ABSTRACT
Quorum Sensing (QS) is a form of cell-to-cell communication that enables bacteria to modify behavior according to their population density. While QS has been proposed as a potential intervention against pathogen infection, QS-mediated communication within the mammalian digestive tract remains understudied. Using an LC-MS/MS approach, we discovered that Citrobacter rodentium, a natural murine pathogen used to model human infection by pathogenic Escherichia coli, utilizes the CroIR system to produce three QS-molecules. We then profiled their accumulation both in vitro and across different gastrointestinal sites over the course of infection. Importantly, we found that in the absence of QS capabilities the virulence of C. rodentium is enhanced. This highlights the role of QS as an effective mechanism to regulate virulence according to the pathogen’s spatio-temporal context to optimize colonization and transmission success. These results also demonstrate that inhibiting QS may not always be an effective strategy for the control of virulence.
Introduction
Bacteria are often found as members of complex heterogeneous communities. To survive in diverse and competitive environments, such as within the gastrointestinal tract, microbes need to sense and adapt to a variety of environmental barriers such as host physiology, nutrient availability, osmotic stress, and the presence of other bacteria.Citation1–3 The use of chemical signals to perform cell-to-cell communication, a process known as quorum sensing (QS), enables bacteria to sense and respond to population density through the production of small diffusible molecules known as autoinducers (AIs).Citation4–6 This process allows bacteria to coordinate behaviors at the population level which enables them to regulate the expression of metabolically expensive activities such as bioluminescence,Citation7,Citation8 expression of virulence genesCitation9,Citation10 and biofilm formation.Citation11–13 This way, QS facilitates dynamic changes of the bacterial transcriptome according to the surrounding environment and promotes rapid adaptation to different environmental niches to increase population fitness.
Many different mechanisms for QS have evolved among both bacterial pathogens and commensals; however, the most common class of AIs in Gram-negative bacteria belongs to the family of N-acyl-homoserine lactones (AHLs). AHLs share a common homoserine-lactone ring, but differ in their structure either by the length of their acyl-chain or by the presence or absence of a substitution (such as a hydroxy- or an oxo- group) at the position C3.Citation14,Citation15 These characteristics can significantly affect stability, signaling dynamics, and specificity to their cognate receptors, explaining why they are generally regarded as intra-species communication signals.Citation16 AHL-mediated QS systems are often composed of a LuxI-family AHL synthase, and a partner LuxR-family AHL response regulator. When a LuxR-type receptor comes in contact with its cognate AHL, they often form LuxR-AHL complexes. This interaction stabilizes the protein complex and facilitates its binding to specific DNA sequences known as “lux boxes,” where LuxR-type receptors can act as either transcriptional activators or repressors.Citation15 QS signaling networks can thereby translate information about the AI levels present in the environment into changes in bacterial gene expression.
Although QS has been described in a wealth of environments, QS-mediated communication within the mammalian digestive tract remains largely understudied. This is surprising given that QS is predicted to play a major role in environmental niches where complex communities of microorganisms interact. Several factors, including low accumulation of AIs and high susceptibility to degradation, make studying cell-to-cell communication in the gut environment exceptionally challenging.Citation17 Nevertheless, research focusing on QS within the gastrointestinal tract is rapidly becoming an area of great interest, where QS has already been shown to play an important role within the host context regulating processes such as maintenance of microbiota eubiosis,Citation18 immune regulation,Citation19,Citation20 or impeding pathogenicity of harmful bacteria.Citation21 A better understanding of chemical communication within the gastrointestinal tract has great potential in developing innovative therapeutic interventions to selectively manipulate microbial behaviors and promote human health.
To better understand AHL-mediated QS signaling by gastrointestinal pathogens, we investigated its importance during natural infection by Citrobacter rodentium, a murine pathogen used to model human infections by enteropathogenic and enterohemorrhagic Escherichia coli (EPEC and EHEC).Citation22,Citation23 During infection, C. rodentium, EPEC, and EHEC induce the formation of attaching and effacing (A/E) lesions, which are characterized by intimate bacterial attachment to the host epithelia, formation of actin rich-pedestals, and the destruction of the brush border microvilli.Citation24,Citation25 This process is mediated by the type III secretion system (T3SS), a molecular syringe-like apparatus encoded by five gene operons within the Locus of Enterocyte Effacement (LEE1–5).Citation26–28 A/E pathogens utilize the T3SS to directly inject effector proteins into the host cell to subvert various host processes. While the virulence machinery of C. rodentium has been extensively studied, little is known about the role that its LuxIR homolog system (CroIR) plays during host infection or the specific signaling dynamics that AHLs have in coordinating pathogen behavior.
In this study, we developed an LC-MS/MS method to characterize the production of AHLs by C. rodentium both in vitro and across different gastrointestinal sites over the course of infection. Our results revealed that disrupting the natural ability of C. rodentium to respond to its population density led to a significant increase in pathogenicity both in vitro and in vivo. We propose that the spatio-temporal composition of AHLs in the environment enables C. rodentium to optimize the timing for epithelial attachment in a T3SS-dependent manner, thereby enhancing pathogen fitness during infection and transmission. These findings also demonstrate that the interruption of QS signaling as a therapeutic strategy may not always be effective and should not be considered a generalized strategy for the control of virulence.
Results
C. rodentium synthesizes multiple AHLs when grown in vitro
To determine the role that AHLs have in the regulation of C. rodentium behavior, we first sought to profile the pathogen’s AHL production profile across time. To detect and quantify autoinducers present in complex mixtures, an LC-MS/MS method was developed. In brief, cell-free supernatants were obtained by growing C. rodentium in various growth media (YCFAG, DMEM, DMEM+++, and LB) across various timepoints (6-, 8-, 12-, and 24-h post-inoculation). AHLs were partially purified using ethyl-acetate, and the extracts were analyzed via LC-MS/MS. Our results showed that C. rodentium primarily synthesizes C4-HSL as the dominant AHL signal with a peak average concentration of 7,973.4 nM observed at 12 h post-inoculation when grown in YCFAG. Additionally, C6-HSL and 3-hydroxy-C6-HSL were also detected at lower concentrations, with peak average levels of 21.8 nM and 0.3 nM at 12-h post-inoculation, respectively ( and Figure S1B). Contrary to previous suggestions hinting that C. rodentium also synthesizes 3-oxo-C6-HSL,Citation29 there was no detection of 3-oxo-C6-HSL in any of the conditions tested. Our results also showed, as with many other QS systems, that the peak concentration for all detected AHLs was attained near the stationary phase (12 h post-inoculation; Figure S1). However, a sharp reduction in all detected AHLs was observed at the stationary phase (24 h post-inoculation), suggesting the presence of an uncharacterized regulatory mechanism in C. rodentium that might control the production or degradation of AHLs at high bacterial cell densities.Citation30
Figure 1. AHL production during Citrobacter rodentium growth. (a) AHL production profile of WT C. rodentium grown over time in YCFAG (yeast extract, casitone and fatty acid glucose media) as analyzed via LC-MS/MS. The black line represents the mean values across time; shaded areas represent the standard deviation. (b) Comparison of AHL production by WT C. rodentium, the ∆croI mutant, and the complemented strain analyzed at 12 hours post-inoculation in YCFAG media. The values reported for the WT strain are the same as those reported in panel (a) at 12 hours post-inoculation. Data are represented as the mean and standard deviation of three independent replicates. Significance was determined by a one-way ANOVA with Tukey’s for multiple comparisons test. (c) Chemical structures of the different N-acyl-homoserine lactones (AHLs) tested: N-butyryl-L-homoserine lactone (C4-HSL), N-hexanoyl-L-homoserine lactone (C6-HSL), N-(β-ketocaproyl)-L-homoserine lactone (3-oxo-C6-HSL), 3-hydroxy-hexanoyl-L-homoserine lactone (3-hydroxy-C6-HSL).
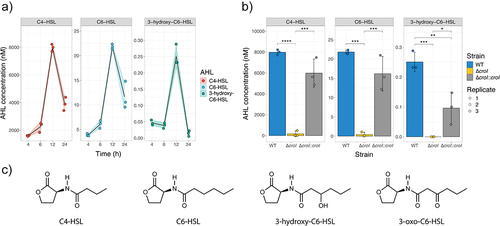
To test whether CroI, the only predicted AHL synthase encoded in C. rodentium, is responsible for synthesizing the multiple AHLs detected, an AHL-synthase deletion mutant (∆croI) and its complemented strain were created. Deletion of croI resulted in a significant reduction of all detected AHLs, whereas chromosomal complementation of the AHL synthase (∆croI:croI) resulted in the restoration of AHL synthesis to levels resembling those by the WT strain (). These data demonstrate that C. rodentium utilizes the AHL-synthase CroI to synthesize C4-HSL, C6-HSL, and 3-hydroxy-C6-HSL (chemical structures in ).
RNA-seq revealed that AHL-mediated QS regulates virulence and metabolism pathways in C. rodentium
To gain insight into which genes are regulated by the AHL-mediated QS system of C. rodentium, we performed RNA-seq comparing the gene expression profile between the wild-type (WT) and ∆croI strains. We identified that in the absence of AHL production, there were 92 transcripts differentially upregulated and 125 transcripts differentially downregulated (; differential expression data are available in Supplementary Data 3). Gene set enrichment analysis (GSEA) identified an enrichment in pathways related to bacterial secretion systems and to flagellar assembly, as well as a downregulation of genes associated with starch and sucrose metabolism (, data available in Supplementary Data 5). KEGG module over-representation analysis further revealed that differentially upregulated genes in the ∆croI strain were strongly associated specifically with the type III secretion (T3S) system and associated effectors (, Supplementary Data 6). Indeed, we found that 35 out of the 41 LEE-encoded genes were significantly upregulated in the ∆croI strain, including several genes encoding transcriptional regulators and key structural, chaperone, translocator, and effector components of the T3SS (), as well as other non-LEE encoded T3S-related effector genes (espT, espN2–2, espJ, espS, and espN1). In contrast, some of the most significantly downregulated genes included genes associated with stress response (rpoS, osmY, elaB, and katE), and with starch and sucrose metabolism (bcsA, treZ, treY, treF, otsA, amyA, glgX, and glgC). These results are significant given the established importance that the interplay between stress-response, metabolism, and virulence has in the successful colonization of a host by a pathogenCitation32,Citation33. Together, these results indicate that C. rodentium is capable of detecting changes in AHL levels in the environment and using them as regulatory signals to initiate targeted changes in gene expression involved in virulence, stress, and metabolism.
Figure 2. QS regulates expression of the type III secretion system in Citrobacter rodentium. (a) Volcano plot showing differentially expressed genes (DEGs) from RNA-seq data comparing the ∆croI strain to WT C. rodentium. Genes shown in red are significantly upregulated with a fold change greater than 1.25 and p-value < 0.05, genes in blue are significantly downregulated with a fold change lower than 1.25 and p-value < 0.05. The top 20 significantly differentially expressed genes ordered by ascending p-value are labelled. Differential expression data are available in Supplementary Data 3. (b) Pathway enrichment analyses were calculated by using gene set enrichment analysis (GSEA; shown in blue); and overrepresented KEGG modules (shown in orange) were calculated by using ClusterProfiler. Only pathways that passed an FDR < 0.05 were considered significantly enriched. Supplementary enrichment data are available in Supplementary Data 5–6. (c) Heatmap showing Z-score expression of genes associated with the type III secretion system. Each operon within the locus of enterocyte effacement (LEE1–5) is displayed using arrowheads to indicate gene orientationsCitation31. Significantly differentially regulated genes when comparing ∆croI and WT are shown as bolded text with an asterisk at the end of the gene name. ORA, over-representation analysis.
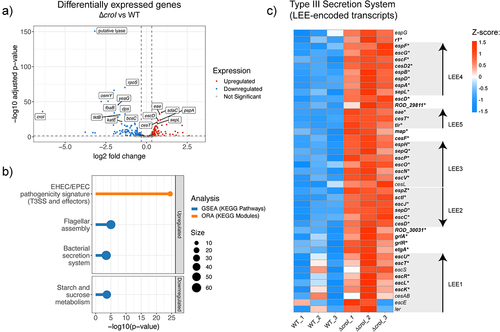
QS regulates T3SS activity and epithelial cell attachment of C. rodentium
To confirm whether the observed increase in T3S-associated transcript levels corresponds to an increase in translation and secretion of type III-associated proteins, a T3S assay was performed. The ΔcroI strain displayed a 49% average increase in the abundance of secreted proteins as compared to WT C. rodentium (), while chromosomal complementation of the AHL synthase (∆croI:croI) restored the protein secretion profile back to WT levels. To investigate whether this phenotype is mediated by CroR, we tested if the deletion of the AHL-receptor CroR would also induce an increase in secretion of type III-associated proteins. Interestingly, both ∆croR and its complemented strain (∆croR:croR) showed no changes in their type III secretion profile when compared to the WT strain. This observation was consistent with a previous study by Colthurst et al.,Citation29 where no phenotypical changes were reported between WT and a ∆croR strain. To further disentangle the AHL-mediated CroI/CroR signaling system, we next engineered a ∆croI∆croR double deletion mutant by deleting croR in the ∆croI mutant background. Our results revealed that the secretion profile of the ∆croI∆croR strain reverted to levels similar to those of the WT. These results suggest that CroR acts as a transcriptional modulator of T3SS, enhancing its expression either directly or indirectly to facilitate bacterial attachment and colonization when bacterial densities are low and AHL levels are minimal. However, as bacterial densities and AHL levels increase, the binding of the cognate AHL to CroR relieves this CroR-mediated enhancement and leads to a decrease in expression of the metabolically expensive T3SS. Collectively, these findings demonstrate that C. rodentium utilizes the CroIR QS system to modulate T3SS gene expression, a critical virulence factor during infection, according to the pathogen’s spatio-temporal context.
Figure 3. CroI plays a role in regulation of type III secretion (T3S) and bacterial attachment to host epithelial cell surfaces. (a) Profile of T3SS secreted proteins derived from the supernatants of wild-type (WT) C. rodentum, ∆croI, ∆croR, ∆croI∆croR, and complemented strains as shown on an SDS-16% PAGE gel stained with Coomassie Blue G250. Data were also displayed as the fold change difference of the type-III secreted proteins measured across at least three independent experiments. Bar heights and error bars represent mean ± standard deviation. Significance was determined by a one-way ANOVA with Dunnett’s for multiple comparisons. (b) Bacterial attachment of WT C. rodentium, ∆croI, ∆croR, and complemented strains to CMT-93 murine epithelial cells 4 hours post-infection. (c) Attachment of WT and ∆croI strains to CMT-93 cells in response to supplementation of culture media with various AHLs (10 µM) or DMSO vehicle control. Data are represented as the mean and SD of at least four independent replicates (N = 4–6). Significance was determined using pairwise t-tests with Bonferroni corrected p-values for multiple comparisons. (d) RT-qPCR gene expression analysis of selected differentially expressed genes identified through RNA sequencing. dnaQ and recA were used as endogenous controls. All primers had an efficiency between 98–103%. Results are the average of three technical replicates prepared from strains grown in DMEM in biological triplicate cultures. Bar heights and error bars represent geometric means ± standard deviation. Statistics were calculated by using multiple t-tests with Bonferroni correction for multiple comparisons. T3SS, type III secretion system; QS, quorum sensing; 3OH-C6-HSL, 3-hydroxy-C6-HSL.
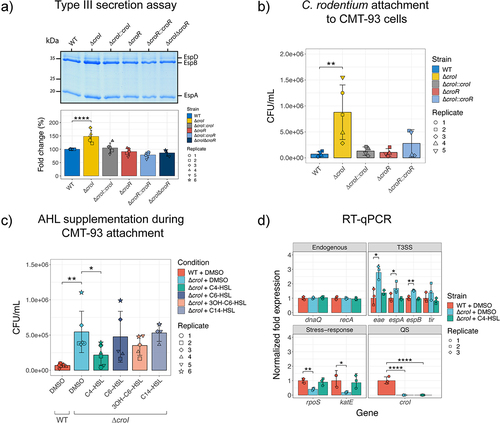
Given the observed upregulation in T3SS activity, we examined whether disruption of CroI could lead to additional functional enhancements in C. rodentium virulence responses during infection. One of the hallmark characteristics of A/E infection is the intimate attachment to the host epithelium, and so in vitro assays were designed to assess bacterial adherence to intestinal epithelial cells, with the aim of modeling conditions within the murine gut. To achieve this, a mouse rectal carcinoma cell line (CMT-93 cells) was utilized. Indeed, the efficiency of C. rodentium attachment to CMT-93 cells was significantly increased in the ∆croI mutant (). Consistent with our previous results, we did not identify any differences in epithelial cell attachment for the ∆croR and ∆croR::croR strains when compared to WT. If the absence of AHLs in the environment enhances epithelial adherence, we hypothesized that exogenous supplementation of AHLs to the non-secreting ∆croI strain would chemically complement the CMT-93 attachment phenotype. In support of this hypothesis, we found that indeed C4-HSL was capable of consistently reverting this phenotype by restoring ∆croI epithelial attachment to levels similar to WT, while no significant differences were observed in any other of the tested AHLs (). This phenotype was not due to any changes in C. rodentium growth dynamics caused by the supplementation of AHLs into the culture media (Figure S2). Collectively, these findings show that in the absence of AHLs, C. rodentium can increase bacterial attachment to host epithelial cells; additionally, they demonstrate that C4-HSL plays a crucial role as a signaling molecule directly influencing the virulence response of C. rodentium.
To verify the modulatory effects of C4-HSL, we measured changes in the expression of key genes identified from our RNA-seq screen using RT-qPCR. As expected, we confirmed that several T3SS-related genes were significantly upregulated when AHL synthesis was disrupted; in contrast, stress-related genes were shown to be significantly downregulated in the ∆croI strain when compared to WT (). Furthermore, we confirmed that the exogenous addition of C4-HSL to the culture media chemically complemented ∆croI by reverting transcript expression to WT levels. Together with our functional analysis, these data suggest that the production and detection of C4-HSL plays a critical role in the regulation of C. rodentium virulence and bacterial stress responses and may therefore have an essential role in pathogen adaptation during host colonization.
AHLs can be detected across the gastrointestinal tract during a time course of infection
Having characterized the link between the AHL-mediated QS system of C. rodentium and pathogen behavior in vitro, we next focused on investigating the role of QS during host infection. Studying C. rodentium offers the rare opportunity to directly profile the concentration of target AHLs present within the murine gut both in naïve mice and over the time course of infection. Adult C57BL/6J mice were gavaged with either WT C. rodentium, ∆croI, or with PBS as a control. AHLs were then partially purified from the regional gut contents and extracts were analyzed via LC-MS/MS. To capture both early- and peak-infection stages, intestinal contents were collected at 4- and 7-days post-infection (p.i.), focusing specifically on the cecum and colon, which are important sites of initial C. rodentium colonization . We detected and quantified C4-HSL in WT colonized mice at both day 4 and 7 p.i. and in both the cecum and colon (; with an average of 0.10 and 12.80 ng/g in the cecum, and 3.71 and 21.50 ng/g in the colon at day 4 and 7 p.i., respectively), confirming C4-HSL as the dominant AHL produced by C. rodentium during infection. Low concentrations of C6-HSL were also detected, primarily within the colon during peak infection, with averages of 0.06 and 0.72 ng/g at day 4 and 7 p.i., respectively. Despite demonstrating the capacity of C. rodentium to synthesize 3-hydroxy-C6-HSL, it could not be detected in any of the tested samples. Since detected 3-hydroxy-C6-HSL concentrations remained low even within saturated culture conditions (), it is likely that the accumulated levels of 3-hydroxy-C6-HSL within the gut contents are lower than our limit of detection. No 3-oxo-C6-HSL was detected in any of the samples tested. Both C4-HSL and C6-HSL were only detected in samples where C. rodentium reached a bacterial burden greater than ~107 CFUs, suggesting that a minimum threshold of pathogen density is required by our methodology to effectively detect AHLs (Figure S3A and Figure S4C).
Figure 4. Multiple AHLs can be detected in vivo over the course of C. rodentium infection. (a) Graphical representation of the murine infection set up. In brief, C57BL/6J mice were gavaged with either WT C. rodentium, ∆croI, or PBS (uninfected control group). At experimental endpoint large intestinal (cecum and colon) and systemic (spleen) organs were collected for CFU enumeration. (b) Cecum and colon contents were also collected on days 4 and 7 post-infection (p.i.) for partial purification and detection of N-acyl-homoserine lactones (AHLs) via LC-MS/MS. (c,d) Changes in concentration of C4-HSL and C6-HSL detected in cecum and colon contents during (c) WT and (d) ∆croI infection. 3-hydroxy-C6-HSL and 3-oxo-C6-HSL were not detected. As a reference, the values detected for the naïve mice were plotted on both panels (c) and (d) as day 0 post-infection. The black line represents the mean values across time, and shaded areas represent the standard deviation with an N = 6–12 mice per group. Statistics were calculated by using multiple t-tests with Bonferroni correction for multiple comparisons.
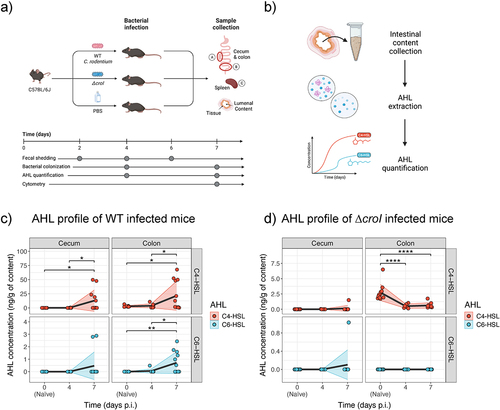
To further investigate the potential role of AHLs during infection, we next correlated the regional C. rodentium burden of individual mice to their corresponding AHL concentrations. We found that both C4-HSL and C6-HSL levels were positively associated with both lumenal (Figure S3A; C4-HSL rho = 0.92, p < 0.001; C6-HSL rho = 0.86, p < 0.001) and tissue-associated (Figure S4C; C4-HSL rho = 0.65, p = 0.026; C6-HSL rho = 0.68, p < 0.014) C. rodentium subpopulations in the colon. This association was not observed in the cecum (Figure S3A and Figure S4C); this may be due to the high degree of variability associated with this intestinal site where factors such as food consumption (further diluting AHL concentrations) or the high variation in the consistency and amount of intestinal content available between different mice made the detection of cecal AHLs particularly challenging. Remarkably, low levels of C4-HSL were also detected in colonic samples of naïve mice (shown as day 0 p.i. in ; with an average of 2.74 ng/g) despite having no detectable colonization of C. rodentium (as measured via selective plating). These findings suggest that there might be members of the resident microbiota capable of synthesizing AHLs.
We next examined how AHL concentrations change after infection with the ∆croI mutant strain. We found that detected C4-HSL levels were significantly reduced in ∆croI-infected mice when compared to the naïve samples (; average of 0.51 ng/g and 0.63 ng/g detected in the colon at day 4 and 7 p.i., respectively). Furthermore, our data revealed that AHL levels tended to decrease with increasing ∆croI burden (Figure S3B), further supporting the possibility that members of the resident microbiota can synthesize C4-HSL and that the invading non-AHL producing ∆croI strain is displacing any AHL-producing commensal strains from their natural intestinal niche, leading to a loss of microbiota-derived C4-HSL from the gut. Altogether, these data validate the production of AHLs within the gut, not only by C. rodentium during infection but possibly also by the resident microbiota. Furthermore, the temporal accumulation of C. rodentium-produced AHLs in vivo, suggests that the production and detection of AHLs is likely a key regulatory signal used by C. rodentium to coordinate infection stage-specific gene expression.
C. rodentium utilizes AHLs to regulate colonization dynamics and control host damage during infection
To further expand on the role of the AHL-mediated QS system of C. rodentium during host infection, we assessed the colonization dynamics of both the WT and ∆croI strains following inoculation of C57BL/6 mice. Enumeration of C. rodentium shed in the feces daily post-infection revealed a significant increase in bacterial shedding over the course of ∆croI infection (; Mixed-effects model with Geisser-Greenhouse correction, Time × Strain: p = 0.0037), indicating a possible role for QS in pathogen transmissibility to new hosts. On days 4 and 7 post-infection, we assessed pathogen burden across GI tissues, as well as within the spleen to represent systemic spread. We found no significant differences in pathogen burden between the ∆croI and WT strains in either lumen-associated C. rodentium subpopulations in the cecum or colon nor in tissue-associated subpopulations (Figure S4A-B). These observations could suggest that the local environment has reached its carrying capacity limit, and the C. rodentium burden has reached peak infection levels.Citation34,Citation35 When measuring the systemic burden, we observed that by day 7 p.i. the ∆croI strain had a 100% rate of systemic spread, as compared to 50% during WT infection (; Fisher’s exact test, p = 0.015), as well as an overall increase in spleen pathogen burden (). We hypothesize that this may be a consequence of the increased expression of virulence machineries, such as the T3SS, in the ∆croI strain which leads to an increase in damage to the epithelial cell barrier, and an increase in translocation of bacteria entering host circulation.Citation22,Citation36,Citation37 Analysis of the tissue-derived cytokine profile across the large intestine did not identify any major changes between WT- and ∆croI-infected tissues, although there was a trend toward decreased levels of cecal TNFα in the context of ∆croI infection (Figure S5A-B). These data indicate that pathogen transmissibility and systemic spread may be influenced by QS, possibly as an important strategy to coordinate the expression of virulence genes during in vivo infection.
Figure 5. C. rodentium ∆croI displays increased pathogenicity in C57BL/6J and C3H/HeJ mouse infection models. (a) Fecal shedding of C. rodentium throughout the infection time course. Data were analyzed using a mixed-effects model with geisser-greenhouse correction (time × strain: p = 0.0037) to account for the repeated measurements of the same mice across the time. A Wilcoxon rank test using Holm-Šidák correction for multiple comparisons was utilized to evaluate differences at each individual timepoint. (b,c) C. rodentium burden in cecal and colonic contents measured at day 4 and 7 post-infection (p.i.). (d,e) Systemic colonization found in whole spleens at day 4 and 7 post-infection. (d) Success rate of C. rodentium spleen colonization. Statistical analysis was calculated using Fisher’s exact test. (e) CFU burden found in whole spleens. (f) Survival of susceptible C3H/HeJ mice infected with WT, ∆croI, ∆croR and ∆croI∆croR strains of C. rodentium reveals an increased morbidity and mortality in mice infected with the ∆croI strain as compared to WT infection. For WT an N = 16 was used, while for each of the mutant strains tested an N = 10 mice was used instead. Statistics were calculated using a Gehan-Breslow-Wilcoxon test comparing each strain against WT C. rodentium. (a-c, e) Lines represent geometric mean ± geometric standard deviation, with an N = 6–12 mice per group. The limit of detection was displayed as a dotted line. Statistical analysis was performed by using a Mann-Whitney test.
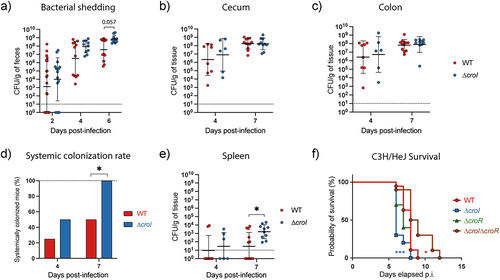
Given the observed increase in systemic spread in mice infected with the ∆croI strain, we sought to determine whether interfering with AHL synthesis altered host damage or displayed any changes to the lethality of infection. This was done by infecting C3H/HeJ mice, which are highly susceptible to C. rodentium and succumb to 100% lethality.Citation36 Indeed, mice gavaged with the ∆croI strain displayed hypervirulence, resulting in an increase in morbidity and mortality of the mice as compared to WT infection (). In contrast, this phenotype was successfully reversed upon restoration of AHL-production during infection with the ∆croI:croI complemented strain (Figure S6A). Consistent with our previous results, deletion of the AHL-receptor CroR or its complemented strain (∆croR:croR) did not result in differences in host disease outcome as compared to WT infection ( and Figure S6A-C). Infection with the ∆croI∆croR double deletion mutant also successfully rescued the hypervirulent phenotype observed in the ∆croI strain, confirming the importance of the CroIR system as a critical regulator utilized by C. rodentium to modulate its virulence response. Together, these data show that interference of the AHL-mediated QS system in C. rodentium leads to increased pathogenesis and host disease severity. Additionally, these results highlight the complex regulatory networks that pathogens can employ to dynamically change their behavior according to their surrounding environment to ultimately maximize colonization success.
Discussion
QS dynamics within the mammalian gastrointestinal tract remains poorly understood, and many questions remain regarding the composition and abundance of different AHLs across distinct GI regions, the kinetics of AHL synthesis and degradation, and the potential regulatory capacity that AHLs have as signaling molecules for both commensal and pathogenic microbes. In this study, we show that AHLs can be detected across different regions of the mammalian gastrointestinal tract both during health and disease, hinting toward their use by both pathogens and commensals during niche colonization. We also highlight the regulatory capacity that AHLs have during a natural model of infection by demonstrating how C. rodentium utilizes QS-mediated signaling to control the timing of expression of virulence genes to maximize its chances to successfully colonize its host ().
Figure 6. QS plays an important role regulating C. rodentium pathogenesis within the gut. Our proposed model illustrates how the CroIR system in C. rodentium regulates the pathogen’s virulence response and host infection dynamics. The CroIR system relies on a LuxI-type synthase, CroI, which produces multiple AHLs: C4-HSL, C6-HSL and 3-hydroxy-C6-HSL; and also depends on a LuxR-type receptor and transcriptional regulator, CroR, whose function is mediated by the binding of its cognate AHL, C4-HSL. When the cell density is low, CroR enhances the expression of several signaling cascades that directly or indirectly lead to the upregulation of virulence machineries, such as the type III secretion (T3S) system, which promotes the intimate attachment of the pathogen to the host epithelium, and enhances colonization success. As the population density increases, the binding of C4-HSL to CroR reverts the T3SS expression to basal levels and reduces the metabolic cost associated with expressing this virulence machinery. Together, this highlights the role of CroIR as an effective mechanism to regulate virulence and infection dynamics according to the pathogen’s spatio-temporal context.
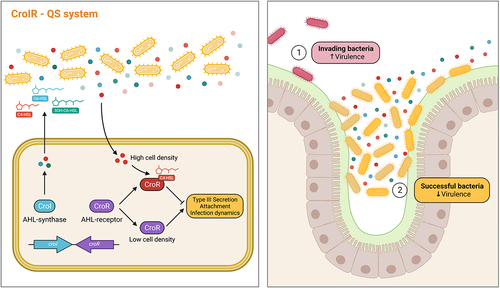
The detection of AHLs from the mammalian intestine has been notoriously elusive. Reasons for this include low accumulation of AHLs found in vivo, low sensitivity and biases associated with the use of biosensors, difficulty in detecting a wide array of molecular structures of AHLs, and possible degradation of AHLs by either the host or the microbiota.Citation17 To date, only a handful of studies have detected AHLs from in vivo samples. These observations have mostly been indirect, either by using biosensorsCitation38, or by using mass spectrometry to analyze fecal samples derived from IBD patients.Citation39 To our knowledge, only one study has successfully detected AHLs directly from biological samples by analyzing cecal contents, sera, and liver of mice.Citation40 Our study not only supports previous findings by showing that AHLs can be detected in vivo, but further demonstrates how AHL composition changes across multiple intestinal sites throughout the course of infection (). While we observed a similar abundance of C4-HSL in cecal contents of infected animals to Xue et al.,Citation40 we were also able to detect low levels of C6-HSL, a secondary AHL molecule synthesized by C. rodentium (). An interesting observation was the overall increase in AHL levels in colonic contents when compared with cecal contents in both infected and uninfected animals. These findings suggest the existence of ecological forces affecting QS dynamics within the gastrointestinal tract, such as the effects of complex topology present within intestinal colonic crypts leading to the prevention of AHL flow through,Citation41 or the effects of long-range flow from bacteria colonizing upstream in the intestine allowing for higher concentrations of AHLs to accumulate downstream.Citation42
It is rare to be able to investigate the role of a QS system during pathogen infection of its natural host, and studying C. rodentium offered us the opportunity to characterize the AHL-mediated QS regulon both in vitro and in vivo. By studying a strain lacking an AHL-synthase (∆croI), we were able to recreate conditions that mimic the environment that C. rodentium encounters as it initially enters the gastrointestinal tract (when AHL concentrations are likely minimal). Our results show that in the absence of AHLs, C. rodentium promotes the expression of the T3SS (), highlighting the role that the absence of AHLs has as a regulatory cue stimulating the pathogen to prepare for its intimate attachment to the host epithelia. This relationship is likely context-dependent, as previous studies have shown that a ∆croI mutant strain had reduced attachment to non-biotic surfaces.Citation29 Collectively, these observations demonstrate that QS regulation of pathogen behavior according to their spatio-temporal context is critical for infection success. This regulation can range from coordinating behaviors that promote colonization during an initial infection, such as enhancing virulence machinery like the T3SS, to modulating those that lead to host damage, potential immune evasion, and even transmission to a new host ( and Figure S5).
It was intriguing to note that a single synthase, CroI, could lead to the production of three distinct AHLs (). However, it is important to mention that several LuxI-family AHL synthases have been reported to enable bacteria to produce multiple AHLs.Citation43,Citation44 While the exact mechanism remains to be defined, the absence of other AHL-synthases within the C. rodentium genome suggests that CroI might be capable of interacting with multiple acylated acyl-carrier proteins (acyl-ACPs), which serve as one of the precursor molecules utilized to synthesize AHLs and which play a crucial role in determining the specific AHL structure formed.Citation45,Citation46 Typically, AHL-synthases have a reduced catalytic efficiency for non-native acyl-ACPs, potentially explaining why the concentration of C6-HSL and 3-hydroxy-C6-HSL remained significantly lower when compared to C4-HSL. The production of multiple AHLs also supports the idea of heterogeneity in AI accumulation that can happen under a natural model of infection, and highlights possible mechanisms of host-adaptation that can arise from having subpopulations of isogenic bacteria existing in different phenotypical states which consequently lead to an overall increase in bacterial fitness of the population as a whole.Citation2,Citation47 Considering this, high accumulation of C4-HSL could be considered a late-stage infection signal indicating to the attached subpopulation of C. rodentium that they were successful in reaching their colonization niche and on establishing an infection, consequently triggering a downregulation in expression of the metabolically expensive T3S machinery. Alternately, high C4-HSL concentrations could also indicate the presence of another competing pathogen or microbiota member within the C. rodentium colonization niche, indicating that colonization conditions are not optimal and the expression of the T3SS could come at a disadvantage.Citation48 It would be interesting to explore what role C6-HSL and 3-hydroxy-C6-HSL have as signaling molecules during C. rodentium infection. One hypothesis is that they allow C. rodentium to expand its QS signaling networkCitation49 allowing for a fine-tuned regulation of pathogen behavior according to the infection stage and geographic location within the gut. Alternately, they could potentially play a role as inter-species signaling molecules which could increase C. rodentium’s ability to outcompete other microbes that share its colonization nicheCitation48; or they could act as trans-kingdom signals capable of modulating host immune responses to increase C. rodentium colonization success rate.Citation19,Citation20
Interfering with the QS communication of bacteria could provide a powerful strategy to control virulence of pathogenic bacteria. Strategies such as degradation or interference of the binding of an autoinducer to its cognate receptor have been shown to lead to the repression of virulence in other pathogens, thus allowing the immune system to naturally clear the infection without the need for additional interventions such as antibiotics.Citation50,Citation51 Results presented here, as well as by others, show that in the absence of AHLs, some pathogens can display an increase in pathogenesis and host disease severity.Citation10,Citation52–54 This highlights the necessity of understanding QS regulation in the context of infection of individual pathogens, and also demonstrates that the development of future therapeutics targeting QS signaling needs to be pathogen-specific, and should not be considered a generalized strategy.
In summary, our work demonstrates that AHLs can be detected in different regions of the mammalian gastrointestinal tract. These results have the potential to be expanded to other experimental systems and aid the understanding on how AHLs fluctuate throughout the gastrointestinal tract in both health and disease. Additionally, we demonstrated the ability of the enteric pathogen C. rodentium to utilize its QS system to produce, release and respond to AHLs to regulate its virulence and maximize colonization success. Through a comprehensive analysis of AHL-mediated QS, we characterized the temporal production of AHLs by C. rodentium, as well as their effect on gene expression, virulence behavior, and host infection dynamics. Together, these findings demonstrate the important role that QS plays within the mammalian gut and further expand our understanding of QS regulation by gastrointestinal pathogens.
Materials and methods
Bacterial strains and culturing conditions
Bacterial strains and plasmids used in this study are listed in the Supplemental Tables S1-S2. Unless otherwise stated, bacterial strains were routinely grown aerobically in Lysogeny Broth (LB) at 37°C with agitation at 220 rpm. When necessary, chloramphenicol was supplemented at 30 µg/mL.
Construction of deletion and complemented strains
Chromosomal in-frame deletions of croI and croR were created via allelic exchange. The double deletion mutant, ∆croI∆croR was generated by removing croR from the ∆croI mutant background. In short, target deletion constructs were generated using primers found in Supplemental Table S3. Purified DNA fragments were ligated into the suicide plasmid pRE112 by using Gibson assembly.Citation55 Biparental mating was performed using MFDpir Citation56 as a donor strain to transfer the plasmid into C. rodentium. The loss of the pRE112 plasmid was induced via sucrose counter-selection.Citation57 The in-frame deletion was confirmed via PCR and sequencing.
Chromosomally complemented strains were generated by amplifying the gene of interest along with its native promoter using primers listed in Supplemental Table S4. The constructs were then cloned into the pUC18R6KT-mini-Tn7T plasmid system.Citation58 Conjugation to the target Citrobacter strain was achieved via triparental mating using MDFpir as the donor and pTNS2Citation58 as the helper strain. The correct chromosomal insertion was confirmed via PCR and sequencing using the primers PTn7R and PglmS-down (Supplemental Table S4). All deletion and complemented strains had similar growth rates to wild-type (WT) (Figure S1A).
Materials and reagents
N-butyryl-L-homoserine lactone (C4-HSL), N-hexanoyl-L-homoserine lactone (C6-HSL), N-(β-ketocaproyl)-L-homoserine lactone (3-oxo-C6-HSL), and N-tetradecanoyl-L-homoserine lactone (C14-HSL) were purchased from Cayman Chemical Company (Ann Arbor, MI, USA). 3-hydroxy-hexanoyl-L-homoserine lactone (3-hydroxy-C6-HSL) was obtained from Chemodex (St. Gallen, Switzerland). Analytical standards were resuspended in dimethyl sulfoxide (DMSO) and stored at −70°C until analysis. HPLC-grade acetonitrile was obtained from Fisher Chemical; HPLC-grade ethyl acetate, formic acid (FA), and DMSO were purchased from Sigma-Aldrich (St. Louis, MO, USA).
Mouse infections
Animal experiments were performed in accordance with the Canadian Council on Animal care and the University of British Columbia (UBC) Animal Care Committee guidelines (Animal Care Protocol A20–0187). Female C57BL/6J mice were purchased from Jackson Laboratory (Bar Harbor, ME) and maintained on a 12-h light–dark cycle in a specific pathogen-free facility at UBC. Upon arrival, the mice were housed in groups of 3–4 per cage, and all experiments were conducted using at least three independent cages per experimental group. All mice were allowed to acclimatize to the facility for a period of 1 week before the infection.
C. rodentium infection was performed as follows: mice were gavaged orally with 108 CFU (in 100 µL volume) of either the WT or ∆croI strain of C. rodentium from overnight cultures, as confirmed by retrospective plating. Mice were monitored daily post-gavage for weight loss and clinical symptoms before euthanasia at the experimental endpoint on day 4 or day 7 post-infection by isoflurane anesthesia followed by carbon dioxide inhalation. Mice were 7 weeks old at the time of infection. Mice that remained uninfected on the day of the experimental endpoint were excluded from all analyses.
To assess morbidity and mortality, female C3H/HeJ mice were purchased from Jackson Laboratory. At 7-weeks-old mice were gavaged orally with 108 CFU of either wild-type, mutant, or complemented strains of C. rodentium (100 µL volume) from overnight cultures. Mice were monitored twice daily for weight loss and clinical symptoms and were euthanized upon reaching the humane endpoint.
In vivo sample collection
Fecal samples were collected every 2 days post-infection to enumerate C. rodentium burden. At the experimental endpoint, large intestinal (cecum and colon) and systemic (spleen) organs were collected. Cecum and colon samples were separated into lumenal and tissue-associated subpopulations by opening the tissues longitudinally to gently collect lumenal content, before washing the remaining tissue twice in phosphate buffered saline (PBS) for collection of mucosal associated-bacteria. Spleens were collected whole.
CFU enumeration of bacterial burden was performed by collecting samples in 1 mL PBS followed by homogenization in a FastPrep-24 (MP Biomedicals) at 5.5 m/s for 2 min. Sample homogenate was then diluted for plating on MacConkey agar (Difco). Bacterial plates were incubated at 37°C for 18–20 h before counting.
Intestinal samples for AHL quantification were collected from cecal and colonic contents as described above. Samples were weighed for normalization and stored at −70°C until further processing during AHL extraction (see ‘AHL extraction and sample preparation’).
Supernatants for in vitro detection of AHLs
Overnight cultures of C. rodentium were inoculated (1:40) in 5 mL of the different media of interest: LB broth and YCFAG (Yeast extract, Casitone, and Fatty Acid Glucose)Citation59 were incubated with agitation at 37°C; DMEM (Dulbecco’s Modified Eagle’s Medium; Hyclone) and DMEM+++ (DMEM supplemented with 10% heat-inactivated fetal bovine serum, 1% Non-Essential Amino Acids, and 1% L-glutamine) were incubated statically at 37°C in 5% CO2. Cell-free bacterial-derived supernatants were obtained at 6-, 8-, 12-, and 24-h post-inoculation by centrifuging and filter sterilizing extracts through a 0.22 µm filter. Extracts were stored at −70°C until processing.
AHL extraction and sample preparation
The AHLs were extracted by utilizing a method based on ShawCitation60 and ZhuCitation61 with modifications. In short, for in vitro experiments, cell-free derived supernatants were extracted three times using three volumes of ethyl acetate. The organic fraction was collected and evaporated until dry using a vacuum centrifuge (Vacufuge, Eppendorf). Samples were resuspended in 200 µL of 70% acetonitrile acidified with 0.1% formic acid (FA), filtered through a 0.22 µm porosity filter, and stored at −70°C until analysis. For murine intestine-derived samples, extractions were performed three times by first homogenizing the cecal and colonic contents in 50% ethyl acetate using a Mixer Mill MM 400 (Retsch), followed by constant agitation for at least 10 min at 4°C using a rocker. Mixtures were centrifuged at 8000 ×g for 10 min, and the supernatants were collected into a separate tube. Samples were re-extracted three times in 100% ethyl acetate, collecting only the organic fraction after centrifugation. Extracts were completely dried using a vacuum centrifuge, reconstituted in 50 µL of 70% acetonitrile (0.1% FA), filtered through a 0.22 µm porosity filter, and stored at −70°C until analysis.
LC-MS/MS analysis
Extracted samples were injected into an Agilent 6460 Triple Quadrupole (QQQ) Mass Spectrometer (Agilent) equipped with an electrospray ionization source operated in the positive ion mode. A reversed-phase Nucleosil C18 5 µm, 250 × 4.6 mm column was used for the chromatographic separation of AHLs. The MS was conducted with an electrospray ionization voltage of 3500 V, while using 50 psi nebulizer gas at a temperature of 350°C. The sample injection volume was 5 µL. LC separation was performed while using mobile phase A (0.1% FA in 3% acetonitrile 97% water) and mobile phase B (0.1% FA in 90% acetonitrile 10% water), at a flow rate of 450 µL/min. The separation gradient was as follows: 40% B for 2 min, 40% to 60% B in 4 min, 60% to 70% B in 2 min, 70% to 80% in 3 min, 80% for 1 min, 80% to 40% in 1 min, and 40% for 2 min. For the identification and quantification of analytes, multiple-reaction-monitoring (MRM) was utilized (Supplemental Table S6). Data were analyzed using MassHunter Qualitative Analysis B.06.00 software (Agilent Technologies). Calibration curves were constructed with a minimum of 6-points using analytical standards of C4-HSL, C6-HSL, 3-hydroxy-C6-HSL, and 3-oxo-C6-HSL with concentrations ranging from 0.1 to 250 ppb for murine intestine-derived samples, and from 10 to 5000 ppb for in vitro experiments. Regression coefficients (R2) of all AHL-calibration curves were greater than 0.99.
RNA isolation and sequencing
Wild-type and ∆croI strains were subcultured in 6-well plates with 3 mL of DMEM and grown statically for 6 h at 37°C in 5% CO2. Bacterial cells were collected and treated with RNAprotect Bacteria Reagent (Qiagen) and stored at −70°C until extraction. RNA was isolated using the Qiagen RNeasy Mini Kit, and genomic DNA was digested on column by using the RNase-Free DNase Set (Qiagen). RNA quality was assessed using a 2100 Bioanalyzer (Agilent Technologies, USA); all samples had RNA Integrity scores higher than 6 which were considered as high quality for C. rodentium Citation62. Library preparation, rRNA depletion, and sequencing were performed by Canada’s Michael Smith Genome Sciences Centre. In short, rRNA was depleted by using the bacterial NEBNext rRNA Depletion Kit (New England BioLabs, E7850). Sequencing libraries were created by end repair and phosphorylation, followed by 3’ A-addition and adapter ligation using a custom reagent formulation (New England BioLabs, E6000B–10). Libraries were pooled in equal molar amounts and were sequenced using an Illumina HiSeqX platform (Illumina) using paired-end (PE) reads.
RNA-seq data analysis workflow and pathway enrichment analysis
Reads were aligned to C. rodentium ICC168 reference genome (NC_013716, NC_013717, NC_013718, and NC_013719) by using STAR 2.7.10aCitation63 (v2.7.10a). The quality of the alignment was assessed using FastQCCitation31 (v0.11.9), MultiQCCitation64 (v1.12), and RSeQCCitation65 (v4.0.0). At least 9 million PE reads were obtained from each library. General alignment and quality statistics are available in Supplementary Data 1. Gene counts were imported into R (v4.1.0) for further processing (Supplementary Data 2). Only reads that were present in at least three samples with a count of 10 or higher were included for analysis. Differentially expressed genes (DEGs) were identified via DESeq2Citation66 using Independent Hypothesis Weighting (IHW) for p-value adjustmentCitation67 and ‘apeglm’ for log fold-change shrinkage.Citation68 Genes were considered differentially regulated when the comparison between ∆croI and WT passed an adjusted p-value of <0.05 and had a fold change equal to or greater than ±1.25 (Supplementary Data 3).
Pathway enrichment analysis was performed by using Gene Set Enrichment Analysis (GSEA, v4.2.3)Citation69,Citation70 using a pre-ranked list of all genes within the dataset (after pre-filtering) arranged according to the Wald-test statistic (Supplementary Data 4). Pathway annotation for C. rodentium was retrieved from the Kyoto Encyclopedia of Genes and Genomes database (KEGG; https://www.genome.jp/kegg/pathway.html). The analysis was performed using 100,000 gene set permutations using gene sets limits of 5–500. Only pathways that passed an FDR < 0.05 were considered to be significantly enriched. Module over-representation analysis was performed using a separate pre-ranked list for up- and down-regulated genes against the background universe of all detected genes (after pre-filtering) using ClusterProfilerCitation71 (v4.6.0) and a Benjamini–Hochberg (BH) adjusted p-value threshold of <0.05. Enrichment analysis data are available in Supplementary Data 5–6.
RT-qPCR analysis
C. rodentium was grown in 6-well plates with 3 mL of DMEM supplemented with either DMSO or 10 µM of C4-HSL for 6 h at 37°C with 5% CO2. RNA was isolated by using the Qiagen RNeasy Mini Kit; gDNA was depleted using the Turbo DNA-free kit (Invitrogen, AM1907). Reverse Transcription was performed on 1 µg of RNA using a QuantiTect Reverse Transcription Kit (Qiagen), and qPCR was performed using a QuantiNova SYBR Green PCR kit (Qiagen). Samples were analyzed in a ViiA 7 Real-Time PCR System (Applied Biosystems). All primer pairs used had an efficiency between 98% and 103% (data not shown); sequences are reported in Supplemental Table S5. Normalized fold expression was calculated by using the comparative CT methodCitation72 with dnaQ and recA as endogenous controls.
Type III secretion assay
Analysis of type III secreted proteins was performed as previously describedCitation25. In brief, C. rodentium strains were diluted in DMEM from an overnight culture in LB and grown statically for 6 h at 37°C with 5% CO2. Proteins secreted into the culture supernatant were precipitated using trichloroacetic acid, separated by a 16% SDS-PAGE gel, and stained using Coomassie Blue G250. Protein quantification was performed using the AzureSpot Analysis Software (Azure Biosystems).
Bacterial attachment to CMT-93 cells
Mouse intestinal epithelial cells (CMT-93; ATCC CCL-223) were grown and maintained in DMEM+++ at 37°C and 5% CO2. Before infection, cells were grown to confluence in a 12-well plate. A 3.5-h subculture of WT C. rodentium, deletion mutants, or complemented strains grown in LB to mid-log phase were used to infect cells to a multiplicity of infection (MOI) of 100. Upon inoculation, the plates were spun for 5 min at 1000 rpm to synchronize the infection. At 4-h post infection, cells were washed five times with PBS -/- to remove any non-adherent bacteria. Epithelial cells were lysed using 0.1% Triton X-100, and tissue adherent bacteria were quantified via CFU plating.
Bacterial growth curves
Overnight cultures of C. rodentium were inoculated into different media of interest to a final optical density of 0.005 in a 96-well plate. Bacterial growth was then monitored by measuring absorbance at 600 nm by using a plate reader. Cultures were grown at 37°C with continuous shaking, and measurements were taken every 10 min for 24 h.
Cytometric bead array (CBA) assay
At days 4 and 7 post-murine infection, cecal and colonic tissues were homogenized in 1 mL of PBS -/- supplemented with the cOmplete EDTA-free protease inhibitor cocktail (Roche Diagnostics). The supernatants were collected by centrifuging the homogenate at 16,000 ×g at 4°C for 20 min. Cytokine concentrations were determined using a Mouse Inflammation Cytometric Bead Array Kit (CBA; BD Biosciences) as per the manufacturer’s instructions. Samples were analyzed on an Attune NxT Flow Cytometer (ThermoFisher Scientific). Cytokine levels were normalized according to the sample weight.
Data and statistical analysis
Statistical analyses were performed using GraphPad Prism (v9.3.1) (www.graphpad.com) or R (v4.1.0). Data were reported as the mean ± SD unless otherwise indicated. Sample size or number of replicates are indicated in the figure captions. When appropriate, significance was assessed by using a t-test or an ANOVA; for non-normal distributed data a Kruskal–Wallis or Mann–Whitney test was used. Post-tests are reported in the figure captions. Statistical significance is reported as follows: *p ≤ 0.05; **p < 0.01; ***p < 0.001; ****p < 0.0001.
Author contributions
J.P.D. and B.B.F conceived the project. J.P.D., S.E.W., A.S.P., A.C.C., W.D., and S.O.J. performed and designed experiments. J.P.D., S.E.W., and A.C.C., analyzed data. J.P.D. wrote bioinformatics pipelines. J.P.D. wrote the original draft of the manuscript with input from all authors. All authors revised the manuscript. B.B.F. acquired funding for the project and provided supervision.
Supplemental Material
Download MS Word (11.9 MB)Supplemental Material
Download MS Excel (436.4 KB)Acknowledgments
The authors would like to thank all of our colleagues in the Finlay laboratory for their support and assistance. B.B.F. is a University of British Columbia Peter Wall Distinguished Professor. J Peña-Díaz received support from the University of British Columbia (UBC) and from Mitacs. Supporting images were created with BioRender (BioRender.com). This research was enabled in part by software provided by the Digital Research Alliance of Canada (alliancecan.ca). We are grateful to Matthew Croxen and Kirsten Koymans for generating the DBS100 ∆croI mutant. We would also like to thank Lisa Thorson for the fundamental logistical support of the project.
Disclosure statement
No potential conflict of interest was reported by the author(s).
Data availability statement
RNA-seq data generated from this study are available on the NCBI Sequence Read Archive (SRA) under the BioProject accession number PRJNA935367. The source data as well as any additional information necessary for the reanalysis of the data reported in this paper are available from the corresponding author upon reasonable request.
Supplementary material
Supplemental data for this article can be accessed online at https://doi.org/10.1080/19490976.2023.2267189
Additional information
Funding
References
- Vogt SL, Peña-Díaz J, Finlay BB. Chemical communication in the gut: effects of microbiota-generated metabolites on gastrointestinal bacterial pathogens. Anaerobe. 2015;34:106–19. doi:10.1016/j.anaerobe.2015.05.002.
- Woodward SE, Krekhno Z, Finlay BB. Here, there, and everywhere: how pathogenic Escherichia coli sense and respond to gastrointestinal biogeography. Cell Microbiol. 2019;21:1–15. doi:10.1111/cmi.13107.
- Storz G, Hengge R. Bacterial stress responses. 2nd ed. Washington, DC: American Society for Microbiology Press; 2011.
- Miller MB, Bassler BL. Quorum sensing in bacteria. Annu Rev Microbiol. 2001;55:165–199. doi:10.1146/annurev.micro.55.1.165.
- Waters CM, Bassler BL. Quorum sensing: cell-to-cell communication in bacteria. Annual review of cell and developmental biology. Annu Rev Cell Dev Biol. 2005;21:319–346. doi:10.1146/annurev.cellbio.21.012704.131001.
- Rutherford ST, Bassler BL. Bacterial quorum sensing: its role in virulence and possibilities for its control. Cold Spring harbor perspectives in medicine. Cold Spring Harb Perspect Med. 2012;2:a012427. doi:10.1101/cshperspect.a012427.
- Nealson KH, Hastings JW. Bacterial bioluminescence: its control and ecological significance. Microbiol Rev. 1979;43(4):496–518. doi:10.1128/mr.43.4.496-518.1979.
- Engebrecht J, Nealson K, Silverman M. Bacterial bioluminescence: isolation and genetic analysis of functions from Vibrio fischeri. Cell. 1983;32:773–781. doi:10.1016/0092-8674(83)90063-6.
- de Kievit TR, Iglewski BH, Portnoy DA. Bacterial quorum sensing in pathogenic relationships. Infect Immun. 2000;68:4839–4849. doi:10.1128/IAI.68.9.4839-4849.2000.
- Bronesky D, Wu Z, Marzi S, Walter P, Geissmann T, Moreau K, Vandenesch F, Caldelari I, Romby P. Staphylococcus aureus RNAIII and its regulon link quorum sensing, stress responses, metabolic adaptation, and regulation of virulence gene expression. Annu Rev Microbiol. 2016;70:299–316. doi:10.1146/annurev-micro-102215-095708.
- Davies DG, Parsek MR, Pearson JP, Iglewski BH, Costerton JW, Greenberg EP. The involvement of cell-to-cell signals in the development of a bacterial biofilm. Sci. 1998;280:295–298. doi:10.1126/science.280.5361.295.
- Hammer BK, Bassler BL. Quorum sensing controls biofilm formation in Vibrio cholerae: biofilms in V. cholerae. Mol Microbiol. 2003;50:101–104. doi:10.1046/j.1365-2958.2003.03688.x.
- Kong K-F, Vuong C, Otto M. Staphylococcus quorum sensing in biofilm formation and infection. Int J Med Microbiol. 2006;296:133–139. doi:10.1016/j.ijmm.2006.01.042.
- Fuqua C, Parsek MR, Greenberg EP. Regulation of gene expression by cell-to-cell communication: acyl-homoserine lactone quorum sensing. Annu Rev Genet. 2001;35:439–468. doi:10.1146/annurev.genet.35.102401.090913.
- Papenfort K, Bassler BL. Quorum sensing signal–response systems in gram-negative bacteria. Nat Rev Microbiol. 2016;14:576–588. doi:10.1038/nrmicro.2016.89.
- von Bodman SB, Willey JM, Diggle SP. Cell-cell communication in bacteria: united we stand. J Bacteriol. 2008;190:4377–4391. doi:10.1128/JB.00486-08.
- Swearingen MC, Sabag-Daigle A, Ahmer BMM. Are there acyl-homoserine lactones within mammalian intestines? J Bacteriol. 2013;195:173–179. doi:10.1128/JB.01341-12.
- Thompson JA, Oliveira R, Djukovic A, Ubeda C, Xavier KB. Manipulation of the quorum sensing signal AI-2 affects the antibiotic-treated gut microbiota. Cell Rep. 2015;10:1861–1871. doi:10.1016/j.celrep.2015.02.049.
- Coquant G, Grill J-P, Seksik P. Impact of N-Acyl-homoserine lactones, quorum sensing molecules, on gut immunity. Front Immunol. 2020;11:1827. doi:10.3389/fimmu.2020.01827.
- Coquant G, Aguanno D, Brot L, Belloir C, Delugeard J, Roger N, Pham H-P, Briand L, Moreau M, de Sordi L, et al. 3-oxo-C12: 2-HSL, quorum sensing molecule from human intestinal microbiota, inhibits pro-inflammatory pathways in immune cells via bitter taste receptors. Sci Rep. 2022;12(12):9440. doi:10.1038/s41598-022-13451-3.
- Hsiao A, Ahmed AMS, Subramanian S, Griffin NW, Drewry LL, Petri WA, Haque R, Ahmed T, Gordon JI. Members of the human gut microbiota involved in recovery from Vibrio cholerae infection. Nature. 2014;515:423–426. doi:10.1038/nature13738.
- Collins JW, Keeney KM, Crepin VF, Rathinam VAK, Fitzgerald KA, Finlay BB, Frankel G. Citrobacter rodentium: infection, inflammation and the microbiota. Nat Rev Microbiol. 2014;12:612–623. doi:10.1038/nrmicro3315.
- Crepin VF, Collins JW, Habibzay M, Frankel G. Citrobacter rodentium mouse model of bacterial infection. Nat Protoc. 2016;11:1851–1876. doi:10.1038/nprot.2016.100.
- Schauer DB, Falkow S. Attaching and effacing locus of a Citrobacter freundii biotype that causes transmissible murine colonic hyperplasia. Infect Immun. 1993;61:2486–2492. doi:10.1128/iai.61.6.2486-2492.1993.
- Deng W, Vallance BA, Li Y, Puente JL, Finlay BB. Citrobacter rodentium translocated intimin receptor (Tir) is an essential virulence factor needed for actin condensation, intestinal colonization and colonic hyperplasia in mice. Mol Microbiol. 2003;48:95–115. doi:10.1046/j.1365-2958.2003.03429.x.
- McDaniel TK, Jarvis KG, Donnenberg MS, Kaper JB. A genetic locus of enterocyte effacement conserved among diverse enterobacterial pathogens. Proc Natl Acad Sci USA. 1995;92:1664–1668. doi:10.1073/pnas.92.5.1664.
- Deng W, Li Y, Vallance BA, Finlay BB, O’Brien AD. Locus of enterocyte effacement from Citrobacter rodentium: sequence analysis and evidence for horizontal transfer among attaching and effacing pathogens. Infect Immun. 2001;69:6323–6335. doi:10.1128/IAI.69.10.6323-6335.2001.
- Deng W, Puente JL, Gruenheid S, Li Y, Vallance BA, Vázquez A, Barba J, Ibarra JA, O’Donnell P, Metalnikov P, Ashman, K., et al. Dissecting virulence: systematic and functional analyses of a pathogenicity island. Proc Natl Acad Sci USA. 2004;101:3597–3602. doi:10.1073/pnas.0400326101.
- Coulthurst SJ, Clare S, Evans TJ, Foulds IJ, Roberts KJ, Welch M, Dougan G, Salmond GPC. Quorum sensing has an unexpected role in virulence in the model pathogen Citrobacter rodentium. EMBO Rep. 2007;8(7):698–703. doi:10.1038/sj.embor.7400984.
- Hense BA, Schuster M. Core principles of bacterial autoinducer systems. Microbiol Mol Biol Rev. 2015;79(1):153–169. doi:10.1128/MMBR.00024-14.
- Andrews S. FastQC: A Quality Control Tool for High Throughput Sequence Data. 2010. http://www.bioinformatics.babraham.ac.uk/projects/fastqc/.
- Dong T, Schellhorn HE. Role of RpoS in virulence of pathogens. Infect Immun. 2010;78(3):887–897. doi:10.1128/IAI.00882-09.
- Spratt MR, Lane K, Dunlop M, Yount J. Navigating environmental transitions: the role of phenotypic variation in bacterial responses. mBio. 2022;13(6):e02212–22. doi:10.1128/mbio.02212-22.
- Contijoch EJ, Britton GJ, Yang C, Mogno I, Li Z, Ng R, Llewellyn SR, Hira S, Johnson C, Rabinowitz KM, et al. Gut microbiota density influences host physiology and is shaped by host and microbial factors. eLife. 2019;8:e40553. doi:10.7554/eLife.40553.
- Woodward SE, Vogt SL, Peña-Díaz J, Melnyk RA, Cirstea M, Serapio-Palacios A, Neufeld LMP, Huus KE, Wang MA, Haney CH, et al. Gastric acid and escape to systemic circulation represent major bottlenecks to host infection by Citrobacter rodentium. ISME J. 2023;17(1):36–46. doi:10.1038/s41396-022-01321-9.
- Vallance BA, Deng W, Jacobson K, Finlay BB. Host susceptibility to the Attaching and effacing bacterial pathogen Citrobacter rodentium. Infect Immun. 2003;71(6):3443–3453. doi:10.1128/IAI.71.6.3443-3453.2003.
- Guttman JA, Li Y, Wickham ME, Deng W, Vogl AW, Finlay BB. Attaching and effacing pathogen-induced tight junction disruption in vivo. Cell Microbiol. 2006;8(4):634–645. doi:10.1111/j.1462-5822.2005.00656.x.
- Dyszel JL, Smith JN, Lucas DE, Soares JA, Swearingen MC, Vross MA, Young GM, Ahmer BMM. Salmonella enterica serovar typhimurium can detect acyl homoserine lactone production by Yersinia enterocolitica in mice. J Bacteriol. 2010;192(1):29–37. doi:10.1128/JB.01139-09.
- Landman C, Grill J-P, Mallet J-M, Marteau P, Humbert L, Le Balc’h E, Maubert M-A, Perez K, Chaara W, Brot L, et al. Inter-kingdom effect on epithelial cells of the N-Acyl homoserine lactone 3-oxo-C12: 2, a major quorum-sensing molecule from gut microbiota. PLoS One. 2018;13(8):e0202587. doi:10.1371/journal.pone.0202587.
- Xue J, Chi L, Tu P, Lai Y, Liu C-W, Ru H, Lu K. Detection of gut microbiota and pathogen produced N-acyl homoserine in host circulation and tissues. NPJ Biofilms Microbio. 2021;7(1):53. 10.1038/s41522-021-00224–5. doi:10.1038/s41522-021-00224-5.
- Kim MK, Ingremeau F, Zhao A, Bassler BL, Stone HA. Local and global consequences of flow on bacterial quorum sensing. Nat microbiol. 2016;1(1):15005. doi:10.1038/nmicrobiol.2015.5.
- Siryaporn A, Kim MK, Shen Y, Stone HA, Gitai Z. Colonization, competition, and dispersal of pathogens in fluid flow networks. Curr Biol. 2015;25(9):1201–1207. doi:10.1016/j.cub.2015.02.074.
- Thomson NR, Crow MA, McGowan SJ, Cox A, Salmond GPC. Biosynthesis of carbapenem antibiotic and prodigiosin pigment in serratia is under quorum sensing control: production of antibiotic and pigment by serratia. Mol Microbiol. 2002;36(3):539–556. doi:10.1046/j.1365-2958.2000.01872.x.
- Ortori CA, Atkinson S, Chhabra SR, Cámara M, Williams P, Barrett DA. Comprehensive profiling of N-acylhomoserine lactones produced by Yersinia pseudotuberculosis using liquid chromatography coupled to hybrid quadrupole–linear ion trap mass spectrometry. Anal Bioanal Chem. 2007;387(2):497–511. doi:10.1007/s00216-006-0710-0.
- Gould TA, Herman J, Krank J, Murphy RC, Churchill MEA. Specificity of acyl-homoserine lactone synthases examined by mass spectrometry. J Bacteriol. 2006;188(2):773–783. doi:10.1128/JB.188.2.773-783.2006.
- Montebello AN, Brecht RM, Turner RD, Ghali M, Pu X, Nagarajan R. Acyl-ACP substrate recognition in Burkholderia mallei BmaI1 acyl-homoserine lactone synthase. Biochemistry. 2014;53(39):6231–6242. doi:10.1021/bi5009529.
- Arnoldini M, Vizcarra IA, Peña-Miller R, Stocker N, Diard M, Vogel V, Beardmore RE, Hardt W-D, Ackermann M, Balaban N. Bistable expression of virulence genes in salmonella leads to the formation of an antibiotic-tolerant subpopulation. PLoS Biol. 2014;12(8):e1001928. doi:10.1371/journal.pbio.1001928.
- Gorelik O, Levy N, Shaulov L, Yegodayev K, Meijler MM, Sal-Man N. Vibrio cholerae autoinducer-1 enhances the virulence of enteropathogenic Escherichia coli. Sci Rep. 2019;9(1):4122. doi:10.1038/s41598-019-40859-1.
- Subramoni S, Venturi V. LuxR-family ‘solos’: bachelor sensors/regulators of signalling molecules. Microbiology. 2009;155(5):1377–1385. doi:10.1099/mic.0.026849-0.
- Whiteley M, Diggle SP, Greenberg EP. Progress in and promise of bacterial quorum sensing research. Nature. 2017;551(7680):313–320. doi:10.1038/nature24624.
- Paluch E, Rewak-Soroczyńska J, Jędrusik I, Mazurkiewicz E, Jermakow K. Prevention of biofilm formation by quorum quenching. Appl Microbiol Biotechnol. 2020;104(5):1871–1881. doi:10.1007/s00253-020-10349-w.
- Zhu J, Miller MB, Vance RE, Dziejman M, Bassler BL, Mekalanos JJ. Quorum-sensing regulators control virulence gene expression in Vibrio cholerae. Proc Natl Acad Sci USA. 2002;99(5):3129–3134. doi:10.1073/pnas.052694299.
- Bridges AA, Bassler BL, Sourjik V. The intragenus and interspecies quorum-sensing autoinducers exert distinct control over Vibrio cholerae biofilm formation and dispersal. PLoS Biol. 2019;17(11):e3000429. doi:10.1371/journal.pbio.3000429.
- Krzyżek P. Challenges and limitations of anti-quorum sensing therapies. Front Microbiol. 2019;10:2473. doi:10.3389/fmicb.2019.02473.
- Gibson DG, Young L, Chuang R-Y, Venter JC, Hutchison CA, Smith HO. Enzymatic assembly of DNA molecules up to several hundred kilobases. Nat Methods. 2009;6(5):343–345. doi:10.1038/nmeth.1318.
- Ferriéres L, Hémery G, Nham T, Guérout AM, Mazel D, Beloin C, Ghigo JM. Silent mischief: bacteriophage mu insertions contaminate products of Escherichia coli random mutagenesis performed using suicidal transposon delivery plasmids mobilized by broad-host-range RP4 conjugative machinery. J Bacteriol. 2010;192(24):6418–6427. doi:10.1128/JB.00621-10.
- Donnenberg MS, Kaper JB. Construction of an eae deletion mutant of enteropathogenic Escherichia coli by using a positive-selection suicide vector. Infect Immun. 1991;59(12):4310–4317. doi:10.1128/iai.59.12.4310-4317.1991.
- Choi KH, Schweizer HP. Mini-Tn7 insertion in bacteria with single attTn7 sites: example Pseudomonas aeruginosa. Nat Protoc. 2006;1(1):153–161. doi:10.1038/nprot.2006.24.
- Duncan SH, Hold GL, Harmsen HJM, Stewart CS, Flint HJ. Growth requirements and fermentation products of Fusobacterium prausnitzii, and a proposal to reclassify it as faecalibacterium prausnitzii gen. nov., comb. nov. Int J Syst Evol Microbiol. 2002;52(6):2141–2146. doi:10.1099/00207713-52-6-2141.
- Shaw PD, Ping G, Daly SL, Cha C, Cronan JE, Rinehart KL, Farrand SK. Detecting and characterizing N -acyl-homoserine lactone signal molecules by thin-layer chromatography. Proc Natl Acad Sci USA. 1997;94(12):6036–6041. doi:10.1073/pnas.94.12.6036.
- Zhu J, Beaber JW, Moré MI, Fuqua C, Eberhard A, Winans SC. Analogs of the autoinducer 3-oxooctanoyl-homoserine lactone strongly inhibit activity of the TraR protein of agrobacterium tumefaciens. J Bacteriol. 1998;180(20):5398–5405. doi:10.1128/JB.180.20.5398-5405.1998.
- Bhagwat AA, Ying ZI, Karns J, Smith A. Determining RNA quality for NextGen sequencing: some exceptions to the gold standard rule of 23S to 16S rRNA ratio§. Microbiol Discov. 2013;1(1):10. doi:10.7243/2052-6180-1-10.
- Dobin A, Davis CA, Schlesinger F, Drenkow J, Zaleski C, Jha S, Batut P, Chaisson M, Gingeras TR. STAR: ultrafast universal RNA-seq aligner. Bioinformatics. 2013;29(1):15–21. doi:10.1093/bioinformatics/bts635.
- Ewels P, Magnusson M, Lundin S, Käller M. MultiQC: summarize analysis results for multiple tools and samples in a single report. Bioinformatics. 2016;32(19):3047–3048. doi:10.1093/bioinformatics/btw354.
- Wang L, Wang S, Li W. RSeQC: quality control of RNA-seq experiments. Bioinformatics. 2012;28(16):2184–2185. doi:10.1093/bioinformatics/bts356.
- Love MI, Huber W, Anders S. Moderated estimation of fold change and dispersion for RNA-seq data with DESeq2. Genome Biol. 2014;15(12):550. doi:10.1186/s13059-014-0550-8.
- Ignatiadis N, Klaus B, Zaugg JB, Huber W. Data-driven hypothesis weighting increases detection power in genome-scale multiple testing. Nat Methods. 2016;13(7):577–580. doi:10.1038/nmeth.3885.
- Zhu A, Ibrahim JG, Love MI, Stegle O. Heavy-tailed prior distributions for sequence count data: removing the noise and preserving large differences. Bioinformatics. 2019;35(12):2084–2092. doi:10.1093/bioinformatics/bty895.
- Subramanian A, Tamayo P, Mootha VK, Mukherjee S, Ebert BL, Gillette MA, Paulovich A, Pomeroy SL, Golub TR, Lander ES, Mesirov, J P., et al. Gene set enrichment analysis: a knowledge-based approach for interpreting genome-wide expression profiles. Proc Natl Acad Sci USA. 2005;102(43):15545–15550. doi:10.1073/pnas.0506580102.
- Mootha VK, Lindgren CM, Eriksson K-F, Subramanian A, Sihag S, Lehar J, Puigserver P, Carlsson E, Ridderstråle M, Laurila E, et al. PGC-1α-responsive genes involved in oxidative phosphorylation are coordinately downregulated in human diabetes. Nat Genet. 2003;34(3):267–273. doi:10.1038/ng1180.
- Wu T, Hu E, Xu S, Chen M, Guo P, Dai Z, Feng T, Zhou L, Tang W, Zhan L, et al. clusterProfiler 4.0: a universal enrichment tool for interpreting omics data. Innov. 2021;2(3):100141. doi:10.1016/j.xinn.2021.100141.
- Taylor SC, Nadeau K, Abbasi M, Lachance C, Nguyen M, Fenrich J. The ultimate qPCR Experiment: producing publication quality, reproducible data the first time. Trends Biotechnol. 2019;37(7):761–774. doi:10.1016/j.tibtech.2018.12.002.