ABSTRACT
For many years, it has been hypothesized that pathological changes to the gut microbiome in critical illness is a driver of infections, organ dysfunction, and other adverse outcomes in the intensive care unit (ICU). The advent of contemporary microbiome methodologies and multi-omics tools have allowed researchers to test this hypothesis by dissecting host–microbe interactions in the gut to better define its contribution to critical illness pathogenesis. Observational studies of patients in ICUs have revealed that gut microbial communities are profoundly altered in critical illness, characterized by markedly reduced alpha diversity, loss of commensal taxa, and expansion of potential pathogens. These key features of ICU gut dysbiosis have been associated with adverse outcomes including life-threatening hospital-acquired (nosocomial) infections. Current research strives to define cellular and molecular mechanisms connecting gut dysbiosis with infections and other outcomes, and to identify opportunities for therapeutic modulation of host–microbe interactions. This review synthesizes evidence from studies of critically ill patients that have informed our understanding of intestinal dysbiosis in the ICU, mechanisms linking dysbiosis to infections and other adverse outcomes, as well as clinical trials of microbiota-modifying therapies. Additionally, we discuss novel avenues for precision microbial therapeutics to combat nosocomial infections and other life-threatening complications of critical illness.
1. Introduction
Critically ill patients suffer from life-threatening conditions, including sepsis, trauma, respiratory failure, and others that require life-support interventions in intensive care units (ICUs). Patients with critical illness often suffer from multiple-organ failure, extreme physiologic stress, and a high susceptibility to hospital-acquired (nosocomial) infections, which all culminate in a high risk of mortality,Citation1–3 as well as long-term physical, cognitive, and psychiatric sequelae in survivors.Citation4 Among the organ systems affected in critical illness, emerging evidence has revealed that the microbial communities of the intestinal tract experiences profound ecological and functional disturbances.Citation5 Importantly, like injury to other organ systems (cardiovascular, pulmonary, renal, etc.), dysfunction of the gut microbiota in critical illness is associated with major adverse clinical outcomes including death, prolonged life support, and life-threatening nosocomial infections.Citation5–8 This association between intestinal dysbiosis and adverse outcomes has led to the hypothesis that therapeutic correction of intestinal microbial communities may represent a new avenue for life-support therapy in the ICU.Citation9 A crucial next step in the development of microbiome-targeted interventions for critical illness is to build upon these observational associations, toward a causal and mechanistic understanding of how gut microbes contribute to pathogenesis, to identify rationalized and effective therapeutic targets.
Critically ill patients demonstrate severe microbiota dysbiosis across multiple body sites, with the gut being the most extensively studied to date. A recent systematic review and meta-analysis highlighted core features of early gut dysbiosis in critical illness that is characterized by depletion of anaerobes that are prominent commensals in the healthy gut including Bifidobacterium, Blautia, and Faecalibacterium, coupled with expansion and overabundance of putative pathogens including Enterococcaceae and Enterobacteriaceae.Citation6 This review will summarize the prominent ecological and functional changes of the gut microbiota in critical illness, and explore recent advances in our understanding of mechanisms linking dysbiosis to adverse clinical outcomes in the ICU including nosocomial infections, and how these mechanistic insights may be targeted therapeutically.
2. Gut microbiome dysbiosis in critical illness
The gut microbiome of ICU patients undergoes extreme compositional and functional changes compared to healthy individuals (). Hallmarks of this ICU dysbiosis include reduced alpha diversity, reduction of commensal taxa that are abundant in the healthy gut, and overgrowth of organisms with pathogenic potential (pathobionts).Citation6 For the purpose of this review, the term “dysbiosis” will broadly encompass altered microbial composition associated with disease state, which may include loss of colonization resistance, pathobiont expansion, or both, as well as other changes to the composition and function of the microbiota that contribute to disease pathogenesis. Taxa such as Enterococcaceae, Staphylococcus, and Enterobacteriaceae are found in the healthy gut at very low levels and display little to no pathogenicity under homeostasis.Citation10,Citation11 In contrast, sequencing analysis of fecal samples from ICU patients often demonstrates that 50% or more of sequence reads are assigned to individual bacterial or fungal species including Enterococcus faecium, Escherichia coli, Candida albicans, or other pathobiont taxa.Citation12 These pathobionts are increased in both relative abundance and total biomass, indicating that the gut microenvironment in critical illness favors their growth.Citation12,Citation13 Importantly, this prominent expansion of pathobionts in the gut of critically ill patients has been linked, both directly and indirectly, with adverse outcomes including infections and increased mortality, as discussed in more detail below.
Figure 1. Gut microbiota dysbiosis in critical illness. Hallmark features of ICU gut dysbiosis include reduction of community alpha diversity, associated with the loss of commensal anaerobes, and expansion of pathobionts. Created in BioRender.com.
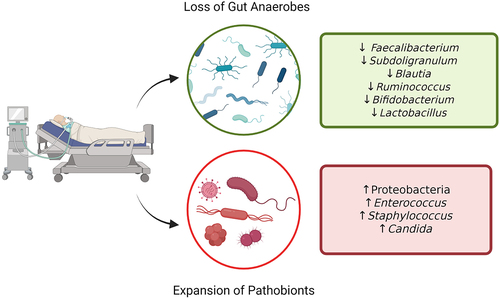
In addition to the expansion of potentially pathogenic organisms, another hallmark of ICU dysbiosis is the corresponding loss of commensal members of a healthy gut community. Studies of critically ill patients have consistently found reductions in the relative abundance of key obligate anaerobes, notably the Firmicutes and Bacteroidetes phyla.Citation5,Citation9,Citation14 The loss of anaerobic fermenters leads to a reduction in beneficial microbial metabolites such as short-chain fatty acids (SCFAs) like butyrate, which is known to maintain epithelial barrier integrity and contribute to immune homeostasis in the gut.Citation15 In critical illness, there is a notable loss of key butyrate-producing genera Faecalibacterium and Subdoligranulum.Citation5,Citation16 In addition, multiple studies have reported reduced Bifidobacterium abundance, a taxa that plays an important role in maintaining immune regulation and nutrient absorption, and when analyzed in combination with severity of illness the loss of this genera was associated with increased risk of death in a medical ICU.Citation7
Given the prominent changes in gut ecology that appear to characterize ICU dysbiosis and its associations with adverse outcomes, studies have begun to investigate the factors that drive these multi-taxa changes in the gut when people become critically ill. Most notably, alterations in gut microbial communities are attributed to the widespread use of broad-spectrum antibiotics in the ICU. Antibiotic administration in the ICU is commonplace, as demonstrated by the most recent iteration of a worldwide point-prevalence study of infections and antibiotic administration in 15,165 ICU patients, which found 79% of patients received antibiotics (either prophylactically or therapeutically).Citation1 Indeed, studies of healthy subjects have confirmed that systemic administration of broad-spectrum antibiotics drives marked changes in microbial ecology in the intestine, including depletion of commensal anaerobes akin to what is observed in ICU patients.Citation17 The use of multi-variable analyses in cohort studies of ICU patients has suggested that systemic antimicrobial exposure is an important variable driving microbiota dysbiosis.Citation18–21 In addition, comparisons between ICU patients who received antibiotics versus those who did not have also demonstrated an impact on microbiome composition. For example, a prospective observational study of septic and non-septic ICU patients found that antibiotic treatment (in septic patients) was associated with variation in microbiome composition, a reduction in SCFAs (purportedly due to the reduction in anaerobic fermenters), and increased fungal burden.Citation22 In addition to systemic antibiotic treatment, prophylactic anti-bacterial and anti-fungal administration for the goal of “selective digestive decontamination” (SDD) is also practiced in some jurisdictions, which will be discussed further below. However, it is important to note that gut microbiota dysbiosis is also observed in patients who have not received antimicrobial treatments (such as those admitted with neurological emergencies, trauma, or other noninfectious diagnoses), and that pathological dysbiosis has been reported in many patients at the time of presentation to the ICU (often within only hours of the inciting illness) prior to treatment with antibiotics.Citation5,Citation9,Citation23–26 Therefore, although aggressive antimicrobial exposure surely contributes to gut dysbiosis in the ICU, there are clearly additional drivers of the extreme shifts in microbial communities in this patient population.
In addition to antibiotics, critically ill patients are exposed to a variety of interventions that may impact gut bacteria and contribute to dysbiosis. For example, gastric acid suppression with medications like proton-pump inhibitors (PPI) are widely used for gastric ulcer prophylaxis in mechanically ventilated patients. Alteration of the upper GI pH with PPI therapy is known to induce microbiota alterations, and use of these medications has been associated with higher rates of dysbiosis-related complications including C. difficile infection and ventilator-associated pneumonia (VAP).Citation27,Citation28 In addition, profound alterations in nutritional intake that occur during critical illness likely also contribute to alterations in gut microbial ecology. Many patients in the ICU experience periods of time without nutrition, while others receive exclusively intravenous nutrition. Furthermore, enteral nutrition in the ICU is far from normal, as it is administered in the form of partially digested formula via a gastric tube. Coupled with the presence of invasive devices, and the ICU environment from which pathogens (and other microbes) can be acquired, these host-extrinsic factors impart significant selective pressure on microbes of the gut and contribute to ICU dysbiosis ().
Figure 2. Factors contributing to dysbiosis in critical illness. Multiple factors contribute to the significant shifts observed in microbial ecology of the gut during critical illness, including host intrinsic factors (circulatory shock, systemic acidosis, altered mucosal blood flow, inflammation, and others), as well as host extrinsic influences (antibiotics, nutritional alterations, medications, invasive devices, and others). Created in BioRender.com.
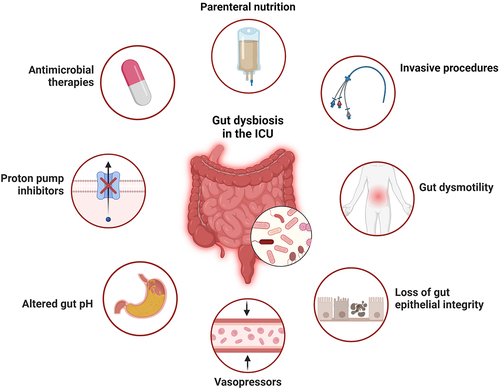
There are also a variety of host-intrinsic factors during critical illness that contribute to intestinal dysbiosis. Gastrointestinal dysmotility is present in up to 70% of ICU patients, and manifests as a spectrum from impaired motility (impaired gastrointestinal transit (IGT), ileus, acute colonic pseudo-obstruction), to diarrhea. Risk factors for developing GI dysmotility in the ICU include sepsis, mechanical ventilation, and the use of vasopressors, opioids, or anticholinergic medications.Citation29 Dysmotility and slowed transit time can result in small intestinal bacterial overgrowth (SIBO) syndrome,Citation30 which was found to affect up to 36.5% of patients in a small observational study of critically ill patients.Citation31 Overall, gastrointestinal dysmotility presents a challenge in the context of critical illness, as it can worsen dysbiosis, impact nutrient absorption, exacerbates bacterial translocation or overgrowth, and contributes to increased systemic inflammation.Citation32,Citation33 Beyond the gut, systemic physiological derangements in critical illness may also exert an important influence on bacterial communities. The presence of circulatory shock, and associated treatment with vasopressors, alters mucosal blood flow and impacts epithelial integrity.Citation34 Systemic inflammation associated with critical illness can also impact the gut microenvironment, and gut mucosal inflammation in animal models has been shown to generate a selective advantage for overgrowth of pathobionts such as Enterobacteriaceae.Citation35
Finally, aside from host-microbe interactions, it is likely that altered microbe–microbe interactions contribute to ICU dysbiosis. In particular, it is hypothesized that breakdown of colonization resistance (CR) mechanisms contributes to pathobiont expansion during critical illness.Citation36 Notably, the co-occurrence of anaerobic fermenter depletion with Enterobacteriaceae expansion is suggestive of disrupted homeostatic mechanisms of CR. Indeed, abundant preclinical evidence has unraveled important roles for anaerobic fermenters in the suppression of Enterobacteriaceae in the gut through production of SCFA, reduction of luminal pH, maintenance of anaerobiosis, and suppression of inflammation which all antagonize growth of Enterobacteriaceae.Citation37–39 Indeed, a recent study of critically ill patients found that treatment with anti-anaerobe antibiotics was associated with expansion of Enterobacteriaceae in gut, possibly due to the disruption of anaerobe-mediated CR mechanisms.Citation26 Other common ICU pathobionts such as Enterococcus spp. are influenced by disrupted CR during acute illness. For example, in individuals who received hematopoietic stem cell transplant (HSCT) for hematologic malignancies, antibiotic therapy is associated with intestinal overgrowth by vancomycin-resistant Enterococcus (VRE), a common cause of nosocomial infections.Citation40,Citation41 VRE overgrowth in this patient population was associated with reduced production of a VRE-suppressing antimicrobial peptide (lantibiotic) by the microbiota. Restoring the production of this lantibiotic in the gut of mice using a defined microbial consortium restored colonization resistance against VRE.Citation41,Citation42 Further studies are needed to determine the role of specific mechanisms of CR within the ICU microbiome of critically ill patients, but these intriguing observations suggest the possibility that therapeutic strategies aimed at reconstitution of beneficial anaerobes and restoration of colonization resistance may suppress pathobiont overgrowth and reduce adverse outcomes.
3. Consequences of gut dysbiosis in critical illness
Gut dysbiosis has been associated with adverse outcomes including increased mortality, duration of life support, duration of hospitalization, and severity of illness.Citation5,Citation7,Citation8,Citation18 There have been recent advances in our understanding of mechanisms driving these associations between microbiome dysbiosis and adverse clinical outcomes, including their impact on multi-organ failure, and perhaps most importantly, the role of gut dysbiosis in the development of hospital-acquired (nosocomial) infections. In this section, we will review the literature on intestinal dysbiosis and key adverse outcomes in the ICU including multi-organ dysfunction, and hospital-acquired infections.
3.1. Dysbiosis and hospital-acquired (nosocomial) infections in critical illness
Emerging evidence links gut dysbiosis with the development of deadly hospital-acquired (nosocomial) infections and adverse outcomes in critical illness (). Multiple factors contribute to the risk of infections in the ICU, including the breach of physical barriers (intravascular catheters, bladder catheters, endotracheal intubation) and impaired immune defenses, but recent studies have highlighted the gut microbiota as a putative source and driver of nosocomial infections. Observational studies have reported associations between alterations of gut ecology and nosocomial infections, including community metrics such as reduced alpha diversity, as well as specific taxa that are associated with a risk of secondary infections.Citation43 The appreciation of microbiota dysbiosis as a contributor to infections in the ICU has prompted researchers to begin translating these correlations into causal linkages, identifying pathogenic mechanisms, and seeking novel treatment targets for rationalized microbiota modification therapies. In this section, we will discuss emerging evidence around the mechanisms linking gut dysbiosis to infection in the ICU.
Figure 3. Mechanisms linking gut microbiota dysbiosis with hospital-acquired (nosocomial) infections in critical illness. The loss of commensal anaerobes contributes to breakdown of gut barrier integrity, as well as impaired colonization resistance against pathobiont taxa. Expansion of pathobionts (eg. Enterobacteriaceae), coupled with impaired epithelial barrier integrity and systemic immune dysfunction, enables the development of invasive infections by both gut pathobionts (through translocation and dissemination) as well as other pathogens due to impaired systemic immune defense. Created in BioRender.com.
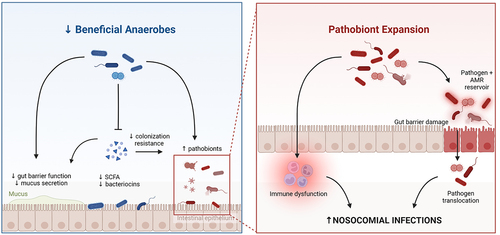
The gut as a pathogen reservoir in the ICU – As described above, a hallmark feature of the ICU gut microbiome is overrepresentation of potentially pathogenic organisms that are usually at low abundance or absent from the healthy gut. For decades, it has been appreciated that there is prominent overlap between the pathobionts that dominate the gut of ICU patients, and the microbiology of pathogens that are cultured from sites of infection – most commonly lung infections in patients on mechanical ventilation (VAP), and/or bloodstream infections.Citation1,Citation44–46 The application of culture-independent analysis methods in this patient population has confirmed that among the vast changes in microbial ecology in ICU patients, the presence and/or dominance of certain pathobiont taxa in the gut is associated with higher incidences of extra-intestinal infections by the same taxa, such as Enterobacteriaceae and Enterococcus spp. that commonly dominate acutely-ill patients.Citation25,Citation40,Citation47–52 These findings suggest that the dysbiotic microbiota of critical illness contributes to hospital-acquired infections, in part, through the emergence of a pathogen reservoir in the gut. In response to a breakdown of gut barrier integrity and dysregulation of immune defenses, this reservoir of pathogens may translocate out of the gut and disseminate to other body compartments to cause invasive infections. In addition to translocation and dissemination of whole pathogens, gut dysbiosis and permeability may contribute to systemic organ damage and dysfunction through translocation of microbial products and metabolites that exacerbate systemic inflammation and end-organ injury.
Translocation of pathogens out of the gut lumen and into the blood or lymphatic channels as a route of systemic infection has been well documented in animal models.Citation53–55 In humans, establishing gut translocation as the source of a systemic infections (such as bloodstream infections or pneumonia) is much more complicated and painstaking. Early evidence of this was observed in patients with hematologic malignancies undergoing hematopoietic stem cell transplantation (HSCT). Pre-transplant conditioning regimens for allogeneic HSCT often includes radiotherapy, chemotherapy, and antibiotics leading to suppressed host immunity, mucosal barrier injury, and intestinal dysbiosis, culminating in a high risk of bloodstream infections (BSI).Citation40,Citation41,Citation56 Analyses of the gut microbiota in combination with BSI pathogens in HSCT patients revealed that intestinal domination by Enterococcus (defined in these studies as at least 30% relative abundance) was associated with a 9-fold increased risk of vancomycin-resistant Enterococcus bacteremia.Citation40 Even stronger associations with BSI have been reported for intestinal domination by Gram-negative pathobionts including E. coli, Klebsiella spp, Enterobacter ssp., Stenotrophomonas spp., and Citrobacter spp.Citation49 These data suggested that BSIin HSCT patients may be preceded by intestinal overgrowth of the same organism, providing indirect evidence for translocation as a causal mechanism linking gut dysbiosis to nosocomial infections.Citation40 Similar relationships have been identified between gut colonization by Candida spp. and the risk of candidemia in HSCT patients.Citation57 In a study of patients with severe COVID-19 (>50% were admitted to ICU), individuals with bloodstream infections had an associated enrichment of the same genera of bacteria in their gut together with depletion of Faecalibacterium prauznitzii, suggesting that secondary bacterial bloodstream infections in COVID-19 may also be linked to pathobiont translocation from the gut.Citation50 To further define causal relationships between gut dysbiosis and bloodstream infections, multiple studies have conducted painstaking molecular detective work using metagenomic strain-tracking approaches to convincingly demonstrate that BSI pathogens originate from the gut microbiota (as opposed to other potential sources such as the ICU environment). In HSCT recipients with bloodstream infections, Tamburini and colleagues developed a bioinformatic StrainSifter tool to demonstrate that bloodstream infections caused by E. coli and Klebsiella pneumoniae could be traced back to the patient’s gut microbiota as the likely source.Citation48 Genomic analysis revealed that BSI and gut metagenome-assembled genomes (MAGs) of infecting pathogen strains within the same patient were more closely related than strains between different patients, indicating an individual-specific source of infection.Citation48 In addition, a recent study of critically ill neonates with bloodstream infections found that more than half patients harbored the identical (or nearly identical) pathogen strain in their gut microbiota prior to infection, with genomic similarity of <20 nucleotide differences.Citation51 These rigorous nucleotide-level comparisons of infecting pathogens and gut-derived MAGs provided the best evidence that some bloodstream infections in acutely ill and critically ill patients originate from translocation of pathobionts from the gut microbiota into the bloodstream.
Despite the methodological challenges of source tracking pathogens in the blood, this is more easily performed for bloodstream infections that tend to be monomicrobial and have a logical and direct anatomical route for translocation. In contrast, the more complex microbiology of other nosocomial infections, such as pneumonia in the lung, are more difficult to directly link to the gut as a source of pathogens. Indeed, the clinical microbiology of hospital- and ventilator-associated pneumonia reveals an important role for Gram-negative pathogens that are classically gut-associated.Citation1 However, the lung harbors its own bona fide microbiome which itself demonstrates severe dysbiosis during critical illness.Citation58 How the dysbiosis in the lung relates to dysbiosis of the gut is incompletely understood. Multiple studies have reported the accumulation of putatively gut-derived microbes in the lung microbiota in patients who develop nosocomial pneumonia,Citation58–60 as well as patients with acute respiratory distress syndrome (ARDS).Citation58,Citation61 In fact, this gut-lung axis has inspired large randomized controlled trials of gut microbiome modification with the goal of reducing the risk of lung infections (VAP, discussed further below).Citation62 Of note, there is also a substantial body of evidence linking airway dysbiosis in ventilated patients with micro- (or macro-) aspiration of oropharyngeal organisms, rather than microbes of the lower gastrointestinal tract.Citation63–65 A recent patient-level meta-analysis of microbiome sequencing data from airway secretions of mechanically ventilated patients revealed a large number of differentially abundant taxa in the airway microbiome of patients who developed nosocomial pneumonia versus those who did not, including a number of gut-associated genera including Enterococcus, Bacteroides, Faecalibacterium.Citation58 However, machine-learning based selection of factors that predict pneumonia identified reductions in core members of the respiratory microbiome (Streptococcus, Veillonella) as predictors of pneumonia, rather than enrichment of specific pathobionts.Citation58 Overall, further work on the complex microbiology of the lungs is needed to clarify the mechanisms underlying the gut–lung relationship in nosocomial pneumonia.
In addition to taxonomic shifts that transform the gut into a reservoir of potential pathogens, microbiota dysbiosis in critical illness may also lead to an accumulation of antibiotic-resistant organisms, and subsequent risk of infection by antimicrobial-resistant (AMR) pathobionts.Citation66,Citation67 A number of studies have found that infections with highly drug-resistant bacteria are associated with increased ICU mortality compared to infections by antibiotic-susceptible organisms.Citation68–70 In particular, infections by a common set of highly virulent and antibiotic-resistant pathogens, colloquially called ESKAPE (Enterococcus spp., S. aureus, K. pneumoniae, A. baumannii, P. aeruginosa, and E. coli), are a leading causes of nosocomial infections worldwide, in addition to being associated with higher economic burden, mortality, and morbidity.Citation71 Exposure to antimicrobials, especially those that are active against anaerobes, has been shown to facilitate expansion of antibiotic-resistant pathogens.Citation72,Citation73 Suppression of commensal anaerobes by antibiotics results in the loss of both microbe–microbe colonization resistance mechanisms,Citation74 as well as host–microbe interactions such as the down-regulation of intestinal C-type lectin RegIIIɣ that keep Gram-positive pathobionts like Enterococcus at bay.Citation75 However, the contribution of antimicrobial treatments toward AMR in critical illness may depend on the dose, route, and timing of broad-spectrum antibiotic use. For example, studies of patients receiving prophylactic enteral antimicrobials (selective digestive decontamination, SDD) found that AMR gene abundance was not different from patients who did not receiving SDD.Citation76 Furthermore, there may be factors beyond antibiotic selective pressure that contribute to the enrichment of AMR pathobionts in the ICU microbiome. For example, recent studies in germ-free and gnotobiotic mice have revealed that AMR bacteria may have a colonization advantage within the gut that is independent of antibiotic pressure. For example, colonization of germ-free mice with 2 strains of E. coli, one extensively drug resistant and another that is not, founds that AMR-E. coli out-competed and displaced non-resistant E. coli in the absence of any antibiotic administration.Citation77 Pan-genomic analysis of E. coli isolates found that that AMR carriage was associated with diversification of carbohydrate metabolism, providing a potential mechanism underlying gut colonization advantage for drug-resistant E. coli.Citation77 Overall, gut dysbiosis suffered by ICU patients not only creates a reservoir of pathogens, but also the accumulation of virulent and drug-resistant organisms that contribute to the high incidence, morbidity, and mortality of nosocomial infections in the ICU.
Pathological microbiome-immune interactions and infections in critical illness – While most infections in the ICU are from prototypically gut-associated microorganisms, there is not always a clear and direct relationship between gut overgrowth and infecting pathogens. Furthermore, ICU patients are susceptible to a variety of pathogens beyond gut pathobionts, and many infecting microbes in ICU patients do not cause invasive disease in healthy people (such as Coagulase-negative Staphylococcus, Enterococcus, Candida spp. and others).Citation1 Instead, this profound vulnerability to a variety of microbes is indicative of a state of compromised immune defenses. Indeed, widespread abnormalities of both innate and adaptive immunity have been observed in critically ill patients, and is known to contribute to their susceptibility to hospital acquired infections.Citation78–80 Given the important role of the gut microbiota in shaping immune development and function, together with studies showing pathogen-independent associations between dysbiosis and infections, this has led to the hypothesis that ICU dysbiosis may contribute to nosocomial infections in critical illness via its impact on immune defense mechanisms.
Animal models have elegantly demonstrated the importance of gut microbes in shaping immune development and function.Citation81 In addition, animal models have established the detrimental impact of gut dysbiosis on anti-pathogen immune defense mechanisms, both locally in the gut and systemically, which is coupled with impaired survival in mouse models of infection and sepsis.Citation82,Citation83 In humans, landmark observational studies have also identified important interactions between the gut microbiota and systemic immunity, including evidence that gut microbiota composition modulates cytokine responses by peripheral blood mononuclear cells,Citation84 and guides immune reconstitution after HSCT.Citation85 In critically ill patients, longitudinal analysis of microbiota-immune interactions identified that gut Enterobacteriaceae enrichment was associated with an increased risk of infection by a diversity of pathogens.Citation9 Furthermore, single-cell analysis of blood immune profiles in these patients found that Enterobacteriaceae enrichment was coupled with dysregulated mechanisms of innate immune defense, including immature hypofunctional neutrophil populations.Citation9 Such findings suggest that the microbiota and immune system are functionally coupled as a “metasystem”, and that dysbiosis of this inter-related metasystem in critical illness renders patients susceptible to diverse pathogens in multiple body compartments.Citation9
3.2. Dysbiosis and end-organ dysfunction in critical illness
In addition to infections, intestinal dysbiosis has been linked to adverse outcomes in the ICU through its putative contribution to intestinal and extra-intestinal organ dysfunction. Multi-organ dysfunction syndrome (MODS), defined as the failure in two or more organ systems requiring clinical intervention (i.e. mechanical ventilation for respiratory failure, circulatory support for cardiovascular failure, hemodialysis for renal failure, parenteral nutrition for gastrointestinal failure, etc.), is a common and deadly consequence of critical illness.Citation86 For many years, the gut has been hypothesized as a “motor” of multi-organ dysfunction. Initially, this hypothesis was based on evidence showing that increased intestinal permeability in ICU patients was associated with, and in fact preceded, multiple organ dysfunction syndrome (MODS).Citation87 Additional preclinical data has revealed that loss of gut epithelial barrier integrity led to endotoxin translocation, increased systemic inflammation, culminating in end-organ dysfunction.Citation83 In the contemporary era of microbiome science, it is now appreciated that there are multiple cellular and molecular axes of communication between microbes in the gut and extra-intestinal organs.Citation88 As such, our understanding of how gut microbes contribute to organ dysfunction in critical illness is becoming more refined. Below we will highlight recent advances in the role of gut dysbiosis in key organ dysfunction syndromes in critical illness, highlighting respiratory failure (acute respiratory distress syndrome, ARDS), and acute neurological dysfunction (delirium/encephalopathy) as some of the best studied organ systems.
Respiratory failure and ARDS – A substantial body of research links the gut microbiota to both acute and chronic lung diseases, described as a gut-lung axis.Citation89 In critical illness, much of the work on gut-lung axis has focused on the connection between intestinal microbes and acute respiratory distress syndrome (ARDS, a syndrome of acute lung injury and gas-exchange failure that can be precipitated by many common diseases in the ICU including sepsis, trauma, and others) as well as ventilator-associated pneumonia (VAP). The gut-lung axis has been recently reviewed in detail by others,Citation90 and therefore will only be briefly summarize here to highlight the role of the gut microbiota in lung dysfunction during critical illness. Seminal work from Dickson and colleagues using both mouse models and ICU patient samples demonstrated that the lung microbiota in ARDS becomes enriched with intestinal microbes (including pathobionts), and this contributes to the propagation of lung inflammation and damage.Citation61 Indeed, clinical microbiology of ventilator-associated pneumonia (VAP) in the ICU also reveals a high prevalence of gut-derived pathogens (such as E. coli, Klebsiella, Enterobacter, and others).Citation1 The exact route and mechanisms that allow gut organisms to become enriched in the lungs in critical illness are incompletely understood, but may include aspiration of upper GI contents, hematogenous seeding, and changes to the airway microenvironment (eg. inflammation, altered mucous) that favor colonization by pathobionts.Citation90 Importantly, the airways contain a homeostatic microbiota that is markedly disrupted in patients with ARDS and VAP. Montassier et al. recently characterized lung microbiome signatures that are characteristic of patients with ARDS in the ICU and found that the presence of Staphylococcus, Ralstonia, and Enterococcus were the best predictors of ARDS.Citation58 Therefore, the gut-lung axis in ARDS likely involves complex interplay between injury to the local lung microbiota, relocation of intestinal organisms to the airways, as well as a variety of host factors (immune alterations, airway inflammation, mucous changes) that remain to be fully elucidated.
Delirium – Delirium is a nonspecific state of acute neurological dysfunction manifesting as fluctuating confusion and impaired cognition. In the ICU, delirium is associated with increased mortality, duration of ventilation and hospitalization, and long-term cognitive impairment in survivors.Citation91–93 Although pathogenesis of delirium is poorly understood, recent studies have highlighted a potential role for gut dysbiosis and abnormal gut-brain axis. Significant differences in gut microbiota composition have been observed between older adults with delirium compared to participants who did not have delirium.Citation94 Furthermore, Enterobacteriaceae was associated with impaired consciousness and delirium severity.Citation94 While work looking into the gut-brain axis in critical illness is limited, an interesting clinical example that sheds light on the potential impact of gut dysbiosis and acute neurological dysfunction is hepatic encephalopathy (HE), which is acute delirium associated with decompensated cirrhosis. Dysbiosis characterized by an overabundance of urease-producing organisms in the gut of cirrhotic patients is thought to create excessive production of ammonia, which spills into the bloodstream causing a state of hyperammonemia.Citation95 Ammonia (and possibly other gut-microbe-derived primary or secondary metabolites) may cross the blood–brain barrier, resulting in neuron dysfunction and brain edema.Citation96 Interestingly, the treatment for HE is directed at the gut microbiota, through administration of the non-absorbable antibiotic rifaximin as well as nonabsorbable disaccharide lactulose. The example of hepatic encephalopathy provides a compelling link between gut dysbiosis and delirium that can be targeted therapeutically, raising the possibility that other forms of delirium in the ICU may be rooted in pathological gut-brain communication that may be amenable to microbiota-modulating therapies.
In addition to the organ dysfunction in the lung and the brain, gut dysbiosis has been connected with the severity of acute kidney injury,Citation97,Citation98 acute and chronic liver injury,Citation99,Citation100 coagulopathy (through impacts of dysbiosis on bacterial vitamin K production),Citation101 and hematologic abnormalities. However, further research is needed to define the mechanisms underlying the contribution of the microbiota to multiple-organ dysfunction, and guide rationalize therapeutic interventions.
4. Microbiome-targeted interventions in critical illness
Evidence linking ICU dysbiosis with adverse outcomes including nosocomial infections, together with the therapeutic malleability of the gut microbiota, has inspired great interest in microbiota modification as a therapeutic adjunct in critical illness. Multiple strategies have been studied in clinical trials, ranging from treatments aimed at depleting the pathogen reservoir with antimicrobials, to treatments aimed at reconstituting “normal” commensals with probiotics or fecal transplantation. Notably, as most evidence positions the microbiota as a proximal driver (or source) of adverse outcomes including nosocomial infections, microbiota interventions in the ICU to date have largely been prophylactic (that is, administered to patients early as prevention against infections or other adverse outcomes). Below, we provide a summary of evidence for microbiome-targeting therapies that have been studied in critically ill humans (an in-depth systematic review of the totality of clinical trial data is beyond the scope of this review), followed by a discussion of current research gaps and strategies to advance the translation of microbiome science in the ICU toward precision microbial therapeutics.
4.1. Targeting the intestinal pathogen reservoir with anti-microbials
Evidence implicating altered gut microbiota as a pathogen reservoir in critical illness has led to the hypothesis that “decontaminating” the gut with antimicrobials may reduce infections and mortality in the ICU. To test this hypothesis, studies were conducted testing cocktails of enterally administered, minimally absorbed (low bioavailability) antibacterial agents including polymyxin B, colistin, tobramycin, and/or neomycin, an antifungal agent (amphotericin B), along with a short course of IV cefotaxime. Due to minimal systemic absorption of the enteral agents, this approach was termed “selective digestive decontamination” (SDD), however there are likely effects beyond the GI tract given that systemic antibacterial drugs are also typically used. Microbiota analysis of ICU patients receiving SDD has shown that this treatment decreased pathobionts like E. coli and Candida burden in the gut, but simultaneously contributes to the loss of commensals including butyrate producers of Clostridium clusters IV, XIVa and F. prausnitzii .Citation102,Citation103 The clinical impact of SDD microbiota modulation has been assessed in multiple large randomized controlled trials, and recent systematic reviews and meta-analyses have concluded that SDD has a favorable impact on nosocomial infections and survival.Citation104,Citation105 A more recent, large, randomized controlled trial of SDD in Australian ICUs reported no difference the primary outcome of 90-day mortality between those receiving SDD compared to standard of care, however, there was a reduction in bacteremia as well as a reduction in culturable drug-resistant organisms.Citation106 However, concerns over the inconsistent outcomes of SDD trials as well as concerns over its possible contribution to rising rates of drug-resistant microbes has resulted in limited adoption of this practice outside of the Netherlands. Interestingly, despite theoretical concerns about SDD and the risk of AMR, trial data have found that SDD does not appear to increase rates of colonization or infection with AMR pathogens, and on the contrary may be associated with fewer AMR infections,Citation106–108 In addition to the gut, antimicrobial-mediated modulation of microbial colonizers in the oropharyngeal tract (selective oropharyngeal decontamination, SOD) has also been evaluated, and is reviewed elsewhere.Citation105 Head-to-head comparisons of SDD and SOD have indicated superiority of digestive tract decontamination with respect to hospital and ICU survival.Citation105 Nevertheless, the totality of evidence has not led to widespread adoption of SDD in most of the world.
The evidence indicating a reduction of nosocomial infections with SDD underscores the role of the gut microbiota in driving systemic infections in ICU patients. Interestingly, prophylactic antimicrobial therapy outside the gut has also been shown to reduce ICU-acquired infections.Citation109,Citation110 Most recently, Ehrmann et al. conducted a RCT of inhaled amikacin prophylaxis in mechanically ventilated ICU patients and found that compared to placebo, 3-days of inhaled amikacin reduced the incidence of nosocomial pneumonia.Citation110 The underlying mechanism by which inhaled amikacin reduced pneumonia (for example, suppression of specific pathobionts in the lung) and its influence on the lung microbiota were not assessed and raises interesting questions for future research. Overall, depletion of pathogen reservoirs in the microbiota through antibiotic treatments may improve clinical outcomes in the ICU, and the challenge for future research will be to refine the current relatively-broad-spectrum antimicrobial approach toward precision strategies that target pathobionts without eliminating putatively beneficial commensals or driving drug resistance.
4.2. Repopulating intestinal commensals with probiotics, prebiotics, and synbiotics
The consistent finding of reduced core microbiome commensals, in particular anaerobic fermenters, in the gut of ICU patients has inspired clinical interventions aiming to repopulate selected commensals. Mechanistically, restoration of core commensals could target both the gut pathogen reservoir by reestablishing colonization resistance against pathobionts, as well as potentially restoring homeostatic microbiota-immune interactions that aid in host defense against infections. Unfortunately, clinical application of probiotic, prebiotic, and synbiotics within randomized controlled trials has been markedly heterogeneous (diverse probiotic strains and prebiotic fibers of varying formulations, doses, and schedules), resulting in a lack of clarity around efficacy that limits their translation into routine clinical practice in the ICU.Citation111–114 Furthermore, the choice of probiotic organisms and prebiotic fibers used in this patient population has not been clearly aligned with biological mechanisms. Thus, not surprisingly, most of the largest trials have found these interventions do not improve clinical outcomes in adult patients. For example, the largest randomized controlled trial to date of probiotic therapy in critical illness investigated daily administration of enteral Lactobacillus rhamnosus GG versus placebo, and found no difference in rates of nosocomial pneumonia, other infections, nor survival.Citation62 The mechanistic rationale for Lactobacillus rhamnosus GG as the intervention of choice in this trial is unclear, and unfortunately its impact on the gut microbiota was not studied in trial participants. More detailed understanding of the molecular mechanisms mediating microbiota-immune communication, as well as mechanisms of impaired colonization resistance in the gut during critical illness may highlight particular species (or rationally designed consortia) that are more likely to yield clinical benefit due to keystone roles in these patients. Furthermore, there are unresolved safety concerns with administering live microbes to vulnerable, immunocompromised ICU patients, highlighted by recent reports of Lactobacillus bacteremia in 6 of 522 participants treated with Lactobacillus probiotics.Citation115
In addition to re-populating the gut with live probiotic bacteria, investigators have also begun to evaluate the impact of prebiotics to stimulate the growth and functions of putatively beneficial organisms. Observational data from a prospective cohort of ICU patients found that individuals who received soluble fiber were less acutely ill and less likely to receive broad-spectrum antibiotics, which was linked with higher numbers of SCFA producers including Blautia and F. prausnitzii.Citation116 A synbiotic therapy consisting of a consortia of 7 microbes from the Streptococcus, Lactobacillus, and Bifidobacterium genera together with fructooligosaccharide resulted in a significant reduction in serum endotoxins levels and neutrophil:lymphocyte ratio compared to placebo.Citation117 The impact of prebiotic and synbiotic therapies on clinical outcomes have been assessed in multiple, relatively small randomized controlled trials, and a systematic review and meta-analysis of 55 RCTs found that synbiotic therapy was likely superior to prebiotics alone with respect to the outcome of nosocomial infections.Citation112 However, there was no evidence indicating that they reduce hospital and ICU mortality.Citation112 While investigations have indicated that prebiotic and synbiotic treatments yield varying effects on outcomes, it is important to note the significant methodological variations across studies likely contributes to the lack of uniformity in results.
4.3. Fecal microbiota transplant
Studies using FMT in critically ill patients have been limited to case reports/series, and thus are heavily susceptible to bias. A limited number of cases of FMT in critically ill patients with multi-system disease have reported dramatic clinical improvements in recipients including reduction in fever and systemic inflammatory response within days, and an immediate improvement in diarrhea.Citation118–121 A case series of 18 ICU patients who underwent rescue FMT for intractable antibiotic-associated diarrhea reported that 13 out of 15 patients achieved improvement in diarrhea.Citation122 The biological impact of FMT on microbial communities of the gut (and other organs) in ICU patients has not been explored. It will also be important for future studies to address concerns around the safety of FMT in severely ill and immunocompromised patients, underscored by recent high-profile reports of infection by drug-resistant pathogens associated with FMT.Citation123 DeFilipp and colleagues identified 2 immunocompromised patients enrolled in FMT trials who developed bacteremia (one fatal) caused by extended-spectrum beta-lactamase producing E. coli, which was traced back to the same donor stool as the likely source.Citation123 Despite these reports, it is likely that the risk of donor-stool-derived infections in FMT are low, even in immunocompromised patients. For example, in a cohort of 22 HSCT recipients with acute graft-versus-host disease and high rates of infections, metagenomic analysis of host and donor microbiota as well as infecting pathogen did not find any evidence that infections originated from donor stool.Citation124 However, the impact of FMT on gut permeability and translocation of endogenous host-derived pathobionts in acute and critically ill patients remains to be defined. Collectively, FMT remains very uncommon in the ICU, and its potential role in this patient population is uncertain.
5. Key areas for future research
Despite the exciting and impactful research that has emerged in recent years, much remains to be learned about the ICU microbiome. Nearly all ICU microbiome studies to date have focused on bacterial communities, yet it is increasingly apparent that other kingdoms of microorganisms such as fungi and viruses play important roles in the ecology of the gut, as well as underappreciated contributions to microbiome–host interactions. Haak and colleagues used a combination of marker gene sequencing for bacteria and fungi (16S and ITS1 genes, respectively) as well as virus discovery cDNA-amplified fragment length polymorphism next-generation sequencing (VIDISCA-NGS) to describe the multi-kingdom composition of 33 critically ill patients.Citation22 They found that pathobiont bacteria including Staphylococcus, Enterococcus, Klebsiella, Escherichia and Enterobacter tend to co-occur with fungal members like Candida, Aspergillus and Debaryomyces, as well as bacteriophages that target these bacteria.Citation22 Abbas et al. discovered a new family of circular DNA viruses, Redondoviridae, that maybe enriched in the respiratory tract in ICU patients compared to healthy controls.Citation125 A recent study of patients with severe COVID-19 identified fungal dysbiosis in the GI tract characterized by Candida overgrowth, which was coupled to adverse reprogramming of myeloid progenitor cells and altered neutrophil responses that worsened lung inflammation.Citation126 Clearly, ICU dysbiosis extends beyond the bacterial microbiome, but much remains to be discovered about the functional contributions of non-bacterial microbes toward the pathogenesis of critical illness. A key challenge for future research will be to develop a deeper and more comprehensive understanding of the multi-kingdom ecology of ICU dysbiosis, the inter-kingdom dynamics, and their role in immune dysregulation, infections, and other outcomes in critical illness.
An additional challenge for the ICU microbiome research community will be to translate observations linking dysbiosis and adverse outcomes into rationalized and targeted therapeutic interventions. We propose that a crucial ingredient in the success of microbiota-targeted interventions in the ICU involves moving away from empirical and untargeted interventions (generic probiotics, broad-spectrum antibiotics, FMT) toward mechanism-guided approaches that target specific mediators of microbiota–host interactions. For this, further emphasis is needed on defining causal mechanisms mediating the relationships between dysbiosis and outcomes, as well as understanding the mechanistic impacts of microbiome-targeting interventions in the setting of clinical trials. This will likely require an integrative multi-omics approach when studying patient samples, as has been used recently to identify potentially important microbiota-immune dysregulation in nosocomial infections.Citation9 Furthermore, complementing human studies with reverse-translational models such as humanized gnotobiotic mice, wherein human microbiomes are engrafted in germ-free mice, has proven to be a powerful strategy to dissect cellular and molecular mechanisms of microbiome–host interactions in other diseases,Citation127–129 and can be very useful for pre-clinical testing of targeted microbial therapeutics prior to human clinical trials.Citation128 However, it is important to acknowledge that germ-free mice possess a markedly altered immune system compared to conventionally raised mice, which must be considered in the development of therapeutics targeting human diseases that have a large immune component, such as sepsis.
Lastly, the translation of microbiome science into effective targeted therapies in the ICU will require that we address a complex issue in personalized microbiome medicine – heterogeneity – of both microbiota as well as host factors. While population/cohort-level signatures of ICU dysbiosis have emerged, the quest for personalized microbiome therapies in the ICU will require applying our learnings to individual patients. Therefore, further scrutiny and validation of signatures of dysbiosis at the level of individual patients is essential, and will require improved microbiome-related diagnostics. Common sequencing methods such as amplicon-based or shotgun sequencing are unsuitable for rapid point-of-care diagnostics due to their time-consuming workflow, multiplexed design, and cost. Culture-based techniques may be employed for detecting microorganisms of interest, but are also time-consuming and only identify a limited number of microorganisms. Alternatively, next-generation long read sequencing methods are relatively cost-effective and may be a viable method for rapid microbiome diagnostics.Citation130
Beyond the microbiome, there is also substantial heterogeneity of clinical variables between individual patients that may impact a patient’s response to microbial therapeutics in the ICU.Citation16 Factors such as age, sex, comorbidities, and underlying diagnosis causing critical illness will need to be considered together with microbiota phenotype. For example, basic patient factors such as biological sex are known to interact with the microbiome in a manner that may influence the response to microbial therapeutics. Microbial communities in the gut have been found to differ between males and females, and these sex-based microbiome differences can influence sexual dimorphism of immune responses in disease.Citation131 Preclinical studies using mouse models of autoimmune diabetes have found that the incidence and early-onset of diabetes is greater in females compared to males, but this difference was abolished in GF mice.Citation132 Furthermore, male-to-female microbiota transfer protected females from early-onset diabetes, suggesting that the combination of biological sex and microbiome may have an important influence on disease pathogenesis as well as response to therapy.Citation132,Citation133 In critical illness, sex-biased clinical outcomes are common,Citation134 and gut microbiota differences have been demonstrated in ICU patients. Therefore, further work is needed to disentangle the relationships between important patient factors such as sex, microbiome dysbiosis, and mechanisms of disease pathogenesis in order to embrace the need for a personalized approach to precision microbial therapies in the heterogeneous realm of critical care.
List of abbreviations
ICU | = | intensive care unit |
AMR | = | antimicrobial resistance |
CR | = | colonization resistance |
SDD | = | selective digestive decontamination |
SDD | = | selective oropharyngeal decontamination |
SCFA | = | short chain fatty acids |
VAP | = | ventilator-associated pneumonia |
ARDS | = | adult respiratory distress syndrome |
BSI | = | bloodstream infection |
HSCT | = | hematopoietic stem cell transplant |
Authors contributions
All authors contributed to literature searches, article reviews, drafting, and editing the manuscript. In addition, BM provided overall supervision.
Acknowledgments
The authors would like to thank Jared Schlechte for interesting an inspiring discussions of topics in this manuscript.
Disclosure statement
No potential conflict of interest was reported by the author(s).
Data availability statement
This review article does not present any new data.
Additional information
Funding
References
- Vincent J-L, Sakr Y, Singer M, Martin-Loeches I, Machado FR, Marshall JC, Finfer S, Pelosi P, Brazzi L, Aditianingsih D. et al. Prevalence and outcomes of infection among patients in intensive care units in 2017. JAMA. 2020;323(15):1478–21. doi:10.1001/jama.2020.2717.
- Dautzenberg MJD, Wekesa AN, Gniadkowski M, Antoniadou A, Giamarellou H, Petrikkos GL, Skiada A, Brun-Buisson C, Bonten MJM, Derde LPG. et al. The association between colonization with carbapenemase-producing enterobacteriaceae and overall ICU mortality: an observational cohort study. Crit Care Med. 2015;43(6):1170. doi:10.1097/CCM.0000000000001028.
- Auld SC, Caridi-Scheible M, Blum JM, Robichaux C, Kraft C, Jacob JT, Jabaley CS, Carpenter D, Kaplow R, Hernandez-Romieu AC. et al. ICU and ventilator mortality among critically Ill adults with coronavirus disease 2019*. Crit Care Med. 2020;48(9):e799. doi:10.1097/CCM.0000000000004457.
- Hiser SL, Fatima A, Ali M, Needham DM. Post-intensive care syndrome (PICS): recent updates. J Intensive Care. 2023;11(1):23. doi:10.1186/s40560-023-00670-7.
- McDonald D, Ackermann G, Khailova L, Baird C, Heyland D, Kozar R, Lemieux M, Derenski K, King J, Vis-Kampen C. et al. Extreme dysbiosis of the microbiome in critical illness. mSphere. 2016;1(4):e00199–16. doi:10.1128/mSphere.00199-16.
- Evans T, Ali U, Anderton R, Raby E, Manning L, Litton E. Lower gut dysbiosis and mortality in acute critical illness: a systematic review and meta-analysis. Intensive Care Med Exp. 2023;11(1):6. doi:10.1186/s40635-022-00486-z.
- Wei R, Chen X, Hu L, He Z, Ouyang X, Liang S, Dai S, Sha W, Chen C. Dysbiosis of intestinal microbiota in critically ill patients and risk of in-hospital mortality. Am J Transl Res. 2021;13:1548–1557.
- Lamarche D, Johnstone J, Zytaruk N, Clarke F, Hand L, Loukov D, Szamosi JC, Rossi L, Schenck LP, Verschoor CP. et al. Microbial dysbiosis and mortality during mechanical ventilation: a prospective observational study. Respir Res. 2018;19(1):245. doi:10.1186/s12931-018-0950-5.
- Schlechte J, Zucoloto, AZ, Yu, IL, Doig, CJ, Dunbar, MJ, McCoy, KD, McDonald, B. Dysbiosis of a microbiota–immune metasystem in critical illness is associated with nosocomial infections. Nat Med. 2023;29(4):1–11. doi:10.1038/s41591-023-02243-5.
- Hollister EB, Gao C, Versalovic J. Compositional and functional features of the gastrointestinal microbiome and their effects on human health. Gastroenterology. 2014;146(6):1449–1458. doi:10.1053/j.gastro.2014.01.052.
- Human Microbiome Project Consortium. Structure, function and diversity of the healthy human microbiome. Nature. 2012;486:207–214.
- Ravi A, Halstead FD, Bamford A, Casey A, Thomson NM, van Schaik W, Snelson C, Goulden R, Foster-Nyarko E, Savva GM. et al. Loss of microbial diversity and pathogen domination of the gut microbiota in critically ill patients. Microb Genomics. 2019;5(9):e000293. doi:10.1099/mgen.0.000293.
- Zaborin A, Smith D, Garfield K, Quensen J, Shakhsheer B, Kade M, Tirrell M, Tiedje J, Gilbert JA, Zaborina O. et al. Membership and behavior of ultra-low-diversity pathogen communities present in the gut of humans during prolonged critical illness. mBio. 2014;5(5):e01361–14. doi:10.1128/mBio.01361-14.
- Liu Z, Li N, Fang H, Chen X, Guo Y, Gong S, Niu M, Zhou H, Jiang Y, Chang P. et al. Enteric dysbiosis is associated with sepsis in patients. Faseb J. 2019;33(11):12299–12310. doi:10.1096/fj.201900398RR.
- Smith PM, Howitt MR, Panikov N, Michaud M, Gallini CA, Bohlooly-Y M, Glickman JN, Garrett WS. The microbial metabolites, short-chain fatty acids, regulate colonic treg cell homeostasis. Science. 2013;341(6145):569–573. doi:10.1126/science.1241165.
- Lankelma JM, van Vught LA, Belzer C, Schultz MJ, van der Poll T, de Vos WM, Wiersinga WJ. Critically ill patients demonstrate large interpersonal variation in intestinal microbiota dysregulation: a pilot study. Intensive Care Med. 2017;43(1):59–68. doi:10.1007/s00134-016-4613-z.
- Lankelma JM, Cranendonk DR, Belzer C, de Vos AF, de Vos WM, van der Poll T, Wiersinga WJ. Antibiotic-induced gut microbiota disruption during human endotoxemia: a randomised controlled study. Gut. 2017;66(9):1623–1630. doi:10.1136/gutjnl-2016-312132.
- Ojima M, Shimizu K, Motooka D, Ishihara T, Nakamura S, Shintani A, Ogura H, Iida T, Yoshiya K, Shimazu T. et al. Gut dysbiosis associated with antibiotics and disease severity and its relation to mortality in critically Ill patients. Dig Dis Sci. 2022;67(6):2420–2432. doi:10.1007/s10620-021-07000-7.
- Szychowiak P, Villageois-Tran K, Patrier J, Timsit J-F, Ruppé É. The role of the microbiota in the management of intensive care patients. Ann Intensive Care. 2022;12(3). doi:10.1186/s13613-021-00976-5.
- Iapichino G, Callegari ML, Marzorati S, Cigada M, Corbella D, Ferrari S, Morelli L. Impact of antibiotics on the gut microbiota of critically ill patients. J Med Microbiol. 2008;57(8):1007–1014. doi:10.1099/jmm.0.47387-0.
- Agudelo-Ochoa GM, Valdés-Duque BE, Giraldo-Giraldo NA, Jaillier-Ramírez AM, Giraldo-Villa A, Acevedo-Castaño I, Yepes-Molina MA, Barbosa-Barbosa J, Benítez-Paéz A. Gut microbiota profiles in critically ill patients, potential biomarkers and risk variables for sepsis. Gut Microbes. 2020;12(1):1707610. doi:10.1080/19490976.2019.1707610.
- Haak BW, Argelaguet R, Kinsella CM, Kullberg RFJ, Lankelma JM, Deijs M, Klein M, Jebbink MF, Hugenholtz F, Kostidis S. et al. Integrative transkingdom analysis of the gut microbiome in antibiotic perturbation and critical illness. mSystems. 2021;6(2):e01148–20. doi:10.1128/mSystems.01148-20.
- Hayakawa M, Asahara T, Henzan N, Murakami H, Yamamoto H, Mukai N, Minami Y, Sugano M, Kubota N, Uegaki S. et al. Dramatic changes of the gut flora immediately after severe and sudden insults. Dig Dis Sci. 2011;56(8):2361–2365. doi:10.1007/s10620-011-1649-3.
- Livanos AE, Snider EJ, Whittier S, Chong DH, Wang TC, Abrams JA, Freedberg DE. Rapid gastrointestinal loss of clostridial clusters IV and XIVa in the ICU associates with an expansion of gut pathogens. PLOS One. 2018;13(8):e0200322. doi:10.1371/journal.pone.0200322.
- Freedberg DE, Zhou MJ, Cohen ME, Annavajhala MK, Khan S, Moscoso DI, Brooks C, Whittier S, Chong DH, Uhlemann A-C. et al. Pathogen colonization of the gastrointestinal microbiome at intensive care unit admission and risk for subsequent death or infection. Intensive Care Med. 2018;44(8):1203–1211. doi:10.1007/s00134-018-5268-8.
- Chanderraj R, Baker JM, Kay SG, Brown CA, Hinkle KJ, Fergle DJ, McDonald RA, Falkowski NR, Metcalf JD, Kaye KS. et al. In critically ill patients, anti-anaerobic antibiotics increase risk of adverse clinical outcomes. Eur Respir J. 2023;61(2):2200910. doi:10.1183/13993003.00910-2022.
- McDonald EG, Milligan J, Frenette C, Lee TC. Continuous proton pump inhibitor therapy and the associated risk of recurrent clostridium difficile infection. JAMA Internal Medicine. 2015;175(5):784–791. doi:10.1001/jamainternmed.2015.42.
- Lassalle M, Zureik M, Dray-Spira R. Proton pump inhibitor use and risk of serious infections in young children. JAMA Pediatr. 2023;177(10):1028–1038. doi:10.1001/jamapediatrics.2023.2900.
- Adike A, Quigley EMM. Gastrointestinal motility problems in critical care: a clinical perspective. J Dig Dis. 2014;15(7):335–344. doi:10.1111/1751-2980.12147.
- Dukowicz AC, Lacy BE, Levine GM. Small intestinal bacterial overgrowth. Gastroenterol Hepatol. 2007;3:112–122.
- Karakosta A, Bousvaros K, Margaritis A, Moschovi P, Mousafiri O, Fousekis F, Papathanakos G, Samara E, Tzimas P, Christodoulou D. et al. High prevalence of small intestinal bacterial overgrowth syndrome in ICU patients: an observational study. J Intensive Care Med. 2024;39(1):69–76. doi:10.1177/08850666231190284.
- Chang C, Lin H. Dysbiosis in gastrointestinal disorders. Best Pract Res Clin Gastroenterol. 2016;30(1):3–15. doi:10.1016/j.bpg.2016.02.001.
- Ladopoulos T, Giannaki M, Alexopoulou C, Proklou A, Pediaditis E, Kondili E. Gastrointestinal dysmotility in critically ill patients. Ann Gastroenterol. 2018;31:273–281. doi:10.20524/aog.2018.0250.
- Joffre J, Lloyd E, Wong E, Chung-Yeh C, Nguyen N, Xu F, Legrand M, Hellman J. Catecholaminergic vasopressors reduce toll-like receptor agonist-induced microvascular endothelial cell permeability but not cytokine production. Crit Care Med. 2021;49(3):e315–e326. doi:10.1097/CCM.0000000000004854.
- Zeng MY, Inohara N, Nuñez G. Mechanisms of inflammation-driven bacterial dysbiosis in the gut. Mucosal Immunol. 2017;10(1):18–26. doi:10.1038/mi.2016.75.
- Pamer EG. Resurrecting the intestinal microbiota to combat antibiotic-resistant pathogens. Science. 2016;352(6285):535–538. doi:10.1126/science.aad9382.
- Sorbara MT, Dubin K, Littmann ER, Moody TU, Fontana E, Seok R, Leiner IM, Taur Y, Peled JU, van den Brink MRM. et al. Inhibiting antibiotic-resistant enterobacteriaceae by microbiota-mediated intracellular acidification. J Exp Med. 2018;216(1):84–98. doi:10.1084/jem.20181639.
- Sorbara MT, Pamer EG. Interbacterial mechanisms of colonization resistance and the strategies pathogens use to overcome them. Mucosal Immunol. 2019;12(1):1–9. doi:10.1038/s41385-018-0053-0.
- Kamada N, Kim Y-G, Sham HP, Vallance BA, Puente JL, Martens EC, Núñez G. Regulated virulence controls the ability of a pathogen to compete with the gut microbiota. Science. 2012;336(6086):1325–1329. doi:10.1126/science.1222195.
- Taur Y, Xavier JB, Lipuma L, Ubeda C, Goldberg J, Gobourne A, Lee YJ, Dubin KA, Socci ND, Viale A. et al. Intestinal domination and the risk of bacteremia in patients undergoing allogeneic hematopoietic stem cell transplantation. Clin Infect Dis Off Publ Infect Dis Soc Am. 2012;55(7):905–914. doi:10.1093/cid/cis580.
- Kim SG, Becattini S, Moody TU, Shliaha PV, Littmann ER, Seok R, Gjonbalaj M, Eaton V, Fontana E, Amoretti L. et al. Microbiota-derived lantibiotic restores resistance against vancomycin-resistant enterococcus. Nature. 2019;572(7771):665–669. doi:10.1038/s41586-019-1501-z.
- Caballero S, Kim S, Carter RA, Leiner IM, Sušac B, Miller L, Kim GJ, Ling L, Pamer EG. Cooperating commensals restore colonization resistance to vancomycin-resistant enterococcus faecium. Cell Host Microbe. 2017;21(5):592–602.e4. doi:10.1016/j.chom.2017.04.002.
- Orieux A, Enaud R, Imbert S, Boyer P, Begot E, Camino A, Boyer A, Berger P, Gruson D, Delhaes L. et al. The gut microbiota composition is linked to subsequent occurrence of ventilator-associated pneumonia in critically ill patients. Microbiol Spectr. 2023;11(5):e0064123. doi:10.1128/spectrum.00641-23.
- Frencken JF, Wittekamp BHJ, Plantinga NL, Spitoni C, van de Groep K, Cremer OL, Bonten MJM. Associations between enteral colonization with gram-negative bacteria and intensive care unit–acquired infections and colonization of the respiratory tract. Clin Infect Dis Off Publ Infect Dis Soc Am. 2018;66(4):497–503. doi:10.1093/cid/cix824.
- Torres A, Dorca J, Zalacaín R, Bello S, El-Ebiary M, Molinos L, Arévalo M, Blanquer J, Celis R, Iriberri M. et al. Community-acquired pneumonia in chronic obstructive pulmonary disease: a Spanish multicenter study. Am J Respir Crit Care Med. 1996;154(5):1456–1461. doi:10.1164/ajrccm.154.5.8912764.
- Kallel H, Houcke S, Resiere D, Court T, Roncin C, Raad M, Nkontcho F, Demar M, Pujo J, Hommel D. et al. Prior carriage predicts intensive care unit infections caused by extended-spectrum beta-lactamase–producing enterobacteriaceae. Am J Trop Med Hyg. 2022;106(2):525–531. doi:10.4269/ajtmh.20-1436.
- Jung E, Byun S, Lee H, Moon SY, Lee H. Vancomycin-resistant enterococcus colonization in the intensive care unit: clinical outcomes and attributable costs of hospitalization. Am J Infect Control. 2014;42(10):1062–1066. doi:10.1016/j.ajic.2014.06.024.
- Tamburini FB, Andermann TM, Tkachenko E, Senchyna F, Banaei N, Bhatt AS. Precision identification of diverse bloodstream pathogens in the gut microbiome. Nat Med. 2018;24(12):1809–1814. doi:10.1038/s41591-018-0202-8.
- Stoma I, Littmann ER, Peled JU, Giralt S, van den Brink MRM, Pamer EG, Taur Y. Compositional flux within the intestinal microbiota and risk for bloodstream infection with gram-negative bacteria. Clin Infect Dis Off Publ Infect Dis Soc Am. 2021;73(11):e4627–e4635. doi:10.1093/cid/ciaa068.
- Bernard-Raichon L, Venzon M, Klein J, Axelrad JE, Zhang C, Sullivan AP, Hussey GA, Casanovas-Massana A, Noval MG, Valero-Jimenez AM. et al. Gut microbiome dysbiosis in antibiotic-treated COVID-19 patients is associated with microbial translocation and bacteremia. Nat Commun. 2022;13(1):5926. doi:10.1038/s41467-022-33395-6.
- Schwartz DJ, Shalon N, Wardenburg K, DeVeaux A, Wallace MA, Hall-Moore C, Ndao IM, Sullivan JE, Radmacher P, Escobedo M. et al. Gut pathogen colonization precedes bloodstream infection in the neonatal intensive care unit. Sci Transl Med. 2023;15(694):eadg5562. doi:10.1126/scitranslmed.adg5562.
- Andremont O, Armand-Lefevre L, Dupuis C, de Montmollin E, Ruckly S, Lucet J-C, Smonig R, Magalhaes E, Ruppé E, Mourvillier B. et al. Semi-quantitative cultures of throat and rectal swabs are efficient tests to predict ESBL-Enterobacterales ventilator-associated pneumonia in mechanically ventilated ESBL carriers. Intensive Care Med. 2020;46(6):1232–1242. doi:10.1007/s00134-020-06029-y.
- Yue C, Ma B, Zhao Y, Li Q, Li J. Lipopolysaccharide-induced bacterial translocation is intestine site-specific and associates with intestinal mucosal inflammation. Inflammation. 2012;35(6):1880–1888. doi:10.1007/s10753-012-9510-1.
- Gómez-Hurtado I, Santacruz A, Peiró G, Zapater P, Gutiérrez A, Pérez-Mateo M, Sanz Y, Francés R. Gut microbiota dysbiosis is associated with inflammation and bacterial translocation in mice with CCl4-induced fibrosis. PLoS One. 2011;6(7):e23037. doi:10.1371/journal.pone.0023037.
- Knoop KA, McDonald KG, Kulkarni DH, Newberry RD. Antibiotics promote inflammation through the translocation of native commensal colonic bacteria. Gut. 2016;65(7):1100–1109. doi:10.1136/gutjnl-2014-309059.
- Blijlevens NMA, Donnelly JP, de Pauw BE. Prospective evaluation of gut mucosal barrier injury following various myeloablative regimens for haematopoietic stem cell transplant. Bone Marrow Transplant. 2005;35(7):707–711. doi:10.1038/sj.bmt.1704863.
- Zhai B, Ola M, Rolling T, Tosini NL, Joshowitz S, Littmann ER, Amoretti LA, Fontana E, Wright RJ, Miranda E. et al. High-resolution mycobiota analysis reveals dynamic intestinal translocation preceding invasive candidiasis. Nat Med. 2020;26(1):59–64. doi:10.1038/s41591-019-0709-7.
- Montassier E, Kitsios GD, Radder JE, Le Bastard Q, Kelly BJ, Panzer A, Lynch SV, Calfee CS, Dickson RP, Roquilly A. et al. Robust airway microbiome signatures in acute respiratory failure and hospital-acquired pneumonia. Nat Med. 2023;29(11):2793–2804. doi:10.1038/s41591-023-02617-9.
- Kitsios GD, Fitch A, Manatakis DV, Rapport SF, Li K, Qin S, Huwe J, Zhang Y, Doi Y, Evankovich J. et al. Respiratory microbiome profiling for etiologic diagnosis of pneumonia in mechanically ventilated patients. Front Microbiol. 2018;9:1413. doi:10.3389/fmicb.2018.01413.
- Stanley D, Mason LJ, Mackin KE, Srikhanta YN, Lyras D, Prakash MD, Nurgali K, Venegas A, Hill MD, Moore RJ. et al. Translocation and dissemination of commensal bacteria in post-stroke infection. Nat Med. 2016;22(11):1277–1284. doi:10.1038/nm.4194.
- Dickson RP, Singer BH, Newstead MW, Falkowski NR, Erb-Downward JR, Standiford TJ, Huffnagle GB. Enrichment of the lung microbiome with gut bacteria in sepsis and the acute respiratory distress syndrome. Nat Microbiol. 2016;1(10):16113. doi:10.1038/nmicrobiol.2016.113.
- Johnstone J, Meade M, Lauzier F, Marshall J, Duan E, Dionne J, Arabi YM, Heels-Ansdell D, Thabane L, Lamarche D. et al. Effect of probiotics on incident ventilator-associated pneumonia in critically Ill patients: a randomized clinical trial. JAMA. 2021;326(11):1024–1033. doi:10.1001/jama.2021.13355.
- Sommerstein R, Merz TM, Berger S, Kraemer JG, Marschall J, Hilty M. Patterns in the longitudinal oropharyngeal microbiome evolution related to ventilator-associated pneumonia. Antimicrob Resist Infect Control. 2019;8(1):81. doi:10.1186/s13756-019-0530-6.
- Emonet S, Lazarevic V, Leemann Refondini C, Gaïa N, Leo S, Girard M, Nocquet Boyer V, Wozniak H, Després L, Renzi G. et al. Identification of respiratory microbiota markers in ventilator-associated pneumonia. Intensive Care Med. 2019;45(8):1082–1092. doi:10.1007/s00134-019-05660-8.
- de Castilhos J, Zamir E, Hippchen T, Rohrbach R, Schmidt S, Hengler S, Schumacher H, Neubauer M, Kunz S, Müller-Esch T. et al. Severe dysbiosis and specific haemophilus and neisseria signatures as hallmarks of the oropharyngeal microbiome in critically Ill coronavirus disease 2019 (COVID-19) patients. Clin Infect Dis. 2022;75(1):e1063–e1071. doi:10.1093/cid/ciab902.
- Forslund K, Sunagawa S, Kultima JR, Mende DR, Arumugam M, Typas A, Bork P. Country-specific antibiotic use practices impact the human gut resistome. Genome Res. 2013;23(7):1163–1169. doi:10.1101/gr.155465.113.
- Hu Y, Yang X, Qin J, Lu N, Cheng G, Wu N, Pan Y, Li J, Zhu L, Wang X. et al. Metagenome-wide analysis of antibiotic resistance genes in a large cohort of human gut microbiota. Nat Commun. 2013;4(1):2151. doi:10.1038/ncomms3151.
- Razazi K, Mekontso Dessap A, Carteaux G, Jansen C, Decousser J-W, de Prost N, Brun-Buisson C. Frequency, associated factors and outcome of multi-drug-resistant intensive care unit-acquired pneumonia among patients colonized with extended-spectrum β-lactamase-producing enterobacteriaceae. Ann Intensive Care. 2017;7(1):61. doi:10.1186/s13613-017-0283-4.
- Bottazzi A, Nattino G, Tascini C, Poole D, Viaggi B, Carrara G, Rossi C, Crespi D, Mondini M, Langer M. et al. Mortality attributable to different klebsiella susceptibility patterns and to the coverage of empirical antibiotic therapy: a cohort study on patients admitted to the ICU with infection. Intensive Care Med. 2018;44(10):1709–1719. doi:10.1007/s00134-018-5360-0.
- Lakbar I, Medam S, Ronflé R, Cassir N, Delamarre L, Hammad E, Lopez A, Lepape A, Machut A, Boucekine M. et al. Association between mortality and highly antimicrobial-resistant bacteria in intensive care unit-acquired pneumonia. Sci Rep. 2021;11(1):16497. doi:10.1038/s41598-021-95852-4.
- Zhen X, Lundborg CS, Sun X, Hu X, Dong H. Economic burden of antibiotic resistance in ESKAPE organisms: a systematic review. Antimicrob Resist Infect Control. 2019;8(1):137. doi:10.1186/s13756-019-0590-7.
- Ubeda C, Taur Y, Jenq RR, Equinda MJ, Son T, Samstein M, Viale A, Socci ND, van den Brink MRM, Kamboj M. et al. Vancomycin-resistant enterococcus domination of intestinal microbiota is enabled by antibiotic treatment in mice and precedes bloodstream invasion in humans. J Clin Invest. 2010;120(12):4332–4341. doi:10.1172/JCI43918.
- Donskey CJ, Chowdhry TK, Hecker MT, Hoyen CK, Hanrahan JA, Hujer AM, Hutton-Thomas RA, Whalen CC, Bonomo RA, Rice LB. et al. Effect of antibiotic therapy on the density of vancomycin-resistant enterococci in the stool of colonized patients. N Engl J Med. 2000;343(26):1925–1932. doi:10.1056/NEJM200012283432604.
- Kim S, Covington A, Pamer EG. The intestinal microbiota: antibiotics, colonization resistance, and enteric pathogens. Immunol Rev. 2017;279(1):90–105. doi:10.1111/imr.12563.
- Brandl K, Plitas G, Mihu CN, Ubeda C, Jia T, Fleisher M, Schnabl B, DeMatteo RP, Pamer EG. Vancomycin-resistant enterococci exploit antibiotic-induced innate immune deficits. Nature. 2008;455(7214):804–807. doi:10.1038/nature07250.
- Daneman N, Sarwar S, Fowler RA, Cuthbertson BH, SuDDICU Canadian Study Group. Effect of selective decontamination on antimicrobial resistance in intensive care units: a systematic review and meta-analysis. Lancet Infect Dis. 2013;13(4):328–341. doi:10.1016/S1473-3099(12)70322-5.
- Connor CH, Zucoloto AZ, Munnoch JT, Yu I-L, Corander J, Hoskisson PA, McDonald B, McNally A. Multidrug-resistant E. coli encoding high genetic diversity in carbohydrate metabolism genes displace commensal E. coli from the intestinal tract. PLOS Biol. 2023;21(10):e3002329. doi:10.1371/journal.pbio.3002329.
- Duggal NA, Snelson C, Shaheen U, Pearce V, Lord JM. Innate and adaptive immune dysregulation in critically ill ICU patients. Sci Rep. 2018;8(1):10186. doi:10.1038/s41598-018-28409-7.
- Pfortmueller CA, Meisel C, Fux M, Schefold JC. Assessment of immune organ dysfunction in critical illness: utility of innate immune response markers. Intensive Care Med Exp. 2017;5(1):49. doi:10.1186/s40635-017-0163-0.
- Tremblay J-A, Peron F, Kreitmann L, Textoris J, Brengel-Pesce K, Lukaszewicz A-C, Quemeneur L, Vedrine C, Tan LK, Venet F. et al. A stratification strategy to predict secondary infection in critical illness-induced immune dysfunction: the REALIST score. Ann Intensive Care. 2022;12(1):76. doi:10.1186/s13613-022-01051-3.
- Ansaldo E, Farley TK, Belkaid Y. Control of immunity by the microbiota. Annu Rev Immunol. 2021;39(1):449–479. doi:10.1146/annurev-immunol-093019-112348.
- Schlechte J, Skalosky I, Geuking MB, McDonald B. Long-distance relationships – regulation of systemic host defense against infections by the gut microbiota. Mucosal Immunol. 2022;15(5):809–818. doi:10.1038/s41385-022-00539-2.
- Miniet AA, Grunwell JR, Coopersmith CM. The microbiome and the immune system in critical illness. Curr Opin Crit Care. 2021;27(2):157–163. doi:10.1097/MCC.0000000000000800.
- Schirmer M, Smeekens SP, Vlamakis H, Jaeger M, Oosting M, Franzosa EA, Ter Horst R, Jansen T, Jacobs L, Bonder MJ. et al. Linking the human gut microbiome to inflammatory cytokine production capacity. Cell. 2016;167(4):1125–1136.e8. doi:10.1016/j.cell.2016.10.020.
- Schluter J, Peled JU, Taylor BP, Markey KA, Smith M, Taur Y, Niehus R, Staffas A, Dai A, Fontana E. et al. The gut microbiota is associated with immune cell dynamics in humans. Nature. 2020;588(7837):303–307. doi:10.1038/s41586-020-2971-8.
- Marshall JC. The multiple organ dysfunction syndrome. In: Holzheimer RG, Mannick JA, editors. Surgical treatment: evidence-based and problem-oriented. Zuckschwerdt; 2001.
- Doig CJ, Sutherland L, Dean Sandham J, Fick G, Verhoef M, Meddings J. Increased intestinal permeability is associated with the development of multiple organ dysfunction syndrome in critically Ill ICU patients. Am J Respir Crit Care Med. 1998;158(2):444–451. doi:10.1164/ajrccm.158.2.9710092.
- Schroeder BO, Bäckhed F. Signals from the gut microbiota to distant organs in physiology and disease. Nat Med. 2016;22(10):1079–1089. doi:10.1038/nm.4185.
- Dang AT, Marsland BJ. Microbes, metabolites, and the gut–lung axis. Mucosal Immunol. 2019;12(4):843–850. doi:10.1038/s41385-019-0160-6.
- Nath S, Kitsios GD, Bos LDJ. Gut–lung crosstalk during critical illness. Curr Opin Crit Care. 2023;29(2):130–137. doi:10.1097/MCC.0000000000001015.
- Kelly BJ, Imai I, Bittinger K, Laughlin A, Fuchs BD, Bushman FD, Collman RG. Composition and dynamics of the respiratory tract microbiome in intubated patients. Microbiome. 2016;4(7). doi:10.1186/s40168-016-0151-8.
- Ely EW, Shintani, A, Truman, B, Speroff, T, Gordon, SM, Harrell Jr, Frank E, Inouye, SK, Bernard, GR, Dittus, RS Delirium as a predictor of mortality in mechanically ventilated patients in the intensive care unit. JAMA. 2004;291(14):1753–1762. doi:10.1001/jama.291.14.1753.
- Ely E, Gautam S, Margolin R, Francis J, May L, Speroff T, Truman B, Dittus R, Bernard G, Inouye S. et al. The impact of delirium in the intensive care unit on hospital length of stay. Intensive Care Med. 2001;27(12):1892–1900. doi:10.1007/s00134-001-1132-2.
- Garcez FB, Garcia de Alencar JC, Fernandez SSM, Avelino-Silva VI, Sabino EC, Martins RCR, Franco LAM, Lima Ribeiro SM, Possolo de Souza H, Avelino-Silva TJ. et al. Association between gut microbiota and delirium in acutely Ill older adults. J Gerontol Ser A. 2023;78(8):1320–1327. doi:10.1093/gerona/glad074.
- Zhang Z, Zhai H, Geng J, Yu R, Ren H, Fan H, Shi P. Large-scale survey of gut microbiota associated with MHE via 16S rRNA-based pyrosequencing. Am J Gastroenterol. 2013;108(10):1601–1611. doi:10.1038/ajg.2013.221.
- Wijdicks EFM, Longo DL. Hepatic encephalopathy. N Engl J Med. 2016;375(17):1660–1670. doi:10.1056/NEJMra1600561.
- Yang J, Kim CJ, Go YS, Lee HY, Kim M-G, Oh SW, Cho WY, Im S-H, Jo SK. Intestinal microbiota control acute kidney injury severity by immune modulation. Kidney Int. 2020;98(4):932–946. doi:10.1016/j.kint.2020.04.048.
- Lei J, Xie Y, Sheng J, Song J. Intestinal microbiota dysbiosis in acute kidney injury: novel insights into mechanisms and promising therapeutic strategies. Ren Fail. 2022;44(1):571–580. doi:10.1080/0886022X.2022.2056054.
- Schneider KM, Elfers C, Ghallab A, Schneider CV, Galvez EJC, Mohs A, Gui W, Candels LS, Wirtz TH, Zuehlke S. et al. Intestinal dysbiosis amplifies acetaminophen-induced acute liver injury. Cell Mol Gastroenterol Hepatol. 2021;11(4):909–933. doi:10.1016/j.jcmgh.2020.11.002.
- Chen Y, Guo J, Qian G, Fang D, Shi D, Guo L, Li L. Gut dysbiosis in acute-on-chronic liver failure and its predictive value for mortality. J Gastroenterol Hepatol. 2015;30(9):1429–1437. doi:10.1111/jgh.12932.
- Violi F, Pignatelli P, Castellani V, Carnevale R, Cammisotto V. Gut dysbiosis, endotoxemia and clotting activation: a dangerous trio for portal vein thrombosis in cirrhosis. Blood Rev. 2023;57:100998. doi:10.1016/j.blre.2022.100998.
- Buelow E, Bello González TDJ, Fuentes S, de Steenhuijsen Piters WAA, Lahti L, Bayjanov JR, Majoor EAM, Braat JC, van Mourik MSM, Oostdijk EAN. et al. Comparative gut microbiota and resistome profiling of intensive care patients receiving selective digestive tract decontamination and healthy subjects. Microbiome. 2017;5(1):88. doi:10.1186/s40168-017-0309-z.
- van Doorn-Schepens MLM, Abis GSA, Oosterling SJ, van Egmond M, Poort L, Stockmann HBAC, Bonjer HJ, Savelkoul PHM, Budding AE. The effect of selective decontamination on the intestinal microbiota as measured with IS-pro: a taxonomic classification tool applicable for direct evaluation of intestinal microbiota in clinical routine. Eur J Clin Microbiol Infect Dis. 2022;41(11):1337–1345. doi:10.1007/s10096-022-04483-8.
- Hammond NE, Myburgh J, Seppelt I, Garside T, Vlok R, Mahendran S, Adigbli D, Finfer S, Gao Y, Goodman F. et al. Association between selective decontamination of the digestive tract and In-hospital mortality in intensive care unit patients receiving mechanical ventilation: a systematic review and meta-analysis. JAMA. 2022;328(19):1922–1934. doi:10.1001/jama.2022.19709.
- Plantinga NL, de Smet AMGA, Oostdijk EAN, de Jonge E, Camus C, Krueger WA, Bergmans D, Reitsma JB, Bonten MJM. Selective digestive and oropharyngeal decontamination in medical and surgical ICU patients: individual patient data meta-analysis. Clin Microbiol Infect Off Publ Eur Soc Clin Microbiol Infect Dis. 2018;24(5):505–513. doi:10.1016/j.cmi.2017.08.019.
- Boschert C, Broadfield E, Chimunda T, Fletcher J, Knott C, Porwal S, Smith J, Bhonagiri D, Leijten M, Narayan S. et al. Effect of selective decontamination of the digestive tract on hospital mortality in critically Ill patients receiving mechanical ventilation: a randomized clinical trial. JAMA. 2022;328(19):1911–1921. doi:10.1001/jama.2022.17927.
- Wittekamp BH, Plantinga NL, Cooper BS, Lopez-Contreras J, Coll P, Mancebo J, Wise MP, Morgan MPG, Depuydt P, Boelens J. et al. Decontamination strategies and bloodstream infections with antibiotic-resistant microorganisms in ventilated patients: a randomized clinical trial. JAMA. 2018;320(20):2087–2098. doi:10.1001/jama.2018.13765.
- Oostdijk EAN, Kesecioglu J, Schultz MJ, Visser CE, de Jonge E, van Essen EHR, Bernards AT, Purmer I, Brimicombe R, Bergmans D. et al. Notice of retraction and replacement: oostdijk et al. Effects of decontamination of the oropharynx and intestinal tract on antibiotic resistance in ICUs: a randomized clinical trial. JAMA. 2014;312(14):1429–1437. doi:10.1001/jama.2014.7247.
- François B, Cariou A, Clere-Jehl R, Dequin P-F, Renon-Carron F, Daix T, Guitton C, Deye N, Legriel S, Plantefève G. et al. Prevention of early ventilator-associated pneumonia after cardiac arrest. N Engl J Med. 2019;381(19):1831–1842. doi:10.1056/NEJMoa1812379.
- Ehrmann S, Barbier F, Demiselle J, Quenot J-P, Herbrecht J-E, Roux D, Lacherade J-C, Landais M, Seguin P, Schnell D. et al. Inhaled amikacin to prevent ventilator-associated pneumonia. N Engl J Med. 2023;389(22):2052–2062. doi:10.1056/NEJMoa2310307.
- Wang K, Zeng Q, Li KX, Wang Y, Wang L, Sun MW, Zeng J, Jiang H. Efficacy of probiotics or synbiotics for critically ill adult patients: a systematic review and meta-analysis of randomized controlled trials. Burns Trauma. 2022;10:tkac004. doi:10.1093/burnst/tkac004.
- Li C, Liu L, Gao Z, Zhang J, Chen H, Ma S, Liu A, Mo M, Wu C, Chen D. et al. Synbiotic therapy prevents nosocomial infection in critically Ill adult patients: a systematic review and network meta-analysis of randomized controlled trials based on a bayesian framework. Front Med. 2021;8:693188. doi:10.3389/fmed.2021.693188.
- Lou J, Cui S, Huang N, Jin G, Chen C, Fan Y, Zhang C, Li J. Efficacy of probiotics or synbiotics in critically ill patients: a systematic review and meta-analysis. Clin Nutr ESPEN. 2024;59:48–62. doi:10.1016/j.clnesp.2023.11.003.
- Manzanares W, Lemieux M, Langlois PL, Wischmeyer PE. Probiotic and synbiotic therapy in critical illness: a systematic review and meta-analysis. Crit Care. 2016;20(1):262. doi:10.1186/s13054-016-1434-y.
- Yelin I, Flett KB, Merakou C, Mehrotra P, Stam J, Snesrud E, Hinkle M, Lesho E, McGann P, McAdam AJ. et al. Genomic and epidemiological evidence of bacterial transmission from probiotic capsule to blood in ICU patients. Nat Med. 2019;25(11):1728–1732. doi:10.1038/s41591-019-0626-9.
- Fu Y, Moscoso DI, Porter J, Krishnareddy S, Abrams JA, Seres D, Chong DH, Freedberg DE. Relationship between dietary fiber intake and short-chain fatty acid–producing bacteria during critical illness: a prospective cohort study. JPEN J Parenter Enteral Nutr. 2020;44(3):463–471. doi:10.1002/jpen.1682.
- Seifi N, Sedaghat A, Nematy M, Khadem‐Rezaiyan M, Shirazinezhad R, Ranjbar G, Safarian M. Effects of synbiotic supplementation on the serum endotoxin level, inflammatory status, and clinical outcomes of adult patients with critical illness: a randomized controlled trial. Nutr Clin Pract. 2022;37(2):451–458. doi:10.1002/ncp.10758.
- Li Q, Wang C, Tang C, He Q, Zhao X, Li N, Li J. Successful treatment of severe sepsis and diarrhea after vagotomy utilizing fecal microbiota transplantation: a case report. Crit Care. 2015;19(1):37. doi:10.1186/s13054-015-0738-7.
- Wei Y, Yang J, Wang J, Yang Y, Huang J, Gong H, Cui H, Chen D. Successful treatment with fecal microbiota transplantation in patients with multiple organ dysfunction syndrome and diarrhea following severe sepsis. Crit Care. 2016;20(1):332. doi:10.1186/s13054-016-1491-2.
- Wurm P, Spindelboeck W, Krause R, Plank J, Fuchs G, Bashir M, Petritsch W, Halwachs B, Langner C, Högenauer C. et al. Antibiotic-associated apoptotic enterocolitis in the absence of a defined pathogen: the role of intestinal microbiota depletion*. Crit Care Med. 2017;45(6):e600–e606. doi:10.1097/CCM.0000000000002310.
- Li Q, Wang C, Tang C, He Q, Zhao X, Li N, Li J. Therapeutic modulation and reestablishment of the intestinal microbiota with fecal microbiota transplantation resolves sepsis and diarrhea in a patient. Off J Am Coll Gastroenterol ACG. 2014;109(11):1832. doi:10.1038/ajg.2014.299.
- Dai M, Liu Y, Chen W, Buch H, Shan Y, Chang L, Bai Y, Shen C, Zhang X, Huo Y. et al. Rescue fecal microbiota transplantation for antibiotic-associated diarrhea in critically ill patients. Crit Care. 2019;23(1):1–11. doi:10.1186/s13054-019-2604-5.
- DeFilipp Z, Bloom PP, Torres Soto M, Mansour MK, Sater MRA, Huntley MH, Turbett S, Chung RT, Chen Y-B, Hohmann EL. et al. Drug-resistant E. coli bacteremia transmitted by fecal microbiota transplant. N Engl J Med. 2019;381(21):2043–2050. doi:10.1056/NEJMoa1910437.
- Eshel A, Sharon I, Nagler A, Bomze D, Danylesko I, Fein JA, Geva M, Henig I, Shimoni A, Zuckerman T. et al. Origins of bloodstream infections following fecal microbiota transplantation: a strain-level analysis. Blood Adv. 2022;6(2):568–573. doi:10.1182/bloodadvances.2021005110.
- Abbas AA, Taylor LJ, Dothard MI, Leiby JS, Fitzgerald AS, Khatib LA, Collman RG, Bushman FD. Redondoviridae, a family of small, circular DNA viruses of the human oro-respiratory tract associated with periodontitis and critical illness. Cell Host Microbe. 2019;25(5):719–729.e4. doi:10.1016/j.chom.2019.04.001.
- Kusakabe T, Lin W-Y, Cheong J-G, Singh G, Ravishankar A, Yeung ST, Mesko M, DeCelie MB, Carriche G, Zhao Z. et al. Fungal microbiota sustains lasting immune activation of neutrophils and their progenitors in severe COVID-19. Nat Immunol. 2023;24(11):1879–1889. doi:10.1038/s41590-023-01637-4.
- Turnbaugh PJ, Ley RE, Mahowald MA, Magrini V, Mardis ER, Gordon JI. An obesity-associated gut microbiome with increased capacity for energy harvest. Nature. 2006;444(7122):1027–1031. doi:10.1038/nature05414.
- Gordon JI, Dewey KG, Mills DA, Medzhitov RM. The human gut microbiota and undernutrition. Sci Transl Med. 2012;4(137):ps13712–ps13712. doi:10.1126/scitranslmed.3004347.
- Wong SH, Zhao L, Zhang X, Nakatsu G, Han J, Xu W, Xiao X, Kwong TNY, Tsoi H, Wu WKK. et al. Gavage of fecal samples from patients with colorectal cancer promotes intestinal carcinogenesis in germ-free and conventional mice. Gastroenterology. 2017;153(6):1621–1633.e6. doi:10.1053/j.gastro.2017.08.022.
- Branton D, Deamer DW, Marziali A, Bayley H, Benner SA, Butler T, Di Ventra M, Garaj S, Hibbs A, Huang X. et al. The potential and challenges of nanopore sequencing. Nat Biotechnol. 2008;26(10):1146–1153. doi:10.1038/nbt.1495.
- Ma Z (Sam), Li W. How and why men and women differ in their microbiomes: medical ecology and network analyses of the microgenderome. Adv Sci. 2019;6(23):1902054. doi:10.1002/advs.201902054.
- Yurkovetskiy L, Burrows M, Khan A, Graham L, Volchkov P, Becker L, Antonopoulos D, Umesaki Y, Chervonsky A. Gender bias in autoimmunity is influenced by microbiota. Immunity. 2013;39(2):400–412. doi:10.1016/j.immuni.2013.08.013.
- Markle JGM, Frank DN, Mortin-Toth S, Robertson CE, Feazel LM, Rolle-Kampczyk U, von Bergen M, McCoy KD, Macpherson AJ, Danska JS. et al. Sex differences in the gut microbiome drive hormone-dependent regulation of autoimmunity. Science. 2013;339(6123):1084–1088. doi:10.1126/science.1233521.
- Merdji H, Long MT, Ostermann M, Herridge M, Myatra SN, De Rosa S, Metaxa V, Kotfis K, Robba C, De Jong A. et al. Sex and gender differences in intensive care medicine. Intensive Care Med. 2023;49(10):1155–1167. doi:10.1007/s00134-023-07194-6.