ABSTRACT
Amyotrophic lateral sclerosis (ALS) is a progressive neurodegenerative disease that leads to respiratory failure, and eventually death. However, there is a lack of effective treatments for ALS. Here we report the results of fecal microbiota transplantation (FMT) in two patients with late-onset classic ALS with a Japan ALS severity classification of grade 5 who required tracheostomy and mechanical ventilation. In both patients, significant improvements in respiratory function were observed following two rounds of FMT, leading to weaning off mechanical ventilation. Their muscle strength improved, allowing for assisted standing and mobility. Other notable treatment responses included improved swallowing function and reduced muscle fasciculations. Metagenomic and metabolomic analysis revealed an increase in beneficial Bacteroides species (Bacteroides stercoris, Bacteroides uniformis, Bacteroides vulgatus), and Faecalibacterium prausnitzii after FMT, as well as elevated levels of metabolites involved in arginine biosynthesis and decreased levels of metabolites involved in branched-chain amino acid biosynthesis. These findings offer a potential rescue therapy for ALS with respiratory failure and provide new insights into ALS in general.
Introduction
Amyotrophic lateral sclerosis (ALS) is a fatal, progressive neurodegenerative disease characterized by the loss of motor neurons in the brain and spinal cord. Patients with ALS have a median survival time of 2–3 yearsCitation1 and often die from respiratory muscle weakness and respiratory failure.Citation2 Although the exact etiology and pathogenesis of ALS remain unclear, it’s believed to be associated with genetic mutations, mitochondrial dysfunction, oxidative stress, neuroinflammation, protein homeostasis, and glutamate-mediated excitotoxicity.Citation3
Recent studies have indicated disparities in the gut microbiota between patients with ALS and healthy individuals. These differences include diminished abundance of butyrate-producing bacteria such as Roseburia intestinalis and Eubacterium rectale,Citation4 an increase in potentially harmful bacteria, such as Cyanobacteria,Citation5 and an imbalance in potentially protective microbes, such as Bacteroidales, Oscillibacter, Anaerostipes, and Lachnospiraceae.Citation6 Animal models further reinforce the potential role of the gut microbiota in ALS. For instance, Akkermansia muciniphila alleviates ALS symptoms, whereas Ruminococcus torques and Parabacteroides distasonis aggravate them.Citation7 Jun et al. has suggested that early microbiota dysbiosis may be involved in ALS pathology.Citation8
Riluzole and edaravone are the two approved drugs with modest efficacy for ALS treatment by the United States Food and Drug Administration.Citation9 In real-world studies, riluzole can extend survival by 6–19 months,Citation10 but there was no significant difference in limb or bulbar function.Citation11 Furthermore, edaravone can slow disease progression only in specific patients,Citation12,Citation13 and the long-term results were not notably better than those of riluzole alone.Citation14 Effective treatment is still lacking for patients who have undergone tracheostomy.
Fecal microbiota transplantation (FMT) has shown promise for treating various neurological diseases.Citation15,Citation16 However, clinical data on its efficacy in ALS are limited. To date, only one clinical trialCitation17 and a single-patient case study targeting slow limb symptom progression,Citation18 have been reported. There have been no reports on the effectiveness of FMT for ALS patients with respiratory failure.
Patients and methods
Clinical characteristics of patients
Case 1
Case 1 was a 71-year-old man with a 6-month history of poor appetite, 20 kg weight loss, and progressive limb weakness over 3 months. He had experienced breathing and swallowing difficulties and slurred speech over the last 2 months.
Six months before admission, the patient had taken antibiotics orally for 7 days due to a cold and fever and developed limb weakness after antibiotic withdrawal, but did not develop dysphagia or dyspnea. After that, no fever and any infection occurred, and antibiotics were not used. The patient’s condition gradually progressed.
Three months before admission, his limb weakness worsened and his body weight decreased by 20 kg. Two months before admission, the patient began to experience dyspnea, dysphagia, slurred speech, and coughing when drinking water. Owing to type II respiratory failure two weeks before admission, he underwent endotracheal intubation and mechanical ventilation.
Upon transfer to our hospital, the patient had no fever, with a body temperature of 36.8°C, leukocytes levels of 7.88 × 10^9/L, neutrophils percentage of 93.9%, lymphocytes percentage of 3.7%, C-reactive protein (CRP) levels of 1.092 mg/dL, and interleukin-6 (IL-6) levels of 2.7 pg/mL. Physical examination revealed upper limb muscle strength of grades 2–4 and lower limb muscle strength of grades 3–4. Muscle atrophy and abnormal reflexes were localized in the upper (bulbar, cervical, thoracic, and lumbar segments) and lower motor neurons (cervical, thoracic, and lumbar segments). Electromyography (EMG) demonstrated significant impairment in both the upper limbs and right motor axon, with slight sensory nerve amplitude reduction and anterior horn cell damage. According to the revised El Escorial criteriaCitation19 and Japan ALS severity classification,Citation12 the patient was diagnosed with late-onset classic ALS, Grade 5.
After admission, piperacillin sodium and tazobactam were injected intravenously for 11 d (June 22th to July 3rd), and antibiotics were discontinued three weeks before FMT.
Riluzole, edaravone, and butylphthalide treatment for 2 weeks showed no noticeable improvements. Before FMT, respiratory parameters were synchronized intermittent mandatory ventilation-volume control mode: FiO2 = 35%, tidal volume (TV) = 450 mL, positive end expiratory pressure (PEEP) = 5 cmH2O, respiratory rate (RR) = 15/min.
Case 2
Case 2 was a 76-year-old man with a 6-month history of progressive limb weakness and reduced appetite, who had lost 15 kg over the past 3 months. He had experienced breathing and swallowing difficulties and choking over in the last 2 months. He experienced no systemic infections or specific medications during the course of the disease.
The patient’s respiratory distress worsened, leading to admission. Upon admission, the patient’s body temperature was 36.7°C, leukocytes levels of 11.66 × 10^9/L, neutrophils percentage of 92.5%, lymphocytes percentage of 5.2%, CRP levels of 13.3 mg/dL, IL-6 levels of 148.4 pg/mL, while blood gas analysis indicated type II respiratory failure (PO2 53 mmHg, PCO2 72 mmHg). Chest computerized tomography imaging showed multiple mucus thrombi in both lungs with cord shadow. Considering respiratory failure with lung infection, the patient received endotracheal intubation, mechanical ventilation, and antibiotics. Physical examination revealed level 4 limb muscle strength, muscle atrophy, and abnormal reflexes in both the lower (bulbar, cervical, thoracic, and lumbar segments) and upper motor neurons (cervical and thoracic segments). EMG demonstrated extensive neurogenic damage. According to the revised El Escorial criteria and the Japan ALS severity classification, the patient was diagnosed with late-onset classic ALS, grade 5.
Intravenous cefoperazone sodium sulbactam sodium and cefuroxime sodium were administered for 28 d and 3 d, respectively. All antibiotics were discontinued 2 weeks before FMT treatment. Despite 3 weeks of treatment with edaravone and butylphthalide, his condition did not improve. Before FMT, the respiratory parameters were in the volume-controlled ventilation mode (FiO2 = 50%, TV = 450 mL, RR = 14/min, and PEEP = 4 cmH2O).
FMT procedure
Donor selection criteria were based on our previous protocol,Citation20 and both patients received FMT from the same qualified donor, a 28-year-old woman. On the day of treatment, the donor provided approximately 150–200 g of fresh feces, which were then transferred to the microbiology laboratory within 1 h. A portion of the donor fecal sample was aliquoted and stored at −80°C for long-term preservation. Following our fecal microbiota preparation protocol,Citation20 the feces were dissolved in approximately 500 ml of saline solution. The mixture was homogenized, agitated, and subjected to multistage filtration using a fecal pretreatment instrument, producing 300 ml of fecal suspension.
Both patients received two rounds of FMT. For Case 1, the interval between the two FMT treatments was approximately 4 weeks. Evaluations were conducted before FMT, 4 weeks after the first FMT, and 6 weeks after the second FMT. For Case 2, the interval between the two FMT treatments was approximately 5 weeks. Evaluations were conducted before FMT, 4 weeks after the first FMT, and 3 months after the second FMT.
Before FMT, patients underwent intestinal preparation with sodium phosphate oral solution. The FMT procedure was performed at the bedside after a routine colonoscopy. After the standard colonoscopic examination, an endoscopic spray tube was inserted through the biopsy channel of the endoscope with its tip positioned at the end of the ileum. Slow instillation of 300 ml of fecal suspension was administered using a syringe. The patients remained in a supine or right lateral decubitus position for 2 h after the procedure and resume normal eating and drinking 2 h later. The FMT procedure was identical for both patients
Outcome measure
The primary assessment criterion was the revised ALS functional rating scale (ALSFRS-R).Citation21 The secondary outcome measures were metagenomic and untargeted metabolomics changes. Assessment videos and figures were used as supplementary evaluation tools.
Sample collection
Fecal samples collected before the first FMT, after the first FMT, and after the second FMT were labeled F0, F1, and F2, respectively. For Case 1, F0 was collected 1 d before the first FMT, F1 4 weeks after the first FMT, and F2 6 weeks after the second FMT. For Case 2, F0 was collected 1 d before the first FMT, F1 5 weeks after the first FMT, and F2 3 months after the second FMT.
Fecal samples were collected from the donor on the day of treatment. For Case 1, donor fecal samples for the first FMT were labeled Donor1-F1, and those for the second treatment were labeled Donor1-F2. For Case 2, donor fecal samples for the first FMT were labeled Donor2-F1, and those for the second treatment were labeled Donor2-F2.
Fecal samples were transported to the laboratory within 2 hours of collection, immediately aliquoted, and stored at −80°C for long-term preservation.
Metagenomic sequencing
In both cases, DNA was extracted from fecal samples collected before and after FMT (F0, F1, F2) and from donor fecal samples (Donor1-F1, Donor1-F2, Donor2-F1, Donor2-F2). DNA libraries were prepared using the Illumina NEBNext® UltraTM DNA Library Prep Kit (NEB, USA, Catalog #: E7370L) following the manufacturer’s instruction. Library quality was assessed using the Agilent 5400 system (Agilent, USA), and quantification was performed using qPCR (1.5 nM). Metagenomic sequencing was conducted on an Illumina platform using a PE150 sequencer (Novogene Co., Ltd. Beijing, China).
Metagenomic sequencing data were first subjected to quality control using Fastp software V0.23.2. Subsequently, host genes were removed from the sequencing data using BWA V0.7.17 and Samtools software V1.9. The processed sequencing data were aligned to the Chocophlan database (Chocophlan, Version MPa_V30_Chocophlan_201901) for species-level annotation using Metaphlan V3.0.14. Visual analysis of the data was performed using R software V4.1.2. Alpha diversity was calculated using the Shannon index and beta diversity was calculated using the distance matrix obtained from weighted UniFrac analysis. Principal coordinate analysis (PCoA) was performed for dimension reduction and visualization. The top 20 microbial abundances at the phylum and species levels were selected for visualization.
We then used HMP Unified Metabolic Analysis Network 3 (HUMAnN3) to further generate function profiles.Citation22 The pathways are annotated using MetaCyc definitions.Citation23
Untargeted metabolomics analysis
Untargeted metabolomics analysis was performed on fecal samples collected before and after FMT (F0, F1, F2) and donor fecal samples (Donor1-F1, Donor1-F2, Donor2-F1, Donor2-F2) using liquid chromatography-mass spectrometry technology. Chromatographic separation was achieved using a Hypersil Gold column (100 × 2.1 mm, 1.9 μm) on a Vanquish UHPLC chromatograph. Mass spectrometric detection was carried out using the Q Exactive™ HF-X instrument (Thermo Fisher Scientific, Massachusetts, USA). Data files (.raw) were imported into the CD 3.1 search software for processing, which included peak extraction, peak area quantification, database matching, and standardization, resulting in the identification and relative quantification of metabolites. Untargeted metabolomics was performed by Novogene Co., Ltd. (Beijing, China).
The metabolites detected in both cation and anion modes were merged. The top 100 upregulated and downregulated metabolites, based on the differences before and after the second FMT (F2 vs. F0) were selected. Enrichment analysis of Kyoto Encyclopedia of Genes and Genomes (KEGG) pathways related to key metabolites was conducted using the enrichment analysis tool in MetaboAnalyst 5.0.
Results
Clinical improvement after FMT
Case 1
In Case 1, the patient underwent the first FMT on the 32nd day of admission. Before FMT, the patient was mechanically ventilated (synchronized intermittent mandatory ventilation-volume control mode, FiO2 = 35%, TV = 450 mL, PEEP = 5 cmH2O, RR = 15/min) and fed enterally. The patient was unable to hold objects, sit, or stand independently (Supplemental Materials Video 1). Five days after the first FMT, he began weaning off ventilation for a maximum weaning period of 6 h. On the 10th day, he commenced noninvasive ventilation (NIV) with an oxygen concentration of 3–5 L/min, and shifted from nasogastric tube feeding to oral intake. After 4 weeks, he no longer required supplemental oxygen and could eat orally without choking; however, his limb muscle strength remains unchanged (Supplemental Materials Video 2). Subsequently, the patient underwent a second FMT and was discharged (Supplemental Materials Figure S1a).
Six weeks after the second FMT, upon readmission, he utilized NIV, could tolerate spontaneous breathing for 1 h per day, improved oral intake with a 50% increase from the first admission, and an increased thigh circumference by 5–9 cm. His muscle strength also showed a slight improvement, allowing him to stand with assistance (Supplemental Materials Video 3). His ALSFRS-R scores before treatment, and after the first and second FMT, were 15, 18, and 20, respectively ().
Table 1. Scores of the ALS functional rating scale before and after FMT.
Case 2
In Case 2, the patient underwent the first FMT on the 47th day. Ten days after the first FMT, improvements in ventilation were evident: his FiO2 was reduced to 40% and PEEP to 3 cmH2O. Two weeks after FMT, the patient was weaned off ventilation three times a day, each period lasting 20 min. Four weeks after FMT, the weaning time was extended to 18 h daily. The gastrostomy tube was replaced with a nasogastric tube, and the patient began oral intake of liquid food (approximately 50–100 mL). The patient could then sit independently (Supplemental materials Figure S1). The second FMT was administered 5 weeks after the first FMT. Two weeks later, the patient was transitioned to NIV without supplemental oxygen, and discharged (Supplemental Materials Figure S1b).
Three months after the second FMT, during a readmission, he used NIV for approximately 12 h daily, walked independently for short durations, and wrote independently using a pen (Supplemental Materials Video 4). He could eat approximately 150 grams of food orally per meal without choking, resulting in a weight gain of 5 kg. His ALSFRS-R scores before and after the first and second FMT, were 15, 16, and 23, respectively (). Unfortunately, we do not have records before FMT. However, an assessment figure after the first FMT and a video taken after the second FMT are available in the Supplemental Materials.
Metagenomic changes during FMT
Fecal samples were collected from both patients before and after FMT to evaluate microbiome diversity, composition, and metabolomics using metagenomic sequencing and untargeted metabolomics.
In Case 1, before FMT, there were significant differences in both alpha and beta diversity of the gut microbiota compared to that of the donor (). The patient’s microbiota was dominated by Firmicutes and Actinobacteria (Supplemental Materials Figure S2a). After the second FMT, alpha diversity increased, narrowing the gap between the microbiota profiles of the patient and donor. Beta diversity tended to align more closely with the donor’s (). A noticeable shift occurred at the phylum level, resulting in an elevated Bacteroidetes/Firmicutes (B/F) ratio (F0 vs. F2 = 0.15 vs. 1.64). At the species level, Enterococcus faecalis, Collinsella aerofaciens, Bacteroides stercoris, Klebsiella pneumoniae, and Bacteroides uniformis were the top five abundant species before FMT (). After the first FMT, the top five species were Bacteroides fragilis, K. pneumoniae, Bifidobacterium dentium, Ruminococcus gnavus, and Escherichia coli. After the second FMT, these bacteria had a high abundance, including B. stercoris, R. torques, Bacteroides vulgatus, Faecalibacterium prausnitzii, B. uniformis, and Bifidobacterium pseudocatenulatum, five of which were within the top 10 most abundance in the donor ().
Figure 1. Microbiome analysis of Case 1 ALS patient and donor intestinal bacteria by metagenomics sequencing.
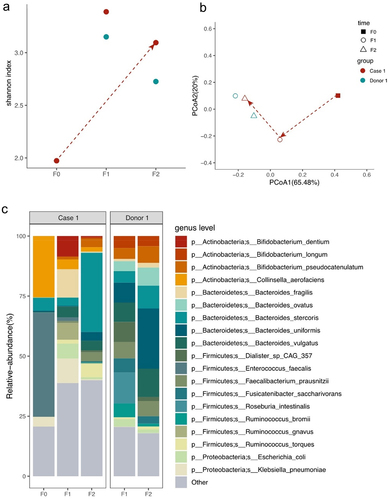
In Case 2, before FMT, there were significant differences in alpha and beta diversity compared to those of the donor (). Firmicutes and Actinobacteria were the predominant phyla (Supplemental Materials Figure S2b). After the second FMT, there was an increase in alpha diversity, with beta diversity aligning with that of the donor. The B/F ratio increased notably from 0 to 3.65. At the species level, the most prevalent species were Enterococcus faecium, E. faecalis, Clostridium clostridioforme, Clostridium innocuum, Klebsiella michiganensis and Flavonifractor plautii (). After the first FMT, the Bifidobacterium genus (B. pseudocatenulatum, B. adolescentis, and B. longum) became predominant. After the second FMT, the top 10 abundant species were B. stercoris, Alistipes finegoldii, Parabacteroides merdae, Bacteroides intestinalis, B. uniformis, B. vulgatus, F. plautii, B. longum, F. prausnitzii, and Bacteroides thetaiotaomicron, four of which were in the top five most abundant in the donor.
Figure 2. Microbiome analysis of Case 2 ALS patient and donor intestinal bacteria by metagenomics sequencing.
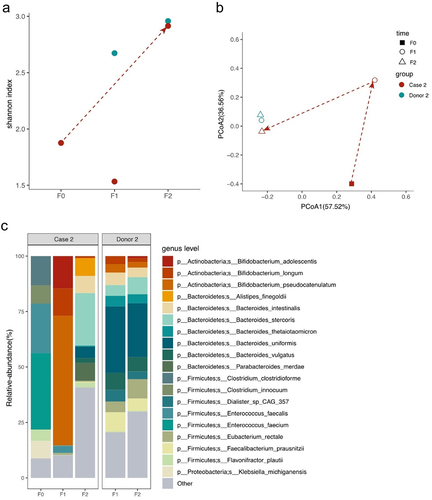
Both patients showed an increase of Bacteroides genus and F. prausnitzii after two rounds of FMT.
Different microbial functional profiles during FMT
As microbial composition differed before and after FMT, we used HUMAnN3 software and MetaCyc database to obtain functional information about the microorganisms present. A total of 472 metabolic pathways were identified in all the samples. After removing the low abundance pathways, we analyzed the pathways with increased abundance.
In Case 1, pathway analysis revealed that F2 was enriched in pathways involved in the metabolism of amino acids (VALSYN-PWY, ARGININE-SYN4-PWY, PWY-5154, HISTSYN-PWY, HISDEG-PWY, PWY-5030, TRPSYN-PWY, and COMPLETE-ARO-PWY), coenzyme A (PANTO-PWY and PANTOSYN-PWY), vitamin (RIBOSYN2-PWY, PYRIDOXSYN-PWY, PWY0–845, and THISYN-PWY) and glycolysis (PWY-1042).
In Case 2, compared with the baseline, pathways were enriched that are involved in the metabolism of amino acids (ASPASN-PWY, PWY-6163, PWY-7977, THRESYN-PWY, COMPLETE-ARO-PWY, HISDEG-PWY, PWY-5030, and ARG+POLYAMINE-SYN), coenzyme A (PANTOSYN-PWY, PANTO-PWY, and PWY-7851), vitamin (PYRIDOXSYN-PWY and THISYN-PWY) and glycolysis (PWY-1042, GLYCOLYSIS, and PWY-5484) after two rounds of FMT.
Both patients had enriched pathways involved in the metabolism of L-arginine and L-Histidine, coenzyme A biosynthesis, and glycolysis. Among these, the Bacteroides genus (B. stercoris, B. uniformis, and B. vulgatus) contributes the most to the coenzyme A biosynthesis superpathway (PANTOSYN-PWY) (Supplementary Figure S5a). B. vulgatus and F. prausnitzii are involved in glycolysis (PWY-1042) (Supplementary Figure S5b). The Bacteroides genus were the greatest taxonomic contributor in amino acid metabolism, especially L-Histidine degeneration (HISDEG-PWY and PWY-5030), as well as L-aspartate and L-asparagine biosynthesis (ASPASN-PWY).
Metabolomic changes during FMT
In Case 1, before FMT, metabolomics analysis highlighted an enrichment of certain metabolites associated with pathways related to arginine and proline metabolism (D-proline, 4-Hydroxyproline, L-Proline, and Ornithine) and aminoacyl-tRNA-biosynthesis (ketoleucine and L-Valine) (Supplemental materials Figure S4a,c). After the second FMT, the metabolomic profiles showed enriched metabolites associated with the arginine biosynthesis pathway (L-glutamic acid, L-aspartic acid, and N-acetylglutamic acid) and histidine biosynthesis (L-glutamic acid, histamine, methylimidazoleacetic acid, and L-aspartic acid) (Supplemental materials Figure S4b,d).
In Case 2, before FMT, metabolomics analysis showed an enrichment of metabolites associated with pathways related to valine, leucine and isoleucine biosynthesis (L-threonine, alpha-ketoisovaleric acid, and L-valine) (Supplemental materials Figure S5a,c). After the second FMT, there was enrichment of metabolites associated with arginine biosynthesis (L-glutamic acid, L-arginine, ornithine, and N-acetylglutamic acid), as well as purine metabolism pathway (adenosine, inosine, guanine, allantoic acid, and adenine) (Supplemental materials Figure S5b,d).
Discussion
ALS is a progressive and incurable disease that commonly causes death due to respiratory complications, including pneumonia and progressive respiratory failure.Citation24 Reports on respiratory reversal and improvement are scarce, particularly in patients who have undergone tracheostomy and mechanical ventilation. Plowman et al.Citation25 showed that 12 weeks of respiratory strength training led to a slow decline in the ALSFRS-R bulbar subscale in patients with early-stage ALS, but did not significant affect the timing of NIV referral compared with controls. Although plateaus and reversals are common during the natural course of ALS,Citation26 this phenomenon is not found in patients with respiratory failure.Citation27–30 There are no reports on the reversal of respiratory function in patients with severe ALS receiving mechanical ventilation.Citation31 To the best of our knowledge, this is the first study to report that FMT significantly improved severe ALS in patients requiring mechanical ventilation. Lo Coco et al.Citation32 reported that in ALS patients with acute respiratory failure treated with tracheostomy, the total ALSFRS score can predict the length of hospital stay and long-term survival after invasive mechanical ventilation. The use of NIV during weaning from mechanical ventilation decreases hospital mortality, the ventilator associated pneumonia incidence, and the length of ICU stay.Citation33 Our results showed significant improvement in respiratory function after two FMT procedures. Patients were able to switch from a mechanical ventilator to a noninvasive ventilator, with an increase in total ALSFRS-R. This means that FMT may be beneficial for improving patient survival rate.
Although various studies have found gut dysbiosis in patients with ALS, the mechanism underlying this link remains unclear. Possible mechanisms include altering the intestinal mucosal barrier, nutrient metabolism, immune and inflammatory responses, and directly affecting the enteric nervous system or brain function through toxins or neurotransmitters produced by the gut microbiota.Citation34
To investigate the mechanisms underlying FMT efficacy in ALS, we analyzed the metagenomics and metabolomics of fecal samples from both ALS patients undergoing FMT and their donor. Metagenomic sequencing highlighted the distinct alpha and beta diversity variations between patients with ALS and their donor. Specifically, the Firmicutes phylum was predominant in patients with ALS, reflected by an elevated F/B ratio. Kim et al. found that the F/B ratio gradually increased as the disease progressed in spinal-onset ALS patients.Citation35 Accordingly, we observed improvements in the symptoms of in patients with ALS after FMT, and a gradual decrease in the F/B ratio. We found that the abundance of beneficial bacteria (B. stercoris, B. uniformis, B. vulgatus and F. prausnitzii) was low in patients with ALS and enriched in donor. FMT can modulate these disorders, and the abundance of these beneficial species significantly increased after the two FMT procedures, especially Bacteroides species. Similarly, Zhai et al. reported that the relative abundance of beneficial micro-organisms (Faecalibacterium and Bacteroides) significantly decreased in abundance in patients with ALS.Citation36
We then analyzed the metabolic pathways of the bacteria enriched after FMT and observed that the enriched pathways were predominantly linked with amino acid, vitamin, and coenzyme A. Among these, both patients showed enrichment of pathways involved in coenzyme A biosynthesis, glycolysis, L-aspartate and L-asparagine, and L-Histidine metabolism. Bacteroides (B. stercoris, B. uniformis, B. vulgatus) contributed the most to the coenzyme A biosynthesis superpathway. In contrast, B. vulgatus and F. prausnitzii are involved in glycolysis.
Glycolysis and coenzyme A biosynthesis are essential pathways for energy metabolism. Defects in energy metabolism are potential pathogenic mechanisms in ALS.Citation37 A high sugar diet improves the locomotor and lifespan defects caused by TDP-43 proteinopathy in motor neurons or glia,Citation38 suggesting that glycolysis upregulation could be neuroprotective in ALS. Thus, glycolysis upregulation in diseased motor neurons in ALS could thus be a compensatory survival mechanism, and enhanced glucose uptake and metabolism appears to be beneficial in ALS.Citation39,Citation40 Bacteroides is the most common taxonomic contributor in amino acid metabolism.Citation41 The Bacteroides genus is enriched in L-ornithine biosynthesis and L-histidine degradation. L-ornithine, the intermediate metabolites of L-arginine metabolism, is involved in both the urea cycle and nitric oxide synthesisCitation42 and is related to Bacteroides metabolic pathways.Citation43
Consistent with the predicted functional pathway, non-targeted metabolomics revealed increased levels of specific amino acids and their metabolic products in patients with ALS after FMT, particularly in the arginine biosynthesis pathway. For example, L-Arginine can delay skeletal muscle atrophy,Citation44 promote muscle growth, maintain muscle function, and protect cells from oxidative damage.Citation45 In addition, animal studies show that L-Arginine supplementation can extend the lifespan of SOD1 mice.Citation46
Our study has some limitations. First, this case study was limited by its small sample size and warrants further validation through large-scale clinical trials. Second, the follow-up period of patients in this study was 4–6 months, which only reflects the short-term efficacy of FMT. Long-term follow-up is needed to comprehensively evaluate the impact of FMT on ALS development.
In conclusion, this study suggests that FMT could be a potential rescue therapy for ALS patients with respiratory failure. The therapeutic mechanism may hinge on modulating the gut microbiota, increasing the abundance of beneficial bacteria (Bacteroides species and F. prausnitzii) and changing metabolic pathways such as those related to arginine biosynthesis. These findings offer new insights and potential targets for the understanding and treatment of ALS.
Ethics declarations
This study obtained approval from the Medical Ethics Committee of the Chinese PLA General Hospital (Approval No. S2019-061-01). The authors confirm that written informed consent was obtained from the patients and their authorized representatives. The authorized representatives signed informed consent forms for the FMT treatment and for the publication of videos and other clinical information in the journal.
Supplemental Material
Download Zip (844.7 KB)Acknowledgments
We express our gratitude to the patients and their families who participated in this study. We also thank the healthcare professionals in the Department of Neurology for their care of the patients.
Disclosure statement
No potential conflict of interest was reported by the author(s).
Data availability statement
The original metagenomic sequencing and metabolomic data in this study have been deposited in the National Center for Biotechnology Information and will be accessible with accession numbers PRJNA1025870 and MTBLS8716 upon publication.
Supplementary material
Supplemental data for this article can be accessed online at https://doi.org/10.1080/19490976.2024.2353396
Additional information
Funding
References
- Oskarsson B, Gendron TF, Staff NP. Amyotrophic lateral sclerosis: an update for 2018. Mayo Clin Proc. 2018;93(11):1617–12. doi:10.1016/j.mayocp.2018.04.007.
- Hardiman O, Al-Chalabi A, Chio A, Corr EM, Logroscino G, Robberecht W, Shaw PJ, Simmons Z, van den Berg LH. Amyotrophic lateral sclerosis. Nat Rev Dis Primers. 2017;3(1):17071. doi:10.1038/nrdp.2017.71.
- Feldman EL, Goutman SA, Petri S, Mazzini L, Savelieff MG, Shaw PJ, Sobue G. Amyotrophic lateral sclerosis. Lancet (London, England). 2022;400(10360):1363–1380. doi:10.1016/S0140-6736(22)01272-7.
- Nicholson K, Bjornevik K, Abu-Ali G, Chan J, Cortese M, Dedi B, Jeon M, Xavier R, Huttenhower C, Ascherio A. et al. The human gut microbiota in people with amyotrophic lateral sclerosis. Amyotroph Lateral Scler Frontotemporal Degener. 2021;22(3–4):186–194. doi:10.1080/21678421.2020.1828475.
- Di Gioia D, Bozzi Cionci N, Baffoni L, Amoruso A, Pane M, Mogna L, Gaggìa F, Lucenti MA, Bersano E, Cantello R. et al. A prospective longitudinal study on the microbiota composition in amyotrophic lateral sclerosis. BMC Med. 2020;18(1):153. doi:10.1186/s12916-020-01607-9.
- Fang X, Wang X, Yang S, Meng F, Wang X, Wei H, Chen T. Evaluation of the microbial diversity in amyotrophic lateral sclerosis using high-throughput sequencing. Front Microbiol. 2016;7:1479. doi:10.3389/fmicb.2016.01479.
- Blacher E, Bashiardes S, Shapiro H, Rothschild D, Mor U, Dori-Bachash M, Kleimeyer C, Moresi C, Harnik Y, Zur M. et al. Potential roles of gut microbiome and metabolites in modulating ALS in mice. Nature. 2019;572(7770):474–480. doi:10.1038/s41586-019-1443-5.
- Zhang Y, Ogbu D, Garrett S, Xia Y, Sun J. Aberrant enteric neuromuscular system and dysbiosis in amyotrophic lateral sclerosis. Gut Microbes. 2021;13(1):1996848. doi:10.1080/19490976.2021.1996848.
- Abe K, Aoki M, Tsuji S, Itoyama Y, Sobue G, Togo M, Hamada C, Tanaka M, Akimoto M, Nakamura K. Safety and efficacy of edaravone in well defined patients with amyotrophic lateral sclerosis: a randomised, double-blind, placebo-controlled trial. Lancet Neurol. 2017;16(7):505–512. doi:10.1016/S1474-4422(17)30115-1.
- Andrews JA, Jackson CE, Heiman-Patterson TD, Bettica P, Brooks BR, Pioro EP. Real-world evidence of riluzole effectiveness in treating amyotrophic lateral sclerosis. Amyotroph Lateral Scler Frontotemporal Degener. 2020;21(7–8):509–518. doi:10.1080/21678421.2020.1771734.
- Bensimon G, Lacomblez L, Meininger V. A controlled trial of riluzole in amyotrophic lateral sclerosis. ALS/Riluzole study group. N Engl J Med. 1994;330(9):585–591. doi:10.1056/NEJM199403033300901.
- Abe K, Itoyama Y, Sobue G, Tsuji S, Aoki M, Doyu M, Hamada C, Kondo K, Yoneoka T, Akimoto M. et al. Confirmatory double-blind, parallel-group, placebo-controlled study of efficacy and safety of edaravone (MCI-186) in amyotrophic lateral sclerosis patients. Amyotroph Lateral Scler Frontotemporal Degener. 2014;15(7–8):610–617. doi:10.3109/21678421.2014.959024.
- Writing Group on behalf of the Edaravone (MCI-186) ALS 18 Study Group. Exploratory double-blind, parallel-group, placebo-controlled study of edaravone (MCI-186) in amyotrophic lateral sclerosis (Japan ALS severity classification: grade 3, requiring assistance for eating, excretion or ambulation). Amyotroph Lateral Scler Frontotemporal Degener. 2017;18(sup1):40–48. doi:10.1080/21678421.2017.1361441.
- Witzel S, Maier A, Steinbach R, Grosskreutz J, Koch JC, Sarikidi A, Petri S, Günther R, Wolf J, Hermann A. et al. Safety and effectiveness of long-term intravenous administration of edaravone for treatment of patients with amyotrophic lateral sclerosis. JAMA Neurol. 2022;79(2):121–130. doi:10.1001/jamaneurol.2021.4893.
- Gupta A, Khanna S. Fecal Microbiota Transplantation. JAMA. 2017;318(1):102. doi:10.1001/jama.2017.6466.
- Vendrik KEW, Ooijevaar RE, De Jong PRC, Laman JD, van Oosten BW, van Hilten JJ, Ducarmon QR, Keller JJ, Kuijper EJ, Contarino MF. Fecal microbiota transplantation in neurological disorders. Front Cell Infect Microbiol. 2020;10:98. doi:10.3389/fcimb.2020.00098.
- Mandrioli J, Amedei A, Cammarota G, Niccolai E, Zucchi E, D’Amico R, Ricci F, Quaranta G, Spanu T, Masucci L. et al. FETR-ALS study protocol: a randomized clinical trial of fecal microbiota transplantation in amyotrophic lateral sclerosis. Front Neurol. 2019;10:1021. doi:10.3389/fneur.2019.01021.
- Lu G, Wen Q, Cui B, Li Q, Zhang F. Washed microbiota transplantation stopped the deterioration of amyotrophic lateral sclerosis: the first case report and narrative review. J Biomed Res. 2022;37(1):1–8. doi:10.7555/JBR.36.20220088.
- Brooks BR, Miller RG, Swash M, Munsat TL. El Escorial revisited: revised criteria for the diagnosis of amyotrophic lateral sclerosis. Amyotroph Lateral Scler Other Motor Neuron Disord. 2000;1(5):293–299. doi:10.1080/146608200300079536.
- Ren R, Gao X, Shi Y, Li J, Peng L, Sun G, Wang Z, Yan B, Zhi J, Yang Y. et al. Long-term efficacy of low-intensity single donor fecal microbiota transplantation in ulcerative colitis and outcome-specific gut bacteria. Front Microbiol. 2021;12:742255. doi:10.3389/fmicb.2021.742255.
- Cedarbaum JM, Stambler N, Malta E, Fuller C, Hilt D, Thurmond B, Nakanishi A. The ALSFRS-R: a revised ALS functional rating scale that incorporates assessments of respiratory function. BDNF ALS study group (phase III). J Neurol Sci. 1999;169(1–2):13–21. doi:10.1016/S0022-510X(99)00210-5.
- Beghini F, Mciver LJ, Blanco-Míguez A, Dubois L, Asnicar F, Maharjan S, Mailyan A, Manghi P, Scholz M, Thomas AM. et al. Integrating taxonomic, functional, and strain-level profiling of diverse microbial communities with bioBakery 3. eLife. 2021;10. doi:10.7554/eLife.65088.
- Caspi R, Altman T, Billington R, Dreher K, Foerster H, Fulcher CA, Holland TA, Keseler IM, Kothari A, Kubo A. et al. The MetaCyc database of metabolic pathways and enzymes and the BioCyc collection of pathway/genome databases. Nucleic Acids Res. 2014;42(Database issue):D459–71. doi:10.1093/nar/gkt1103.
- Braun AT, Caballero-Eraso C, Lechtzin N. Amyotrophic lateral sclerosis and the respiratory system. Clin Chest Med. 2018;39(2):391–400. doi:10.1016/j.ccm.2018.01.003.
- Plowman EK, Gray LT, Chapin J, Anderson A, Vasilopoulos T, Gooch C, Vu T, Wymer JP. Respiratory strength training in amyotrophic lateral sclerosis: a double-blind, randomized, multicenter, sham-controlled trial. Neurology. 2023;100(15):e1634–e42. doi:10.1212/WNL.0000000000206830.
- Bedlack RS, Vaughan T, Wicks P, Heywood J, Sinani E, Selsov R, Macklin EA, Schoenfeld D, Cudkowicz M, Sherman A. How common are ALS plateaus and reversals? Neurology. 2016;86(9):808–812. doi:10.1212/WNL.0000000000002251.
- Hu N, Shen D, Yang X, Cui L, Liu M. Plateaus and reversals evaluated by different methods in patients with limb-onset amyotrophic lateral sclerosis. J Clin Neurosci. 2022;97:93–98. doi:10.1016/j.jocn.2022.01.016.
- Vasta R, D’ovidio F, Canosa A, Manera U, Torrieri MC, Grassano M, de Marchi F, Mazzini L, Moglia C, Calvo A. et al. Plateaus in amyotrophic lateral sclerosis progression: results from a population-based cohort. Eur J Neurol. 2020;27(8):1397–1404. doi:10.1111/ene.14287.
- Hu N, Shen D, Yang X, Cui L, Liu M. The frequency of ALSFRS-R reversals and plateaus in patients with limb-onset amyotrophic lateral sclerosis: a cohort study. Acta Neurol Belg. 2022;122(6):1567–1573. doi:10.1007/s13760-021-01849-1.
- Harrison D, Mehta P, van Es MA, Stommel E, Drory VE, Nefussy B, van den Berg LH, Crayle J, Bedlack R. “ALS reversals”: demographics, disease characteristics, treatments, and co-morbidities. Amyotroph Lateral Scler Frontotemporal Degener. 2018;19(7–8):495–499. doi:10.1080/21678421.2018.1457059.
- Spataro R, Bono V, Marchese S, la Bella V. Tracheostomy mechanical ventilation in patients with amyotrophic lateral sclerosis: clinical features and survival analysis. J Neurol Sci. 2012;323(1–2):66–70. doi:10.1016/j.jns.2012.08.011.
- Lo Coco D, Marchese S, La Bella V, Piccoli T, Lo Coco A. The amyotrophic lateral sclerosis functional rating scale predicts survival time in amyotrophic lateral sclerosis patients on invasive mechanical ventilation. Chest. 2007;132(1):64–69. doi:10.1378/chest.06-2712.
- Yeung J, Couper K, Ryan EG, Gates S, Hart N, Perkins GD. Non-invasive ventilation as a strategy for weaning from invasive mechanical ventilation: a systematic review and Bayesian meta-analysis. Intensive Care Med. 2018;44(12):2192–2204. doi:10.1007/s00134-018-5434-z.
- McCombe PA, Henderson RD, Lee A, Lee JD, Woodruff TM, Restuadi R, McRae A, Wray NR, Ngo S, Steyn FJ. Gut microbiota in ALS: possible role in pathogenesis? Expert Rev Neurother. 2019;19(9):785–805. doi:10.1080/14737175.2019.1623026.
- Kim HS, Son J, Lee D, Tsai J, Wang D, Chocron ES, Jeong S, Kittrell P, Murchison CF, Kennedy RE. et al. Gut- and oral-dysbiosis differentially impact spinal- and bulbar-onset ALS, predicting ALS severity and potentially determining the location of disease onset. BMC Neurol. 2022;22(1):62. doi:10.1186/s12883-022-02586-5.
- Zhai CD, Zheng JJ, An BC, Huang HF, Tan ZC. Intestinal microbiota composition in patients with amyotrophic lateral sclerosis: establishment of bacterial and archaeal communities analyses. Chin Med J. 2019;132(15):1815–1822. doi:10.1097/CM9.0000000000000351.
- Dupuis L, Pradat PF, Ludolph AC, Loeffler JP. Energy metabolism in amyotrophic lateral sclerosis. Lancet Neurol. 2011;10(1):75–82. doi:10.1016/S1474-4422(10)70224-6.
- Manzo E, Lorenzini I, Barrameda D, O’Conner AG, Barrows JM, Starr A, Kovalik T, Rabichow BE, Lehmkuhl EM, Shreiner DD. et al. Glycolysis upregulation is neuroprotective as a compensatory mechanism in ALS. eLife. 2019;8:8. doi:10.7554/eLife.45114.
- Bell SM, Burgess T, Lee J, Blackburn DJ, Allen SP, Mortiboys H. Peripheral glycolysis in neurodegenerative diseases. IJMS. 2020;21(23):8924. doi:10.3390/ijms21238924.
- Tang BL. Glucose, glycolysis, and neurodegenerative diseases. J Cell Physiol. 2020;235(11):7653–7662. doi:10.1002/jcp.29682.
- Chen J, Siliceo SL, Ni Y, Nielsen HB, Xu A, Panagiotou G. Identification of robust and generalizable biomarkers for microbiome-based stratification in lifestyle interventions. Microbiome. 2023;11(1):178. doi:10.1186/s40168-023-01604-z.
- Wu G, Meininger CJ, Mcneal CJ, Bazer FW, Rhoads JM. Role of L-Arginine in nitric oxide synthesis and health in humans. Adv Exp Med Biol. 2021;1332:167–187. doi:10.1007/978-3-030-74180-8_10.
- Zhang S, Qian Y, Li Q, Xu X, Li X, Wang C, Cai H, Zhu J, Yu Y. Metabolic and neural mechanisms underlying the associations between gut bacteroides and cognition: a large-scale functional network connectivity study. Front Neurosci. 2021;15:750704. doi:10.3389/fnins.2021.750704.
- Ham DJ, Caldow MK, Lynch GS, Koopman R. Arginine protects muscle cells from wasting in vitro in an mTORC1-dependent and NO-independent manner. Amino Acids. 2014;46(12):2643–2652. doi:10.1007/s00726-014-1815-y.
- Latif S, Kang YS. Differences of transport activity of arginine and regulation on neuronal nitric oxide synthase and oxidative stress in amyotrophic lateral sclerosis model cell lines. Cells. 2021;10(12):3554. doi:10.3390/cells10123554.
- Lee J, Ryu H, Kowall NW. Motor neuronal protection by L-arginine prolongs survival of mutant SOD1 (G93A) ALS mice. Biochem Bioph Res Co. 2009;384(4):524–529. doi:10.1016/j.bbrc.2009.05.015.