ABSTRACT
The complex symbiotic relationship between the mammalian body and gut microbiome plays a critical role in the health outcomes of offspring later in life. The gut microbiome modulates virtually all physiological functions through direct or indirect interactions to maintain physiological homeostasis. Previous studies indicate a link between maternal/early-life gut microbiome, brain development, and behavioral outcomes relating to social cognition. Here we present direct evidence of the role of the gut microbiome in brain development. Through magnetic resonance imaging (MRI), we investigated the impact of the gut microbiome on brain organization and structure using germ-free (GF) mice and conventionalized mice, with the gut microbiome reintroduced after weaning. We found broad changes in brain volume in GF mice that persist despite the reintroduction of gut microbes at weaning. These data suggest a direct link between the maternal gut or early-postnatal microbe and their impact on brain developmental programming.
Introduction
The mammalian body is inhabited by a myriad of microorganisms originating from the vertical transfer of vaginal-perianal microbes during delivery.Citation1,Citation2 The complex symbiotic relationship rapidly established after birth between the offspring and the microorganisms hosted,Citation3 particularly within the gastrointestinal system, is critical for the establishment and maturation of immune functions,Citation4 metabolism,Citation5,Citation6 and the modulation of physiological homeostasis.Citation7,Citation8 Through direct anatomical connection, indirect endocrine means, or the mobilization of the immune system, microbial metabolites from the GI tract can modulate a broad range of physiological functions.Citation9,Citation10
It is increasingly apparent that the gut microbiota is a direct, modifiable factor of neurodevelopment and neurological outcomes in humans. Early exposure to antibiotics, which alters microbiome diversity,Citation11,Citation12 is associated with an increased risk of cognitive and neurodevelopmental disorders such as attention-deficit hyperactivity disorder and autism spectrum disorder.Citation13,Citation14 Fecal transplantation performed on germ-free (GF) dams using human maternal microbes from preterm mothers suggests poor growth outcomes.Citation15 Interestingly, there is a correlation between the time and duration of antibiotic exposure and the severity of cognitive deficits later in life,Citation16 and the levels of gut microbe by-products 3,4-dihydroxyphenylacetic acid and sodium butyrate are positively associated with mental healthCitation17,Citation18 and adult neurogenesis.Citation19
Experiments using the offspring of germ-free GF and antibiotic-treated dams further revealed that planar indoles and amine oxides produced by gut microbes regulate the expression of genes involved neurogenesis, neuronal differentiation, and axonogenesis.Citation20,Citation21 Alternate gut bacteria-derived metabolites and by-products, such as the short-chain fatty acids and peptidoglycan is a direct modulator of the blood-brain barrier structure, neurogenesis, and neuronal maturation pathways.Citation22–26 In relation, Sven Pettersson and others observed that altered gut microbiome colonization and composition significantly impact cognitive behavior in mice,Citation27–31 with a preferential effect on social behavior. Although pieces of information point to the involvement of gut microbiota in the modulation of brain development and function,Citation32 there is a lack of direct evidence of gut microbiome-dependent change in brain development.
Preclinical magnetic resonance imaging (MRI) methods provide a unique opportunity for a complete brain structure examination under the defined absence of gut microbiome. In this study, we examined the impact of early life absence of gut microbiome on brain development through structural MRI between control, specific-pathogen-free mice carrying normal microbiome population and the GF mice (). Alterations in isocortex and olfactory cortex volumes associated with higher-order cognitive control ensued from the complete depletion of microorganisms sustained through a restriction in environmental exposure. Additionally, the conventionalization of young adult GF mice failed to normalize the brain changes observed. These observations provide a premise for future functional and neurocircuitry studies to explore the structural determinants of cognitive deficits associated with early-life disturbance in the gut microbiome.
Results
GF mice exhibit distinct brain volume changes compared with age-matched SPF mice
Associations between early-life microbiome dysbiosis and later-stage cognitive deficits suggest neurodevelopmental deviations under gnotobiotic conditions.Citation16,Citation33,Citation34 To identify brain regions modulated by commensal bacteria, volumetric analysis of 6 to 8 weeks old SPF and GF mouse brains was achieved with MRI. Corrected statistical maps depicting significant group differences (p corrected < 0.05) are shown with an anatomical underlay. We observed a widespread alteration in brain volume in the GF versus the SPF mouse brain (). Notably, the absence of microbiome results in a significant bilateral increase in the olfactory cortex and isocortical brain volumes (AP + 1.98 to −3.28, orange-red sections) and a decrease in the sensory-related superior colliculus (SCs; AP −4.96), thalamus (TH, AP −2.8), and hypothalamus (AP −0.94; blue-green sections) in the GF compared with SPF mouse (). Specifically, there is a significant increase in the volumes of the somatomotor (MO, includes MOp and MOs, 0.044 ± 0.006, p =.016), somatosensory (SSp, includes SSp-bfd, SSp-ul, SSp-ll, SSp-m, SSp-tr and SSp-n, 0.073 ± 0.014, p =.016; SSs 0.073 ± 0.010, p =.008), anterior cingulate cortex (ACA, including ACAd and ACAv, p =.016), prelimbic area (PL 0.105 ± 0.026, p =.032), ectorhinal area (ECT 0.068 ± 0.009, p =.008), frontal pole of cerebral cortex (FRP 0.074 ± 0.010, p =.008), temporal association areas (TEa 0.063 ± 0.017, p =.016) and the perihinal area (PERI 0.072 ± 0.014, p =.016) of the isocortex, and the anterior olfactory nucleus (AON 0.094 ± 0.017, p =.032) and taenia tecta (TT, includes TTv and TTd, 0.101 ± 0.015, p =.008) of the olfactory cortex in GF mice ( and Supplementary Figure S1).
Figure 1. Widespread alterations in the subcortical brain volume of germ free (GF) mice.
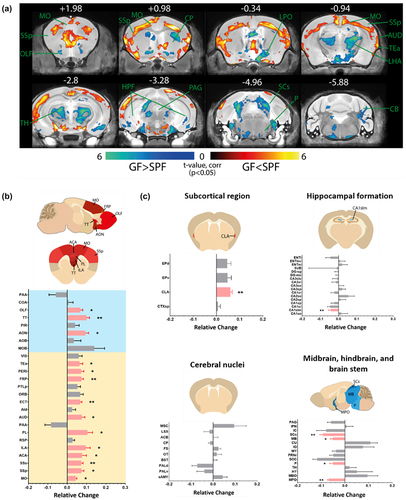
Conventionalization post-weaning does not reverse major brain volume changes observed in GF mice
To further understand the brain volume differences observed and examine the impact of the absence of gut microbiome on brain volume changes, we reviewed the effect of reintroducing gut microbiome post-weaning (conventionalization) on brain volumes of GF mice (CONV) compared against age-matched SPF mice (). Despite having a fecal bacterial population resembling the donor SPF mice post conventionalization,Citation35 brain areas altered in CONV mice are similar to that of GF mice compared against SPF mice (; MO 0.054 ± 0.008, p =.016; SSp 0.069 ± 0.006, p =.008; SSs 0.052 ± 0.015, p =.008; ACA 0.090 ± 0.011, p =.008; PL 0.145 ± 0.032, p =.008; ECT 0.064 ± 0.006; p =.008; FRP 0.116 ± 0.025, p =.032; TEa 0.044 ± 0.011, p =.032; PERI 0.079 ± 0.004, p =.008; AON 0.125 ± 0.011, p =.008; TT 0.107 ± 0.017, p =.008), and that volumes of these regions are comparable between CONV and GF mice (, Supplementary Figure S1; MO 0.009 ± 0.008, p =.421; SSp −0.004 ± 0.006, p >.999; SSs −0.020 ± 0.014, p =.421; ACA 0.011 ± 0.010, p >.999; PL 0 ± 0.010, p =.548; ECT 0 ± 0.008, p =.691; FRP 0 ± 0.009; p =.151; TEa −0.018 ± 0.010, p =.421; PERI 0 ± 0.013, p =.841; AON 0.028 ± 0.011, p =.222; TT 0.005 ± 0.016, p >.999).
Figure 2. Conventionalisation of GF mice post-weaning does not reverse majority of the brain volume changes in GF mice.
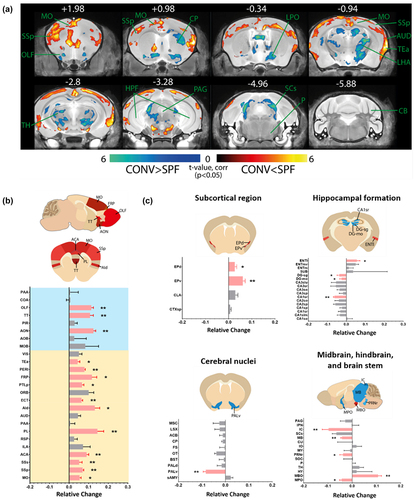
Figure 3. Brain regions sensitive toward gut microbe-dependent biochemical changes are affected by the reintroduction of gut microbiome.
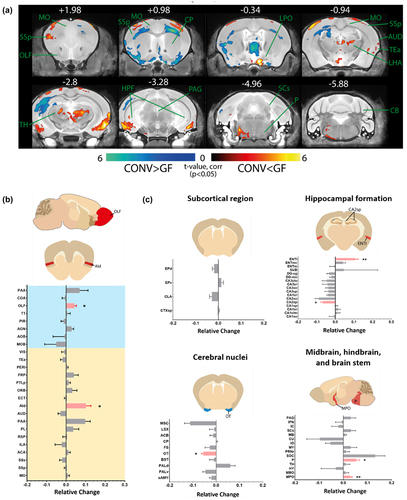
The reintroduction of gut microbiome influenced brain regions sensitive towards gut microbe-dependent biochemical perturbations
Gut microbiome disturbances can induce permanent alterations in key neurological processes and brain development or transient, reversible fluctuations in brain volume through neurological effects by changes in the systemic level of bacteria-dependent metabolites,Citation36 neurotransmitters,Citation37,Citation38 cytokine,Citation39 and endocrinal signals.Citation40 .The reintroduction of microorganisms postnatally is sufficient to normalize the subcortical claustrum (CLA SPF vs GF 0.060 ± 0.008, p =.008; SPF vs CONV 0.029 ± 0.011, p =.151), the isocortex infralimbic area (ILA SPF vs GF 0.096 ± 0.023, p = .016; SPF vs CONV 0.071 ± 0.04, p = .222), auditory area (AUD SPF vs GF 0.076 ± 0.016, p =.032; SPF vs CONV 0.045 ± 0.014, p =.095), hippocampal formation CA1 region (CA1slm SPF vs GF −0.043 ± 0.012, p =.008; SPF vs CONV 0.010 ± 0.015, p =.056), the pons (P SPF vs GF −0.084 ± 0.014, p =.016; SPF vs CONV −0.009 ± 0.020, p =.548), and the brain stem superior colliculus (SCs SPF vs GF −0.093 ± 0.012, p =.008; SPF vs CONV −0.065 ± 0.018, p =.056) (). Surprisingly, we observed a significant alteration in the thalamic nuclei (AP-0.34 to −2.8) and pons (AP −4.96) of the CONV mouse versus GF mouse brain () and alterations in previous unaffected dorsal agranular insular area (; Aid), posterior parietal association area (PTLp), and lateral entorhinal area (; ENTl), endopiriform nucleus (EP consisting of EPd and EPv), dentate gyrus (DG), and the ventral pallidum (PALv). The fiber tract and ventricular system are unaffected by the altered gut microbiome composition (Supplementary Figure S2).
Discussion
Epidemiological studies have reported a possible correlation between alterations in the composition of the gut microbiome and increased risk of neurodevelopmental and neuropsychiatric disorders.Citation13,Citation41 Preclinical animal studies further showed that exposure to gut microbial products can alter key neurodevelopmental processes.Citation20,Citation21,Citation42,Citation43 However, the direct contribution of the gut microbiota and neurocognitive outcomes remains inconclusive due to a lack of understanding of the underlying mechanisms.Citation44–46 Here, we present evidence for an association between structural alterations in the olfactory cortex and isocortex of the GF mice () and the neonatal gut microbe’s regulated developmental structural changes.
Figure 4. Ablation of gut microbiota persistently affects brain development in young adult mice.
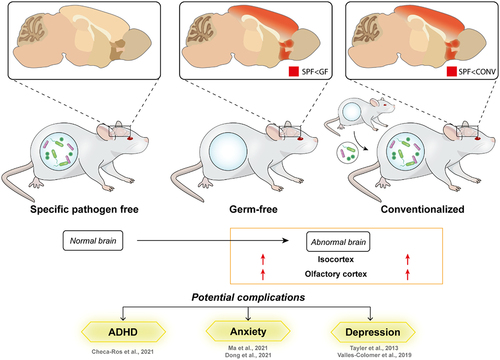
The increase of interest in the effects of intestinal microbiota on human health has led to extensive metagenome sequencing efforts to understand the variation and diversity between organisms and health conditionsCitation47–50 and the generation of preclinical models to elucidate the gut microbiome mechanism of action.Citation51–53 Further research has clarified the contribution of the gut in the brain function of adults through the direct activation of the vagus nerve,Citation54 the transfer of bacteria metabolites into the nervous systemCitation55, or the regulation of immune activation.Citation56 The use of GF mice provides the opportunity to decipher the role of the gut microbiota and regulation of brain development and function, relatively less explored.
Brain volume change is a powerful correlate of neurodevelopmental and neurocognitive outcomes of individuals,Citation57,Citation58 and MRI is a noninvasive method that allows for the complete examination of brain structure changes in a single experimental run. We observed broad structural volume alteration specific to the somatosensory in GF mice, critical for general and higher-order sensory processing and integrationCitation59–63 (, and Supplementary Figure S1). This is consistent with previous reports detailing defects in sensory processing in neuropsychiatric conditions associated with a disturbed gut microbiome.Citation13,Citation64,Citation65 In addition, changes in the ILA and ACA associates with contextual fear conditioningCitation66 and the modulation of fear and anxietyCitation67–69 correspond to altered anxiety behavior commonly observed in GF mice.Citation27,Citation28,Citation70 Previous research on similar-aged GF mice suggests that a combination of alterations in microglia colonization, cell survival,Citation71 and white matter developmentCitation72,Citation73 contributes to gut microbe-dependent brain morphological development and maturation.
Furthermore, there is a directed increase in the volumes of the AON and TT of the olfactory cortex (, and Supplementary Figure S1), which function as areas for olfactory information processing and integration.Citation74 Although relatively less explored, olfactory compromises are often observed in autism spectrum disorder patientsCitation75,Citation76 and correlates with the development of depressionCitation77 and schizophrenia.Citation78 The olfactory nerve undergoes a distinct developmental process,Citation79 thought to involve the function of yet unknown odorant receptors,Citation80 which may be directly responsive to gut microbiome-produced metabolites such as short-chain fatty acids and volatile amines that functions as odorants.Citation81,Citation82 A deep examination of the interactions between gut microbiome activities and the olfactory system is required to understand the relevance of microbiome disturbance in the modulation of olfactory development and behavior.
Gut microbe metabolites were shown to regulate the expression of genes and processes involved in neural stem cell differentiation, neurogenesis, and development.Citation20,Citation21,Citation83 Changes in cerebral metabolism,Citation84 expression of plasticity,Citation27 growth factorCitation15 or myelin-related genes,Citation85 and activity-related transcriptional pathwaysCitation86 were related to changes in the amount of brain structure in the prefrontal cortex observed in GF mice. Also, gut-derived neuroactive substances such as tryptophan and serotonin modulate the inflammatory status and function of the microglia in the central nervous system (CNS).Citation87,Citation88 Due to the time-sensitivity of the production of morphogens,Citation89 and the vulnerability of the neurological system to changes during key neurodevelopmental stages, neurological deviations can lead to persistent structural, functional, or cognitive disability. Specifically, the neurological impact of microbiome disturbance early in life correlates with later cognitive outcomes in alternate GF mouse modelsCitation71 and human infants,Citation90 similar to our observations that the recolonization of gut microbiome does not reverse detected brain changes. In rodents, key neurodevelopmental milestones are concluded by a month of age (postnatal),Citation91 corresponding to the timepoint of gut microbiome reintroduction to the GF mice in this study. Notably, reintroducing the gut microbiome through conventionalization at 3 to 4 weeks of age cannot reverse the majority of observed brain alterations (), in line with a previous report suggesting that behavioral changes in NMRI mice can only be reversed with postnatal colonization of microbes from females transplanted with microbes before pregnancy.Citation27 The conventionalization of GF mice is nonetheless sufficient to evoke changes in the colliculus superior (SCs) and hippocampus (Supplementary Figure S2), reflecting their sensitivity toward metabolic alterations.Citation92–94
We recognize that brain volume changes are dynamic and can be affected by short-term deviations in neurophysiological conditions.Citation95–97 Furthermore, it is not possible to distinguish the role of maternal gestational microbiota and early postnatal microbiome in brain volume changes observed, and the brain volume alterations do not reveal the underlying structural vulnerability responsible for the cognitive correlates observed in individuals with gut microbiome disturbance. It is unclear how the difference in weight and body fat content of GF mice from an altered gut microbiome-dependent metabolic profile may contribute to changes in brain development.Citation98 The involvement of sex-dependent hormones in shaping gut microbiome composition alongside neurodevelopment and brain structure maturation requires further evaluation.Citation72,Citation99,Citation100 Treatment of neurodevelopmental disorders in later stages of life is a possible concept for the normalization of neuronal network function without impacting the underlying defect in consideration of adult neurological plasticity. Further work is required to investigate the mechanisms involved in the observations made in the current report.
Materials and methods
Animal welfare
All animal procedures were carried out per institutional guidelines by Nanyang Technological University and approved by Regional Animal Research Ethical Board, Institutional Animal Care and Use Committee, Singapore. Male NMRI (Naval Medical Research Institute) mice, 6–8 weeks old, are used for MRI analysis in this study. The Germ-free mouse used was provided by Professor Sven Pettersson. The mouse colony is initially obtained from the Core Facility for Germ-free Research, Karolinska Institutet, and maintained in the gnotobiotic facility of the Nanyang Technological University before their use in experiments. All mice were kept under a 12-h light/dark cycle and provided with water and food (autoclaved R36 Lactamin) ad libitum. GF mice were placed and raised in special sterile plastic isolators. The isolator sterility was analyzed weekly through the bacterial and fungal culture of fecal homogenates of GF mice. Part of the GF mice population was conventionalized (CONV) after weaning (between 3 to 4 weeks of age) by gavage of 100 μl of specific pathogen-free (SPF) mouse fecal homogenate (dissolving two fecal pellets from SPF mice in 1 ml of phosphate-buffered saline) and cohoused with SPF mice for 21 days before being euthanized. 5 mice from each group are used in this study. No subjects were excluded from the analysis.
MRI acquisition and data processing
Mouse brains are imaged postmortem in situ.Citation101 Data were acquired with a Bruker 11/16 Biospec spectrometer (Bruker BioSpin MRI, Ettlingen, Germany) operating at 11.75 T, equipped with a BGA-S gradient system, a linear volume resonator coil for transmission, and a two-by-two phased-array cryocoil surface receiver coil. Images were acquired using Paravision 6 software. Localizer images were acquired to ensure the correct positioning of mice to the coil and the magnet isocentre. 3D volumetric scanning occurred for 8h30 min, with scans acquired using spin echo RARE sequenceCitation102 with a field of view saturation slice positioned on the inferior portion of the head and acquisition parameters: repetition time = 2500 ms, echo time = 5.1 ms, RARE factor = 10, matrix size 250 × 225 × 250, and field of view = 20 × 18 × 20 mm3, average = 2, achieving a resolution of 0.08 mm3.
Anatomical scans were processed through voxel-based morphometry using ANTS (Advanced Normalization Tools 2.4.0, picsl.upenn.edu/software/ants). Briefly, images were first corrected for intensity field inhomogeneity using the N4 algorithm. Second, a study template was estimated by realigning individual volumes to each other over 3 iterative steps of non-linear transformation. Third, the individual volumes underwent SyN diffeomorphic image registration to the study template. Fourth, the Jacobian determinants were estimated and voxel-wise comparisons between groups were carried out with a two-sample t-test using FSL 6.0.5 (fsl_glm) and corrected for multiple hypothesis testing using the easy threshold function.
Statistical analysis
Jacobian determinants were extracted using the DSURQE mouse brain atlas.Citation103 All data are expressed as mean ± standard error mean (SEM) and ported to GraphPad Prism 7.04 (GraphPad Software) for analysis. Datasets were tested for normal distribution with the Shapiro-Wilk test. Further analysis was conducted with the Mann-Whitney U test between SPF, GF, and CONV datasets. P values <.05 were considered statistically significant.
Authors’ contributions
Conceptualisation, X.Y.Y., S.P., and S.J.; data acquisition and analysis, J.G., X.Y.Y., and H.U.L., B.H.; writing – original draft preparation. X.Y.Y., W.R.C., and S.J.; writing – review and editing, X.Y.Y., S.P., J.G., W.H., and S.J.; supervision, S.J.; funding acquisition S.P., W.H., and S.J.; critical revision of manuscript, S.J., S.P., W.H., and J.G. All authors have read and agreed to the published version of the manuscript.
Availability of data and materials
The raw datasets generated for this study are available in http://doi:10.18112/openneuro.ds004254.v1.0.0. The programming code used for data preprocessing and analysis can be found at https://gitlab.socsci.ru.nl/preclinical-neuroimaging/germfree. Further inquiries can be directed to the corresponding authors.
Ethics approval
All animal procedures were carried out per institutional guidelines by Nanyang Technological University and approved by Regional Animal Research Ethical Board, Institutional Animal Care and Use Committee, Singapore.
Supplemental Material
Download Zip (791.8 KB)Disclosure statement
The authors declare that the research was conducted in the absence of any commercial or financial relationships that could be construed as a potential conflict of interest.
Supplementary material
Supplemental data for this article can be accessed online at https://doi.org/10.1080/19490976.2023.2283911
Additional information
Funding
References
- Dominguez-Bello MG, Costello EK, Contreras M, Magris M, Hidalgo G, Fierer N, Knight R. Delivery mode shapes the acquisition and structure of the initial microbiota across multiple body habitats in newborns. Proc Natl Acad Sci U S A. 2010 Jun 29;107(26):11971–14. doi:10.1073/pnas.1002601107.
- Grölund MM, Lehtonen OP, Eerola E, Kero P. Fecal microflora in healthy infants born by different methods of delivery: permanent changes in intestinal flora after cesarean delivery. J Pediatr Gastroenterol Nutr. 1999 Jan;28(1):19–25. doi:10.1097/00005176-199901000-00007.
- Henrick BM, Rodriguez L, Lakshmikanth T, Pou C, Henckel E, Arzoomand A, Olin A, Wang J, Mikes J, Tan Z, et al. Bifidobacteria-mediated immune system imprinting early in life. Cell. 2021 Jul;184(15):3884–3898.e11. doi:10.1016/j.cell.2021.05.030.
- Wu HJ, Wu E. The role of gut microbiota in immune homeostasis and autoimmunity. Gut Microbes. 2012 Jan;3(1):4–14. doi:10.4161/gmic.19320.
- Martin AM, Sun EW, Rogers GB, Keating DJ. The influence of the gut microbiome on host metabolism through the regulation of gut hormone release. Front Physiol. 2019 Apr 16;10:428. doi:10.3389/fphys.2019.00428.
- Agus A, Clément K, Sokol H. Gut microbiota-derived metabolites as central regulators in metabolic disorders. Gut. 2021 Jun;70(6):1174–1182. doi:10.1136/gutjnl-2020-323071.
- Rhys-Jones D, Climie RE, Gill PA, Jama HA, Head GA, Gibson PR, Kaye DM, Muir JG, Marques FZ. Microbial interventions to control and reduce blood pressure in Australia (MICRoBIA): rationale and design of a double-blinded randomised cross-over placebo controlled trial. Trials. 2021 Dec;22(1):496. doi:10.1186/s13063-021-05468-2.
- Matenchuk BA, Mandhane PJ, Kozyrskyj AL. Sleep, circadian rhythm, and gut microbiota. Sleep Med Rev. 2020 Oct;53:101340. doi:10.1016/j.smrv.2020.101340.
- Breit S, Kupferberg A, Rogler G, Hasler G. Vagus nerve as modulator of the brain–gut axis in psychiatric and inflammatory disorders. Front Psychiatry. 2018 Mar 13;9:44. doi:10.3389/fpsyt.2018.00044.
- Cryan JF, O’Riordan KJ, Cowan CSM, Sandhu KV, Bastiaanssen TFS, Boehme M, Codagnone MG, Cussotto S, Fulling C, Golubeva AV, et al. The microbiota-gut-brain axis. Physiol Rev. 2019 Oct 1;99(4):1877–2013. doi:10.1152/physrev.00018.2018.
- Gasparrini AJ, Wang B, Sun X, Kennedy EA, Hernandez-Leyva A, Ndao IM, Tarr PI, Warner BB, Dantas G. Persistent metagenomic signatures of early-life hospitalization and antibiotic treatment in the infant gut microbiota and resistome. Nat microbiol. 2019 Sep 9;4(12):2285–2297. doi:10.1038/s41564-019-0550-2.
- Bokulich NA, Chung J, Battaglia T, Henderson N, Jay M, Li HD, Lieber A, Wu F, Perez-Perez GI, Chen Y, et al. Antibiotics, birth mode, and diet shape microbiome maturation during early life. Sci Transl Med. 2016 Jun 15;8(343). [accessed 2022 Dec 19]. doi:10.1126/scitranslmed.aad7121.
- Slob EMA, Brew BK, Vijverberg SJH, Dijs T, van Beijsterveldt CEM, Koppelman GH, Bartels M, Dolan CV, Larsson H, Lundström S, et al. Early-life antibiotic use and risk of attention-deficit hyperactivity disorder and autism spectrum disorder: results of a discordant twin study. Int J Epidemiol. 2021 May 17;50(2):475–484. doi:10.1093/ije/dyaa168.
- Slykerman RF, Thompson J, Waldie KE, Murphy R, Wall C, Mitchell EA. Antibiotics in the first year of life and subsequent neurocognitive outcomes. Acta Paediatr. 2017 Jan;106(1):87–94. doi:10.1111/apa.13613.
- Lu J, Lu L, Yu Y, Cluette-Brown J, Martin CR, Claud EC. Effects of intestinal microbiota on brain development in humanized gnotobiotic mice. Sci Rep. 2018 Dec;8(1):5443. doi:10.1038/s41598-018-23692-w.
- Slykerman RF, Coomarasamy C, Wickens K, Thompson JMD, Stanley TV, Barthow C, Kang J, Crane J, Mitchell EA. Exposure to antibiotics in the first 24 months of life and neurocognitive outcomes at 11 years of age. Psychopharmacology. 2019 May;236(5):1573–1582. doi:10.1007/s00213-019-05216-0.
- Valles-Colomer M, Falony G, Darzi Y, Tigchelaar EF, Wang J, Tito RY, Schiweck C, Kurilshikov A, Joossens M, Wijmenga C, et al. The neuroactive potential of the human gut microbiota in quality of life and depression. Nat microbiol. 2019 Apr;4(4):623–632. doi:10.1038/s41564-018-0337-x.
- Kratsman N, Getselter D, Elliott E. Sodium butyrate attenuates social behavior deficits and modifies the transcription of inhibitory/excitatory genes in the frontal cortex of an autism model. Neuropharmacology. 2016 Mar;102:136–145. doi:10.1016/j.neuropharm.2015.11.003.
- Kundu P, Lee HU, Garcia-Perez I, Tay EXY, Kim H, Faylon LE, Martin KA, Purbojati R, Drautz-Moses DI, Ghosh S, et al. Neurogenesis and prolongevity signaling in young germ-free mice transplanted with the gut microbiota of old mice. Sci Transl Med. 2019 Nov 13;11(518):eaau4760. doi:10.1126/scitranslmed.aau4760.
- Wei GZ, Martin KA, Xing PY, Agrawal R, Whiley L, Wood TK, Hejndorf S, Ng YZ, Low JZY, Rossant J, et al. Tryptophan-metabolizing gut microbes regulate adult neurogenesis via the aryl hydrocarbon receptor. Proc Natl Acad Sci U S A. 2021 Jul 6;118(27):e2021091118. doi:10.1073/pnas.2021091118.
- Vuong HE, Pronovost GN, Williams DW, Coley EJL, Siegler EL, Qiu A, Kazantsev M, Wilson CJ, Rendon T, Hsiao EY, et al. The maternal microbiome modulates fetal neurodevelopment in mice. Nature. 2020 Oct 8;586(7828):281–286. doi:10.1038/s41586-020-2745-3.
- Sentürk A, Pfennig S, Weiss A, Burk K, Acker-Palmer A. Ephrin Bs are essential components of the reelin pathway to regulate neuronal migration. Nature. 2011 Apr 21;472(7343):356–360. doi:10.1038/nature09874.
- Kaul D, Habbel P, Derkow K, Krüger C, Franzoni E, Wulczyn FG, Bereswill S, Nitsch R, Schott E, Veh R, et al. Expression of toll-like receptors in the developing brain. Alexopoulou L, editor. PLoS ONE. 2012 May 30;7(5):e37767. doi:10.1371/journal.pone.0037767.
- Humann J, Mann B, Gao G, Moresco P, Ramahi J, Loh LN, Farr A, Hu Y, Durick-Eder K, Fillon S, et al. Bacterial peptidoglycan traverses the Placenta to induce fetal neuroproliferation and aberrant postnatal behavior. Cell Host & Microbe. 2016 Mar;19(3):388–399. doi:10.1016/j.chom.2016.02.009.
- Braniste V, Al-Asmakh M, Kowal C, Anuar F, Abbaspour A, Tóth M, Korecka A, Bakocevic N, Ng LG, Kundu P, et al. The gut microbiota influences blood-brain barrier permeability in mice. Sci Transl Med. 2014 Nov 19;6(263). [accessed 2023 Jul 10]. doi:10.1126/scitranslmed.3009759.
- Michel L, Prat A. One more role for the gut: microbiota and blood brain barrier. Ann Transl Med. 2016 Jan;4(1):15. doi:10.3978/j.issn.2305-5839.2015.10.16.
- Heijtz RD, Wang S, Anuar F, Qian Y, Björkholm B, Samuelsson A, Hibberd, ML, Forssberg, H, Pettersson, S. Normal gut microbiota modulates brain development and behavior. Proc Natl Acad Sci U S A. 2011 Feb 15;108(7):3047–3052. doi:10.1073/pnas.1010529108.
- Neufeld KM, Kang N, Bienenstock J, Foster JA. Reduced anxiety-like behavior and central neurochemical change in germ-free mice: behavior in germ-free mice. Neurogastroent Motil. 2011 Mar;23(3):255–e119. doi:10.1111/j.1365-2982.2010.01620.x.
- Agranyoni O, Meninger-Mordechay S, Uzan A, Ziv O, Salmon-Divon M, Rodin D, Raz O, Koman I, Koren O, Pinhasov A, et al. Gut microbiota determines the social behavior of mice and induces metabolic and inflammatory changes in their adipose tissue. NPJ Biofilms Microbio. 2021 Mar 19;7(1):28. doi:10.1038/s41522-021-00193-9.
- Desbonnet L, Clarke G, Shanahan F, Dinan TG, Cryan JF. Microbiota is essential for social development in the mouse. Mol Psychiatry. 2014 Feb;19(2):146–148. doi:10.1038/mp.2013.65.
- Wu WL, Adame MD, Liou CW, Barlow JT, Lai TT, Sharon G, Schretter CE, Needham BD, Wang MI, Tang W, et al. Microbiota regulate social behaviour via stress response neurons in the brain. Nature. 2021 Jul 15;595(7867):409–414. doi:10.1038/s41586-021-03669-y.
- Aswendt M, Green C, Sadler R, Llovera G, Dzikowski L, Heindl S, Gomez de Agüero M, Diedenhofen M, Vogel S, Wieters F, et al. The gut microbiota modulates brain network connectivity under physiological conditions and after acute brain ischemia. iScience. 2021 Oct;24(10):103095. doi:10.1016/j.isci.2021.103095.
- Guernier V, Brennan B, Yakob L, Milinovich G, Clements ACA, Soares Magalhaes RJ. Gut microbiota disturbance during helminth infection: can it affect cognition and behaviour of children? BMC Infect Dis. 2017 Dec;17(1):58. doi:10.1186/s12879-016-2146-2.
- Tamana SK, Tun HM, Konya T, Chari RS, Field CJ, Guttman DS, Becker AB, Moraes TJ, Turvey SE, Subbarao P, et al. Bacteroides-dominant gut microbiome of late infancy is associated with enhanced neurodevelopment. Gut Microbes. 2021 Jan 1;13(1):1930875. doi:10.1080/19490976.2021.1930875.
- El Aidy S, Derrien M, Merrifield CA, Levenez F, Doré J, Boekschoten MV, Dekker J, Holmes E, Zoetendal EG, van Baarlen P, et al. Gut bacteria–host metabolic interplay during conventionalisation of the mouse germfree colon. ISME J. 2013 Apr;7(4):743–755. doi:10.1038/ismej.2012.142.
- Rea V, Bell I, Ball T, Van Raay T. Gut-derived metabolites influence neurodevelopmental gene expression and wnt signaling events in a germ-free zebrafish model. Microbiome. 2022 Aug 23;10(1):132. doi:10.1186/s40168-022-01302-2.
- Otaru N, Ye K, Mujezinovic D, Berchtold L, Constancias F, Cornejo FA, Krzystek A, de Wouters T, Braegger C, Lacroix C, et al. GABA production by human intestinal bacteroides spp.: prevalence, regulation, and role in acid stress tolerance. Front Microbiol. 2021 Apr 15;12:656895. doi:10.3389/fmicb.2021.656895.
- Tang X, Jaenisch R, Sur M. The role of GABAergic signalling in neurodevelopmental disorders. Nat Rev Neurosci. 2021 May;22(5):290–307. doi:10.1038/s41583-021-00443-x.
- Salvo E, Stokes P, Keogh CE, Brust-Mascher I, Hennessey C, Knotts TA, Sladek JA, Rude KM, Swedek M, Rabasa G, et al. A murine model of pediatric inflammatory bowel disease causes microbiota-gut-brain axis deficits in adulthood. Am J Physiol-Gastr L. 2020 Sep 1;319(3):G361–74. doi:10.1152/ajpgi.00177.2020.
- Schalla MA, Stengel A. Effects of microbiome changes on endocrine ghrelin signaling – a systematic review. Peptides. 2020 Nov;133:170388. doi:10.1016/j.peptides.2020.170388.
- Chevalier G, Siopi E, Guenin-Macé L, Pascal M, Laval T, Rifflet A, Boneca IG, Demangel C, Colsch B, Pruvost A, et al. Effect of gut microbiota on depressive-like behaviors in mice is mediated by the endocannabinoid system. Nat Commun. 2020 Dec;11(1):6363. doi:10.1038/s41467-020-19931-2.
- Abdelli LS, Samsam A, Naser SA. Propionic acid induces Gliosis and Neuro-inflammation through modulation of PTEN/AKT pathway in autism spectrum disorder. Sci Rep. 2019 Jun 19;9(1):8824. doi:10.1038/s41598-019-45348-z.
- Erny D, Hrabě de Angelis AL, Jaitin D, Wieghofer P, Staszewski O, David E, Keren-Shaul H, Mahlakoiv T, Jakobshagen K, Buch T, et al. Host microbiota constantly control maturation and function of microglia in the CNS. Nat Neurosci. 2015 Jul;18(7):965–977. doi:10.1038/nn.4030.
- Goodrich JK, Waters JL, Poole AC, Sutter JL, Koren O, Blekhman R, Beaumont M, Van Treuren W, Knight R, Bell J, et al. Human genetics shape the gut microbiome. Cell. 2014 Nov;159(4):789–799. doi:10.1016/j.cell.2014.09.053.
- Rosenfeld CS. Gut dysbiosis in animals due to environmental chemical exposures. Front Cell Infect Microbiol. 2017 Sep 8;7:396. doi:10.3389/fcimb.2017.00396.
- Ramirez J, Guarner F, Bustos Fernandez L, Maruy A, Sdepanian VL, Cohen H. Antibiotics as Major Disruptors of Gut Microbiota. Front Cell Infect Microbiol. 2020 Nov 24;10:572912. doi:10.3389/fcimb.2020.572912.
- Bai X, Narayanan A, Nowak P, Ray S, Neogi U, Sönnerborg A. Whole-Genome Metagenomic analysis of the gut microbiome in HIV-1-Infected individuals on antiretroviral therapy. Front Microbiol. 2021 Jun 25;12:667718. doi:10.3389/fmicb.2021.667718.
- Kim CY, Lee M, Yang S, Kim K, Yong D, Kim HR, Lee I. Human reference gut microbiome catalog including newly assembled genomes from under-represented Asian metagenomes. Genome Med. 2021 Dec;13(1):134. doi:10.1186/s13073-021-00950-7.
- Li F, Yang S, Zhang L, Qiao L, Wang L, He S, et al. Comparative metagenomics analysis reveals how the diet shapes the gut microbiota in several small mammals. Ecol Evol [Internet]. 2022 Jan;12(1):e8470. [accessed 2022 Jun 7]. doi:10.1002/ece3.8470.
- Kieser S, Zdobnov EM, Trajkovski M, Nagarajan N. Comprehensive mouse microbiota genome catalog reveals major difference to its human counterpart. Nagarajan N, editor. PLoS Comput Biol. 2022 Mar 8;18(3):e1009947. doi:10.1371/journal.pcbi.1009947.
- Kennedy EA, King KY, Baldridge MT. Mouse microbiota models: comparing germ-free mice and Antibiotics treatment as tools for modifying gut bacteria. Front Physiol. 2018 Oct 31;9:1534. doi:10.3389/fphys.2018.01534.
- Park JC, Im SH. Of men in mice: the development and application of a humanized gnotobiotic mouse model for microbiome therapeutics. Experimental & Molecular Medicine. 2020 Sep;52(9):1383–1396. doi:10.1038/s12276-020-0473-2.
- Kundu P, Blacher E, Elinav E, Pettersson S. Our Gut Microbiome: The Evolving Inner Self. Cell. 2017 Dec;171(7):1481–1493. doi:10.1016/j.cell.2017.11.024.
- Han Y, Wang B, Gao H, He C, Hua R, Liang C, Zhang S, Wang Y, Xin S, Xu J. Vagus nerve and underlying impact on the gut microbiota-brain Axis in behavior and neurodegenerative diseases. JIR. 2022 Nov; 15;Volume 15:6213–6230. doi:10.2147/JIR.S384949.
- Gong Y, Chen A, Zhang G, Shen Q, Zou L, Li J, Miao Y-B, Liu W. Cracking brain diseases from gut microbes-mediated metabolites for precise treatment. Int J Biol Sci. 2023;19(10):2974–2998. doi:10.7150/ijbs.85259.
- Foster JA, Baker GB, Dursun SM. The relationship between the gut microbiome-immune system-brain Axis and major depressive disorder. Front Neurol. 2021 Sep 28;12:721126. doi:10.3389/fneur.2021.721126.
- Choe MS, Ortiz-Mantilla S, Makris N, Gregas M, Bacic J, Haehn D, Kennedy D, Pienaar R, Caviness VS, Benasich AA, et al. Regional infant brain development: an MRI-Based Morphometric analysis in 3 to 13 month olds. Cereb Cortex. 2013 Sep 1;23(9):2100–2117. doi:10.1093/cercor/bhs197.
- Cheong JLY, Thompson DK, Spittle AJ, Potter CR, Walsh JM, Burnett AC, Lee KJ, Chen J, Beare R, Matthews LG, et al. Brain volumes at term-equivalent age are associated with 2-year neurodevelopment in moderate and late preterm children. J Pediatr. 2016 Jul;174:91–97.e1. doi:10.1016/j.jpeds.2016.04.002.
- Gómez LJ, Dooley JC, Sokoloff G, Blumberg MS. Parallel and serial sensory processing in developing primary somatosensory and motor cortex. J Neurosci. 2021 Apr 14;41(15):3418–3431. doi:10.1523/JNEUROSCI.2614-20.2021.
- Laurienti PJ, Wallace MT, Maldjian JA, Susi CM, Stein BE, Burdette JH. Cross-modal sensory processing in the anterior cingulate and medial prefrontal cortices. Hum Brain Mapp. 2003 Aug;19(4):213–223. doi:10.1002/hbm.10112.
- Xerri C, Zennou-Azogui Y. Interplay between primary cortical areas and crossmodal plasticity. In: Heinbockel T Zhou Y, editors. Connectivity and functional specialization in the brain [internet]. IntechOpen; 2021 [accessed 2022 Aug 28]. https://www.intechopen.com/books/connectivity-and-functional-specialization-in-the-brain/interplay-between-primary-cortical-areas-and-crossmodal-plasticity
- Medinaceli Quintela R, Brunert D, Rothermel M. Functional role of the anterior olfactory nucleus in sensory information processing. Neuroforum. 2022 Aug 26;28(3):169–175. doi:10.1515/nf-2022-0008.
- Feigin L, Tasaka G, Maor I, Mizrahi A. Sparse Coding in temporal association cortex Improves complex Sound Discriminability. J Neurosci. 2021 Aug 18;41(33):7048–7064. doi:10.1523/JNEUROSCI.3167-20.2021.
- Dash S, Clarke G, Berk M, Jacka FN. The gut microbiome and diet in psychiatry: focus on depression. Curr Opin Psychiatry. 2015 Jan;28(1):1–6. doi:10.1097/YCO.0000000000000117.
- Habata K, Cheong Y, Kamiya T, Shiotsu D, Omori IM, Okazawa H, Jung M, Kosaka H. Relationship between sensory characteristics and cortical thickness/volume in autism spectrum disorders. Transl Psychiatry. 2021 Dec;11(1):616. doi:10.1038/s41398-021-01743-7.
- Tayler KK, Tanaka KZ, Reijmers LG, Wiltgen BJ. Reactivation of neural ensembles during the retrieval of recent and remote memory. Curr Biol. 2013 Jan;23(2):99–106. doi:10.1016/j.cub.2012.11.019.
- Jinks AL, McGregor IS. Modulation of anxiety-related behaviours following lesions of the prelimbic or infralimbic cortex in the rat. Brain Res. 1997 Oct;772(1–2):181–190. doi:10.1016/S0006-8993(97)00810-X.
- Schulz-Klaus B, Fendt M, Schnitzler HU. Temporary inactivation of the rostral perirhinal cortex induces an anxiolytic-like effect on the elevated plus-maze and on the yohimbine-enhanced startle response. Behav Brain Res. 2005 Sep;163(2):168–173. doi:10.1016/j.bbr.2005.04.022.
- Cádiz-Moretti B, Abellán-Álvaro M, Pardo-Bellver C, Martínez-García F, Lanuza E. Afferent and efferent connections of the cortex-amygdala Transition Zone in mice. Front Neuroanat [Internet]. 2016 Dec 23;10:125. [accessed 2022 Jun 7]. doi:10.3389/fnana.2016.00125.
- Liu HX, Rocha CS, Dandekar S, Wan YJY. Functional analysis of the relationship between intestinal microbiota and the expression of hepatic genes and pathways during the course of liver regeneration. J Hepatol. 2016 Mar;64(3):641–650. doi:10.1016/j.jhep.2015.09.022.
- Castillo-Ruiz A, Gars A, Sturgeon H, Ronczkowski NM, Pyaram DN, Dauriat CJG, Chassaing B, Forger, NG. Brain effects of gestating germ-free persist in mouse neonates despite acquisition of a microbiota at birth. Front Neurosci. 2023 May 3;17:1130347. doi:10.3389/fnins.2023.1130347.
- Lu J, Synowiec S, Lu L, Yu Y, Bretherick T, Takada S, Yarnykh V, Caplan J, Caplan M, Claud EC, et al. Microbiota influence the development of the brain and behaviors in C57BL/6J mice. Skoulakis EMC, editor. PLoS ONE. 2018 Aug 3;13(8):e0201829. doi:10.1371/journal.pone.0201829.
- Ahmed S, Travis SD, Díaz-Bahamonde FV, Porter DDL, Henry SN, Mykins J, Ravipati A, Booker A, Ju J, Ding H, et al. Early influences of microbiota on white matter development in germ-free piglets. Front Cell Neurosci. 2021 Dec 27;15:807170. doi:10.3389/fncel.2021.807170.
- Levinson M, Kolenda JP, Alexandrou GJ, Escanilla O, Cleland TA, Smith DM, Linster C. Context-dependent odor learning requires the anterior olfactory nucleus. Behav Neurosci. 2020 Aug;134(4):332–343. doi:10.1037/bne0000371.
- Xu M, Minagawa Y, Kumazaki H, Okada K-I, Naoi N. Prefrontal responses to odors in individuals with autism spectrum disorders: functional NIRS measurement combined with a fragrance pulse ejection system. Front Hum Neurosci. 2020 Oct 8;14:523456. doi:10.3389/fnhum.2020.523456.
- Koehler L, Fournel A, Albertowski K, Roessner V, Gerber J, Hummel C, Hummel T, Bensafi M. Impaired odor perception in autism spectrum disorder is associated with decreased activity in olfactory cortex. Chemical Senses. 2018 Sep 22;43(8):627–634. doi:10.1093/chemse/bjy051.
- Deems DA, Doty RL, Settle RG, Moore-Gillon V, Shaman P, Mester AF, Kimmelman CP, Brightman VJ, Snow JB. Smell and taste disorders, a study of 750 patients from the University of Pennsylvania smell and taste center. Arch Otolaryngol - Head Neck Surg. 1991 May 1;117(5):519–528. doi:10.1001/archotol.1991.01870170065015.
- Moberg P. Olfactory dysfunction in schizophrenia a qualitative and quantitative review. Neuropsychopharmacology. 1999 Sep;21(3):325–340. doi:10.1016/S0893-133X(99)00019-6.
- Balmer CW, LaMantia AS. Noses and neurons: induction, morphogenesis, and neuronal differentiation in the peripheral olfactory pathway. Dev Dyn. 2005 Nov;234(3):464–481. doi:10.1002/dvdy.20582.
- Feinstein P, Bozza T, Rodriguez I, Vassalli A, Mombaerts P. Axon Guidance of mouse olfactory sensory neurons by odorant receptors and the β2 Adrenergic receptor. Cell. 2004 Jun;117(6):833–846. doi:10.1016/j.cell.2004.05.013.
- Pluznick JL, Protzko RJ, Gevorgyan H, Peterlin Z, Sipos A, Han J, Brunet I, Wan L-X, Rey F, Wang T, et al. Olfactory receptor responding to gut microbiota-derived signals plays a role in renin secretion and blood pressure regulation. Proc Natl Acad Sci U S A. 2013 Mar 12;110(11):4410–4415. doi:10.1073/pnas.1215927110.
- Maraci Ö, Engel K, Caspers BA. Olfactory communication via microbiota: what is known in birds? Genes. 2018 Jul 31;9(8):387. doi:10.3390/genes9080387.
- Dash S, Syed YA, Khan MR. Understanding the role of the gut microbiome in brain development and its association with neurodevelopmental psychiatric disorders. Front Cell Dev Biol. 2022 Apr 14;10:880544. doi:10.3389/fcell.2022.880544.
- Pate T, Anthony DC, Radford-Smith DE. cFOS expression in the prefrontal cortex correlates with altered cerebral metabolism in developing germ-free mice. Front Mol Neurosci. 2023 Apr 20;16:1155620. doi:10.3389/fnmol.2023.1155620.
- Hoban AE, Stilling RM, Ryan FJ, Shanahan F, Dinan TG, Claesson MJ, Clarke G, Cryan JF. Regulation of prefrontal cortex myelination by the microbiota. Transl Psychiatry. 2016 Apr 5;6(4):e774–e774. doi:10.1038/tp.2016.42.
- Stilling RM, Ryan FJ, Hoban AE, Shanahan F, Clarke G, Claesson MJ, Dinan, T G., Cryan, J F. Microbes & neurodevelopment – absence of microbiota during early life increases activity-related transcriptional pathways in the amygdala. Brain, Behavior, And Immunity. 2015 Nov;50:209–220. doi:10.1016/j.bbi.2015.07.009.
- Glebov K, Löchner M, Jabs R, Lau T, Merkel O, Schloss P, Steinhäuser C, Walter J. Serotonin stimulates secretion of exosomes from microglia cells: serotonin stimulates microglial exosome release. Glia. 2015 Apr;63(4):626–634. doi:10.1002/glia.22772.
- Feng W, Wang Y, Liu ZQ, Zhang X, Han R, Miao YZ, Qin Z-H. Microglia activation contributes to quinolinic acid-induced neuronal excitotoxicity through TNF-α. Apoptosis. 2017 May;22(5):696–709. doi:10.1007/s10495-017-1363-5.
- Stoeckli ET. Morphogens and neural development. In: Binder M, Hirokawa N Windhorst U, editors. Encyclopedia of neuroscience [internet]. Berlin, Heidelberg: Springer Berlin Heidelberg; 2009. pp. 2397–2401. [accessed 2022 Jun 7]. doi:10.1007/978-3-540-29678-2_3562.
- Gao W, Salzwedel AP, Carlson AL, Xia K, Azcarate-Peril MA, Styner MA, Thompson AL, Geng X, Goldman BD, Gilmore JH, et al. Gut microbiome and brain functional connectivity in infants-a preliminary study focusing on the amygdala. Psychopharmacology. 2019 May;236(5):1641–1651. doi:10.1007/s00213-018-5161-8.
- Semple BD, Blomgren K, Gimlin K, Ferriero DM, Noble-Haeusslein LJ. Brain development in rodents and humans: identifying benchmarks of maturation and vulnerability to injury across species. Prog Neurobiol. 2013 Jul;106–107:1–16. doi:10.1016/j.pneurobio.2013.04.001.
- Aldridge K, Cole KK, Moffitt Gunn AJ, Peck D, White DA, Christ SE. The effects of early-treated phenylketonuria on volumetric measures of the cerebellum. Mol Genet Metab Rep. 2020 Dec;25:100647. doi:10.1016/j.ymgmr.2020.100647.
- Thanos PK, Michaelides M, Gispert JD, Pascau J, Soto-Montenegro ML, Desco M, Wang R, Wang G-J, Volkow ND. Differences in response to food stimuli in a rat model of obesity: in-vivo assessment of brain glucose metabolism. Int J Obes. 2008 Jul;32(7):1171–1179. doi:10.1038/ijo.2008.50.
- Tang W, Meng Z, Li N, Liu Y, Li L, Chen D, Yang Y. Roles of gut microbiota in the regulation of hippocampal plasticity, inflammation, and hippocampus-dependent behaviors. Front Cell Infect Microbiol. 2021 Jan 27;10:611014. doi:10.3389/fcimb.2020.611014.
- Dieleman N, Koek HL, Hendrikse J. Short-term mechanisms influencing volumetric brain dynamics. NeuroImage Clin. 2017;16:507–513. doi:10.1016/j.nicl.2017.09.002.
- Penna E, Pizzella A, Cimmino F, Trinchese G, Cavaliere G, Catapano A, Allocca I, Chun JT, Campanozzi A, Messina G, et al. Neurodevelopmental disorders: effect of high-fat diet on synaptic plasticity and mitochondrial functions. Brain Sci. 2020 Oct 31;10(11):805. doi:10.3390/brainsci10110805.
- Oury F, Pierani A. Transient perinatal metabolic shifts determine neuronal survival and functional circuit formation. Cell. 2023 Apr;186(9):1819–1821. doi:10.1016/j.cell.2023.03.027.
- Bäckhed F, Ding H, Wang T, Hooper LV, Koh GY, Nagy A, Semenkovich CF, Gordon JI. The gut microbiota as an environmental factor that regulates fat storage. Proc Natl Acad Sci USA. 2004 Nov 2;101(44):15718–15723. doi:10.1073/pnas.0407076101.
- Valeri F, Endres K. How biological sex of the host shapes its gut microbiota. Front Neuroendocrinol. 2021 Apr;61:100912. doi:10.1016/j.yfrne.2021.100912.
- Crider A, Pillai A. Estrogen Signaling as a Therapeutic Target in Neurodevelopmental Disorders. J Pharmacol Exp Ther. 2017 Jan;360(1):48–58. doi:10.1124/jpet.116.237412.
- Oguz I, Yaxley R, Budin F, Hoogstoel M, Lee J, Maltbie E, Liu W, Crews FT. Comparison of magnetic resonance imaging in live vs. Post mortem rat brains. Aoki I, editor. PLoS ONE. 2013 Aug 13;8(8):e71027. doi:10.1371/journal.pone.0071027.
- Hennig J, Nauerth A, Friedburg H. RARE imaging: A fast imaging method for clinical MR. Magn Reson Med. 1986 Dec;3(6):823–833. doi:10.1002/mrm.1910030602.
- Dorr AE, Lerch JP, Spring S, Kabani N, Henkelman RM. High resolution three-dimensional brain atlas using an average magnetic resonance image of 40 adult C57Bl/6J mice. NeuroImage. 2008 Aug;42(1):60–69. doi:10.1016/j.neuroimage.2008.03.037.