and the digestion was incubated for additional 2 h, resulting in free MccI47 in the solution. The histidine-tagged TEV and MBP were extracted from the solution using a Ni-NTA agarose resin (Qiagen, Hilden, Germany) as described in.Citation20
Plate inhibition assays
Solid media inhibition assays were performed as described previously.Citation20,Citation21 Briefly, individual bacterial colonies of the microcin-producing strains (MccH47 or MccI47) were picked with a sterile pipet tip and stabbed into the solid LB agar. Iron-limited conditions during the growth phase were created by supplementing the media with 0.2 mM 2,2-dipyridyl. For the inducible constructs pBBAD-MccH47 and pBBAD-MccI47, the media was supplemented with 100 µg/mL ampicillin for plasmid retention and 0.4% L-arabinose for microcin production. For the self-retaining plasmid pMut2-MccI47, no inducing agent was necessary. Here, were indicated, ampicillin was locally added to the media by drying a drop of sterile water containing 500 µg ampicillin before adding the colony. All plates were incubated for 48 h, and the bacteria were inactivated with chloroform vapors and 10 min of ultraviolet light. For the overlay, target bacteria were diluted from an overnight culture (E. coli BAA 196–1:200; K. pneumoniae BAA 1705–1:1000) in 3 ml LB with 0.2 mM 2,2-dipyridyl and 100 µg/mL ampicillin. Then, molten agar was added to the media to a final concentration of 0.75%. 3.5 ml of the soft agar medium was then evenly spread on the plate, cooled and the plate was incubated for 16 h at 37°C before imaging.
Minimum inhibitory concentration (MIC) assays
The MIC assays were carried out in sterile 96-well round bottom microplates (CELLTREAT Scientific Products, Pepperell, MA) and were set up with the following media. The first row was filled with 20 µl 2x LB with 0.4 mM 2,2’-dipyridyl and 20 µl of purified MccI47 in amylose resin elution buffer (200 mM NaCl, 20 mM Tris-HCl, 10 mM maltose, pH 7.5), resulting in the highest MccI47 concentration for the assay in 1x LB, 0.2 mM 2,2’-dipyridyl, 0.5× amylose resin elution buffer. All other wells were loaded with 20 µl 1x LB, 0.2 mM 2,2’-dipyridyl, 0.5× amylose resin elution buffer and two-fold serial dilution were performed across the plate. Target bacteria were grown over night in standard LB at 200 rpm at 37°C, and added to a final dilution of 10−4 into the individual wells. The plates were incubated in the dark at room temperature with gentle agitation, and MICs were determined as the lowest concentration for which no growth was observed after 24 h. All reported MIC values represent the median of at least three biological replicates from independent MccI47 purifications.
Animal study
The mouse experiment was carried out under an institutionally approved Institutional Animal Care and Use Committee (IACUC) protocol. Per arm 16 C57BL/6J mice (four male, twelve female) at 7 weeks of age were housed in same-sex pairs under specific pathogen-free conditions. Each mouse was individually marked, so they could be traced throughout the entire experiment. To achieve robust gastrointestinal CR Klebsiella pneumoniae (BAA 1705) engraftment, all mice were treated with 0.5 g/l ampicillin in the drinking water for 7 days. We confirmed that CR K. pneumoniae (BAA 1705) was not already present in the mice by selective plating of undiluted feces suspension at both time of arrival as well as on the morning right before infection. For infection, mice were deprived of food and water for 4 h and then given 108 cells of CR K. pneumoniae (BAA 1705) in 20 µl phosphate buffered saline (PBS) by pipet. After one week of engraftment, the four arms were given daily for 7 days either (i) PBS, or 108 cells days of either (ii) EcN, (iii) EcN with a knockout of the genes mchIB (MccH47) and mcmIA (MccM) to prevent interference of the other class IIb microcins in the EcN genome (EcNΔH ΔM), or (iv) EcN with the same gene knockouts and constitutive MccI47-production from a modified version of the native plasmid pMut2 (EcNΔH ΔM-I47). For administration, the mice were again deprived of food and water for 4 h, then given PBS alone or the respective cells orally by pipet in 20 µl PBS, and transferred to a clean cage after the first treament. Fecal samples were taken before the first administration (day 0), day 1, day 3, day 5, and day 7 after the first treatment to assess the colonization of CR K. pneumoniae (BAA 1705). For sampling, mice were placed into sterile isolation containers and at least two fecal samples (approximately 0.03 g each) were collected into sterile two 1.5 ml microcentrifuge tubes. One sample was snapfrozen and stored at −80°C until DNA extraction, the other one was weighed, resuspended in sterile PBS and kept on ice until plating. To determine the bacterial shedding in CFU/g feces, the samples were shortly spun in a minicentrifuge to pellet larger particles and plated in 10-fold serial dilutions from the supernatant on respective antibiotic plates and incubated at 37°C for 16 h, which resulted in a detection limit at 102 CFU/g feces. To compare the effect of treatment on the CR K. pneumoniae reduction rate, we ran linear mixed effect modeling of the form, log10(CFU counts) ~ Time (Days) + Treatment + Time:Treatment + 1|MouseID, using MouseID as random effect. The model was fitted to the data using the lmer function from the lmerTestCitation56 package in ‘R’. The interaction term “Time:Treatment” was inspected to determine significant differences in CR K. pneumoniae reduction rate across treatments. Significance and magnitude of interaction coefficients were inspected to determine differences among the treatment type. Data and ‘R’ code to perform statistics have been deposited at: https://gitlab.com/vanni-bucci/2021_MccI47_paper.
Relative bacterial abundance analyses
DNA was extracted from frozen fecal pellets with the DNeasy Powersoil Pro Kit by Qiagen (Hilden, Germany) according to the manufacturer’s protocol. The bacterial 16S rRNA gene (variable regions V3 to V4) was subjected to PCR amplification using the using the universal 341 _F and 806 _R barcoded primers for Illumina sequencing. Using the SequalPrep Normalization kit, the products were pooled into sequencing libraries in equimolar amounts and sequenced on the Illumina MiSeq platform using v3 chemistry for 2 × 300 bp reads. The forward and reverse amplicon sequencing reads were dereplicated and sequences were inferred using dada2.Citation57 To test for treatment-specific differences compared to EcNΔH ΔM-I47, for every species, we ran linear mixed modeling of the form, Species (normalized counts)∼Time (Day 0 |Day 7) + Treatment + Time:Treatment +1|MouseID with MouseID as random effect using Limma/voom as in.Citation58, Species were determined to be significantly different (Benjamini Hochberg adjusted p-value < 0.05) for the interaction term “Time:Treatment” from the model. All ‘R’ code has been deposited at: https://gitlab.com/vanni-bucci/2021_MccI47_paper. Raw microbiome data have been deposited on the European Sequencing Archive (ENA) accession number PRJEB48537.
Author contribution
B.M.M, J.D.P, V.B, M.W.S and B.A.M conceptualized the study. B.M.M, J.D.P, V.B designed the experiments. B.M.M and J.D.P performed initial engineering and testing of MccI47-MBP expressing strains. B.M.M and H.D performed purification and in vitro minimum inhibitory concentration assays. B.M.M conceptualized and performed EcN stable engineering and testing. B.M.M and R.ML performed in vivo experiments. C.B performed microbiome sequencing. S.K.B performed microbiome analytics. V.B and B.M.M performed statistical testing and data interpretation. M.W.S and B.A.M supported with data interpretation and with microbiological and infectious disease expertise. B.M.M and V.B wrote the manuscript with input of all authors.
Supplemental Material
Download MS Word (1.1 MB)Acknowledgments
We appreciate the help and microbiological expertise by Kenan Murphy. was created with BioRender.com. Figure S3 was created using EasyfigCitation59
Data availability statement
All data and code to create the figures of this study as well as DNA files for genetic engineering work are available at https://gitlab.com/vanni-bucci/2021_MccI47_paper. Raw reads for the 16S rRNA amplicon sequencing are deposited on the European Sequencing Archive (ENA) under accession number PRJEB48537. Plasmids and strains are available from the authors upon request.
Disclosure statement
V.B. receives support from a sponsored research agreement from Vedanta Biosciences, Inc..
Supplementary material
Supplemental data for this article can be accessed online at https://doi.org/10.1080/19490976.2022.2127633
Additional information
Funding
References
- Kadri SS. Key takeaways from the U.S. CDC’s 2019 antibiotic resistance threats report for frontline providers. Crit Care Med. 2020;48(7):939–14. doi:10.1097/CCM.0000000000004371.
- Cassini A, Högberg LD, Plachouras D, Quattrocchi A, Hoxha A, Simonsen GS, Colomb-Cotinat M, Kretzschmar ME, Devleesschauwer B, Cecchini M, et al. Attributable deaths and disability-adjusted life-years caused by infections with antibiotic-resistant bacteria in the EU and the European economic area in 2015: a population-level modelling analysis. Lancet Infect Dis. 2019;19(1):56–66. doi:10.1016/S1473-3099(18)30605-4.
- Bartsch SM, McKinnell JA, Mueller LE, Miller LG, Gohil SK, Huang SS, Lee BY. Potential economic burden of carbapenem-resistant enterobacteriaceae (CRE) in the United States. Clin Microbiol Infect. 2017;23(1):48.e9–.e16. doi:10.1016/j.cmi.2016.09.003.
- Tacconelli E, Carrara E, Savoldi A, Harbarth S, Mendelson M, Monnet DL, Pulcini C, Kahlmeter G, Kluytmans J, Carmeli Y, et al. Discovery, research, and development of new antibiotics: the WHO priority list of antibiotic-resistant bacteria and tuberculosis. Lancet Infect Dis. 2018;18(3):318–327. doi:10.1016/s1473-3099(17)30753-3.
- Gorrie CL, Mirceta M, Wick RR, Edwards DJ, Thomson NR, Strugnell RA, Pratt NF, Garlick JS, Watson KM, Pilcher DV, et al. Gastrointestinal carriage is a major reservoir of Klebsiella pneumoniae infection in intensive care patients. Clin Infect Dis. 2017;65(2):208–215. doi:10.1093/cid/cix270.
- World Health O. Antimicrobial resistance: global report on surveillance. Geneva: World Health Organization; 2014.
- Jacoby GA, Strahilevitz J, Hooper DC, Tolmasky M, Alonso JC, Tolmasky M, Alonso JC. Plasmid-mediated quinolone resistance. Microbiology Spectrum. 2014;2(5):2.5.33. doi:10.1128/microbiolspec.PLAS-0006-2013.
- Wong MHY, Chan EWC, Evolution CS. Dissemination of OqxAB-Like efflux pumps, an emerging quinolone resistance determinant among members of enterobacteriaceae. Antimicrob Agents Chemother. 2015;59(6):3290–3297. doi:10.1128/AAC.00310-15.
- Atarashi K, Suda W, Luo C, Kawaguchi T, Motoo I, Narushima S, Kiguchi Y, Yasuma K, Watanabe E, Tanoue T, et al. Ectopic colonization of oral bacteria in the intestine drives T H 1 cell induction and inflammation. Science. 2017;358(6361):359–365. doi:10.1126/science.aan4526.
- Yuan J, Chen C, Cui J, Lu J, Yan C, Wei X, Zhao X, Li N, Li S, Xue G, et al. Fatty liver disease caused by high-alcohol-producing Klebsiella pneumoniae. Cell Metab. 2019;30(4):675–88.e7. doi:10.1016/j.cmet.2019.08.018.
- Septimus EJ, Schweizer ML. Decolonization in prevention of health care-associated infections. Clin Microbiol Rev. 2016;29(2):201–222. doi:10.1128/cmr.00049-15.
- Foster KR, Schluter J, Coyte KZ, Rakoff-Nahoum S. The evolution of the host microbiome as an ecosystem on a leash. Nature. 2017;548(7665):43–51. doi:10.1038/nature23292.
- Granato ET, Meiller-Legrand TA, Foster KR. The evolution and ecology of bacterial warfare. Current Biol. 2019;29(11):R521–R37. doi:10.1016/j.cub.2019.04.024.
- Fleming A. On the antibacterial action of cultures of a penicillium, with special reference to their use in the isolation of B. influenzæ Br J Exp Pathol. 1929;10:226–236.
- Fjell CD, Hiss JA, Hancock REW, Schneider G. Designing antimicrobial peptides: form follows function. Nat Rev Drug Discov. 2012;11(1):37–51. doi:10.1038/nrd3591.
- Jenssen H, Hamill P, Hancock RE. Peptide antimicrobial agents. Clin Microbiol Rev. 2006;19(3):491–511. doi:10.1128/cmr.00056-05.
- Telhig S, Ben Said L, Zirah S, Fliss I, Rebuffat S. Bacteriocins to thwart bacterial resistance in gram negative bacteria. Front Microbiol. 2020;11:(2807). doi:10.3389/fmicb.2020.586433.
- Sassone-Corsi M, Nuccio SP, Liu H, Hernandez D, Vu CT, Takahashi AA, Edwards RA, Raffatellu M. Microcins mediate competition among enterobacteriaceae in the inflamed gut. Nature. 2016;540(7632):280–283. doi:10.1038/nature20557.
- Gargiullo L, Del Chierico F, D’Argenio P, Putignani L. Gut microbiota modulation for multidrug-resistant organism decolonization: present and future perspectives. Front Microbiol. 2019;10:1704. doi:10.3389/fmicb.2019.01704.
- Palmer JD, Mortzfeld BM, Piattelli E, Silby MW, McCormick BA, Microcin BV. H47: a class IIb microcin with potent activity against multidrug resistant enterobacteriaceae. ACS Infect Dis. 2020;6(4):672–679. doi:10.1021/acsinfecdis.9b00302.
- Palmer JD, Piattelli E, McCormick BA, Silby MW, Brigham CJ, Bucci V. Engineered probiotic for the inhibition of salmonella via tetrathionate-induced production of microcin H47. ACS Infect Dis. 2018;4(1):39–45. doi:10.1021/acsinfecdis.7b00114.
- Vassiliadis G, Destoumieux-Garzón D, Lombard C, Rebuffat S, Peduzzi J. Isolation and characterization of two members of the siderophore-microcin family, microcins M and H47. Antimicrob Agents Chemother. 2010;54(1):288–297. doi:10.1128/aac.00744-09.
- Azpiroz MF, Laviña M. Involvement of enterobactin synthesis pathway in production of microcin H47. Antimicrob Agents Chemother. 2004;48(4):1235–1241. doi:10.1128/aac.48.4.1235-1241.2004.
- Laviña M, Gaggero C, Microcin MF. H47, a chromosome-encoded microcin antibiotic of Escherichia coli. J Bacteriol. 1990;172(11):6585–6588. doi:10.1128/jb.172.11.6585-6588.1990.
- Orellana C, Lagos R. The activity of microcin E492 from Klebsiella pneumoniae is regulated by a microcin antagonist. FEMS Microbiol Lett. 1996;136(3):297–303. doi:10.1111/j.1574-6968.1996.tb08064.x.
- Poey ME, Azpiroz MF, Laviña M. Comparative analysis of chromosome-encoded microcins. Antimicrob Agents Chemother. 2006;50(4):1411–1418. doi:10.1128/aac.50.4.1411-1418.2006.
- Hoerr V, Duggan GE, Zbytnuik L, Poon KK, Große C, Neugebauer U, Methling K, Löffler B, Vogel HJ. Characterization and prediction of the mechanism of action of antibiotics through NMR metabolomics. BMC Microbiol. 2016;16:82. doi:10.1186/s12866-016-0696-5.
- Baquero F, Lanza VF, Baquero M-R, Del Campo R, Bravo-Vázquez DA. Microcins in enterobacteriaceae: peptide antimicrobials in the eco-active intestinal chemosphere. Front Microbiol. 2019;10:(2261). doi:10.3389/fmicb.2019.02261.
- Pradhan S, Weiss AA, Barbour AG. Probiotic properties of Escherichia coli Nissle in human intestinal organoids. mBio. 2020;11(4):e01470–20. doi:10.1128/mBio.01470-20.
- Sonnenborn U, Schulze J. The non-pathogenic Escherichia coli strain Nissle 1917 – features of a versatile probiotic. Microb Ecol Health Dis. 2009;21(3–4):122–158. doi:10.3109/08910600903444267.
- Crook N, Ferreiro A, Gasparrini AJ, Pesesky MW, Gibson MK, Wang B, Sun X, Condiotte Z, Dobrowolski S, Peterson D, et al. Adaptive strategies of the candidate probiotic E. coli Nissle in the mammalian gut. Cell Host Microbe. 2019;25(4):499–512.e8. doi:10.1016/j.chom.2019.02.005.
- Charbonneau MR, Isabella VM, Li N, Kurtz CB. Developing a new class of engineered live bacterial therapeutics to treat human diseases. Nat Commun. 2020;11(1):1738. doi:10.1038/s41467-020-15508-1.
- Isabella VM, Ha BN, Castillo MJ, Lubkowicz DJ, Rowe SE, Millet YA, Anderson CL, Li N, Fisher AB, West KA, et al. Development of a synthetic live bacterial therapeutic for the human metabolic disease phenylketonuria. Nat Biotechnol. 2018;36(9):857–864. doi:10.1038/nbt.4222.
- Leventhal DS, Sokolovska A, Li N, Plescia C, Kolodziej SA, Gallant CW, Christmas R, Gao J-R, James MJ, Abin-Fuentes A, et al. Immunotherapy with engineered bacteria by targeting the STING pathway for anti-tumor immunity. Nat Commun. 2020;11(1):2739. doi:10.1038/s41467-020-16602-0.
- Forkus B, Ritter S, Vlysidis M, Geldart K, Kaznessis YN. Antimicrobial probiotics reduce Salmonella enterica in Turkey gastrointestinal tracts. Sci Rep. 2017;7(1):40695. doi:10.1038/srep40695.
- Kan A, Gelfat I, Emani S, Praveschotinunt P, Joshi NS. Plasmid vectors for in vivo selection-free use with the probiotic E. coli Nissle 1917. ACS Synth Biol. 2021;10(1):94–106. doi:10.1021/acssynbio.0c00466.
- Davis JH, Rubin AJ, Sauer RT. Design, construction and characterization of a set of insulated bacterial promoters. Nucleic Acids Res. 2010;39(3):1131–1141. doi:10.1093/nar/gkq810.
- Young TM, Bray AS, Nagpal RK, Caudell DL, Yadav H, Zafar MA, Monack D. Animal model to study Klebsiella pneumoniae gastrointestinal colonization and host-to-host transmission. Infect Immun. 2020;88(11):e00071–20. doi:10.1128/IAI.00071-20.
- Buffie CG, Bucci V, Stein RR, McKenney PT, Ling L, Gobourne A, No D, Liu H, Kinnebrew M, Viale A, et al. Precision microbiome reconstitution restores bile acid mediated resistance to Clostridium difficile. Nature. 2015;517(7533):205–208. doi:10.1038/nature13828.
- Caballero S, Kim S, Carter RA, Leiner IM, Sušac B, Miller L, Kim GJ, Ling L, Pamer EG. Cooperating commensals restore colonization resistance to vancomycin-resistant enterococcus faecium. Cell Host Microbe. 2017;21(5):592–602.e4. doi:10.1016/j.chom.2017.04.002.
- Adolfsen KJ, Callihan I, Monahan CE, Greisen P Jr., Spoonamore J, Momin M, Fitch LE, Castillo MJ, Weng L, Renaud L, et al. Improvement of a synthetic live bacterial therapeutic for phenylketonuria with biosensor-enabled enzyme engineering. Nat Commun. 2021;12(1):6215. doi:10.1038/s41467-021-26524-0.
- Starr CG, Wimley WC. Antimicrobial peptides are degraded by the cytosolic proteases of human erythrocytes. Biochim Biophys Acta Biomembr. 2017;1859(12):2319–2326. doi:10.1016/j.bbamem.2017.09.008.
- Maroni A, Moutaharrik S, Zema L, Gazzaniga A. Enteric coatings for colonic drug delivery: state of the art. Expert Opin Drug Deliv. 2017;14(9):1027–1029. doi:10.1080/17425247.2017.1360864.
- Ozdemir T, Fedorec AJH, Danino T, Barnes CP. Synthetic Biology and engineered live biotherapeutics: toward increasing system complexity. Cell Syst. 2018;7(1):5–16. doi:10.1016/j.cels.2018.06.008.
- Petros AJ, O’Connell M, Roberts C, Wade P, van Saene HK. Systemic antibiotics fail to clear multidrug-resistant Klebsiella from a pediatric ICU. Chest. 2001;119(3):862–866. doi:10.1378/chest.119.3.862.
- Willing BP, Russell SL, Finlay BB. Shifting the balance: antibiotic effects on host–microbiota mutualism. Nat Rev Microbiol. 2011;9(4):233–243. doi:10.1038/nrmicro2536.
- Feehan A, Bacterial G-DJ. Gut microbiome-modifying therapies to defend against multidrug resistant organisms. Microorganisms. 2020;8:(2). doi:10.3390/microorganisms8020166.
- Dsouza M, Menon R, Crossette E, Bhattarai SK, Schneider J, Kim YG, Reddy S, Caballero S, Felix C, Cornacchione L, et al. Colonization of the live biotherapeutic product VE303 and modulation of the microbiota and metabolites in healthy volunteers. Cell Host Microbe. 2022;30(4):583–98.e8. doi:10.1016/j.chom.2022.03.016.
- Raffatellu M. Learning from bacterial competition in the host to develop antimicrobials. Nat Med. 2018;24(8):1097–1103. doi:10.1038/s41591-018-0145-0.
- Praveschotinunt P, Duraj-Thatte AM, Gelfat I, Bahl F, Chou DB, Joshi NS, Engineered E. coli Nissle 1917 for the delivery of matrix-tethered therapeutic domains to the gut. Nat Commun. 2019;10(1):5580. doi:10.1038/s41467-019-13336-6.
- Gibson DG, Young L, Chuang R-Y, Venter JC, Hutchison CA, Smith HO. Enzymatic assembly of DNA molecules up to several hundred kilobases. Nat Methods. 2009;6(5):343–345. doi:10.1038/nmeth.1318.
- Wycuff DR, Matthews KS. Generation of an AraC-araBAD promoter-regulated T7 expression system. Anal Biochem. 2000;277(1):67–73. doi:10.1006/abio.1999.4385.
- Gaggero C, Moreno F, Laviña M. Genetic analysis of microcin H47 antibiotic system. J Bacteriol. 1993;175(17):5420–5427. doi:10.1128/jb.175.17.5420-5427.1993.
- Datsenko KA, Wanner BL. One-step inactivation of chromosomal genes in Escherichia coli K-12 using PCR products. Proc National Acad Sci. 2000;97(12):6640–6645. doi:10.1073/pnas.120163297.
- Lee C, Kim J, Shin SG, Hwang S. Absolute and relative QPCR quantification of plasmid copy number in Escherichia coli. J Biotechnol. 2006;123(3):273–280. doi:10.1016/j.jbiotec.2005.11.014.
- Kuznetsova A, Brockhoff PB, Christensen RHB. lmerTest package: tests in linear mixed effects models. J Stat Softw. 2017;82(13):1–26. doi:10.18637/jss.v082.i13.
- Callahan BJ, McMurdie PJ, Rosen MJ, Han AW, Johnson AJA, Holmes SP. DADA2: high-resolution sample inference from Illumina amplicon data. Nat Methods. 2016;13(7):581–583. doi:10.1038/nmeth.3869.
- Wipperman MF, Bhattarai SK, Vorkas CK, Maringati VS, Taur Y, Mathurin L, McAulay K, Vilbrun SC, Francois D, Bean J, et al. Gastrointestinal microbiota composition predicts peripheral inflammatory state during treatment of human tuberculosis. Nat Commun. 2021;12(1):1141. doi:10.1038/s41467-021-21475-y.
- Sullivan MJ, Petty NK, Beatson SA. Easyfig: a genome comparison visualizer. Bioinformatics. 2011;27(7):1009–1010. doi:10.1093/bioinformatics/btr039.