ABSTRACT
The emergence of antimicrobial resistance (AMR) is a principal global health crisis projected to cause 10 million deaths annually worldwide by 2050. While the Gram-negative bacteria Escherichia coli is commonly found as a commensal microbe in the human gut, some strains are dangerously pathogenic, contributing to the highest AMR-associated mortality. Strains of E. coli that can translocate from the gastrointestinal tract to distal sites, called extraintestinal E. coli (ExPEC), are particularly problematic and predominantly afflict women, the elderly, and immunocompromised populations. Despite nearly 40 years of clinical trials, there is still no vaccine against ExPEC. One reason for this is the remarkable diversity in the ExPEC pangenome across pathotypes, clades, and strains, with hundreds of genes associated with pathogenesis including toxins, adhesins, and nutrient acquisition systems. Further, ExPEC is intimately associated with human mucosal surfaces and has evolved creative strategies to avoid the immune system. This review summarizes previous and ongoing preclinical and clinical ExPEC vaccine research efforts to help identify key gaps in knowledge and remaining challenges.
1. Introduction
Pathogenic Escherichia coli is an important global health concern. While intestinal pathogenic E. coli (InPEC) plays a vital role in worldwide diarrheal diseases,Citation1 extraintestinal pathogenic E. coli (ExPEC) is responsible for various diseases at non-intestinal sites.Citation2 According to the types of diseases caused in humans, ExPEC strains are divided into three major pathotypes (): uropathogenic E. coli (UPEC), sepsis-causing E. coli (SEPEC), and neonatal meningitis-associated E. coli (NMEC).Citation3 Among all infections caused by ExPECs, the urinary tract is the most common site of infection.Citation4 UPEC is the primary cause of urinary tract infections (UTIs), accounting for 80% of all cases.Citation4,Citation5 UTI is not only a common infection, with over 60% of all women diagnosed at least once during their lifetime, but also incredibly difficult to eliminate.Citation6 More than 30% of women are plagued by a secondary infection within 12 months.Citation6 Globally, antibiotic resistant ExPEC strains are emerging, adding additional complexity to the treatment of these infections. Given these implications, the development of effective vaccines targeting ExPEC becomes of pivotal importance. As such, the goal of this review is to summarize the extensive body of research that has been carried out toward this end.
Figure 1. ExPEC reservoir and infection sites.
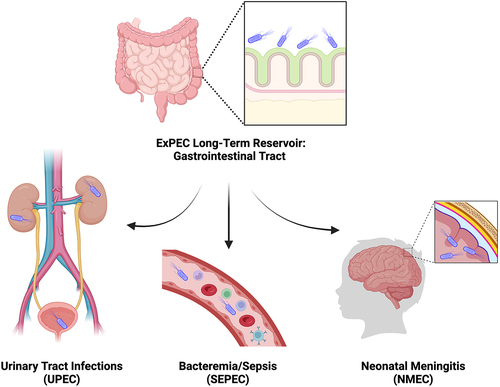
2. Review of prior vaccination attempts for ExPEC
This section will provide an overview of the previous vaccine formulations attempted to protect against disease caused by ExPEC, considering both preclinical development and clinical research. To this end, we evaluated 27 prospective human vaccine trials for ExPEC-associated disease with endpoints for safety, immunogenicity, and/or protective efficacy. We organize these vaccines by their general approach () and contextualize them in the body of basic and preclinical literature that has motivated their progression to clinical trials.
Figure 2. Considerations for antigen and vaccine type selection.
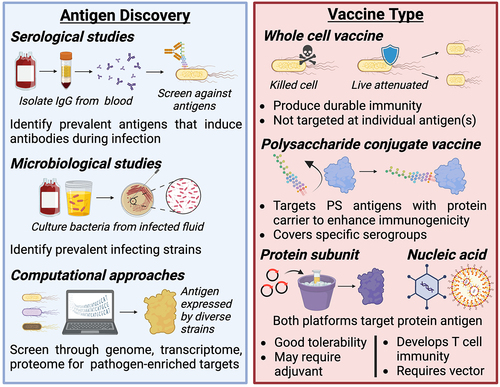
2.1. Traditional vaccinology
Traditional vaccine design refers to the use of whole pathogens in either live-attenuated or killed formulations to elicit a protective immune response. This method of vaccine development represents many of the original vaccines that revolutionized public health. Early vaccination efforts were directed against viral pathogens such as smallpox, rabies, and typhoid. However, this method was later applied against bacterial pathogens including the Bacillus Calmette-Guerin vaccine for tuberculosis and Bordetella pertussis, which in combination with diphtheria and tetanus toxoid subunits constitutes the first general population bacterial vaccine. Traditional vaccines remain in use today and have grown to include vaccines against measles, mumps, and rubella (MMR) and annual influenza strains, among others. These vaccines have strong immunogenicity due to their full repertoire of pathogen-associated molecular patterns that initiate innate immune receptor signaling. As such, they may not require adjuvants and generally induce robust, enduring immune responses without frequent boosters. This is especially true for live-attenuated vaccines since the live pathogen retains its capacity to replicate and thus prolongs antigen exposure. However, using live pathogens carries greater safety concerns, particularly in an immunocompromised host, due to the possibility of reversion to virulence or person-to-person transmission. Outbreaks of virulent poliovirus, for example, have been reported after several independent reversions of the oral attenuated virus vaccine.Citation7
Advancements in traditional vaccine platforms may improve the safety profile of these live-attenuated vaccines. Contemporary gene editing technologies, for example, have achieved pathogen attenuation without risk of reversion by targeting key virulence factors for deletion. In the context of ExPEC, live-attenuated vaccines have been developed using genetic engineering approaches with some success in a mouse model of UTI. For example, Billips et al.Citation8 generated an attenuated NU14 strain, an antibiotic-resistant clinical isolate of cystitis, with a targeted deletion of the O antigen ligase that attaches O antigens to the lipid A core of lipopolysaccharide (LPS). This mutant strain, ΔwaaL NU14, was nonpathogenic, induced cytokine secretion, and yielded a two-log reduction in murine bladder colonization upon challenge with wild-type NU14.Citation8
Genetic engineering can also steer the immune response toward or away from specific antigens. For example, a killed mutant strain deficient in polysaccharide capsule was used to direct adaptive immunity away from capsular antigens, which are less conserved between strains and have variable immunogenicity.Citation9,Citation10 However, despite improved IgG and IgA responses relative to killed wild-type bacteria,Citation9 this vaccine was not protective against a murine model of sepsis.Citation10 It remains to be seen if genetically engineered whole-cell vaccines will prove a viable strategy in a human host.
To date, clinical trials with whole-cell vaccines against ExPEC have only investigated killed, wild-type strains. Three such vaccines – Uro-Vaxom, Solco-Urovac, and Uromune – have been evaluated in clinical trials for protection against recurrent UTI and are discussed below. Of note, evidence for antigen-specific adaptive immune responses generated by these vaccines is lacking, prompting some to refer to these instead as immune active or stimulating agents.Citation11 Various other killed whole-cell vaccines (e.g. Urvakol) are available in countries around the globe, but have no clinical evidence supporting their efficacy.
2.1.1. Uro-Vaxom
Uro-Vaxom, also known as OM-8930 or OM-89, is a lysate fraction vaccine of extracted outer membrane proteins from lyophilized, killed cocktails of select E. coli strains delivered in a glycerin capsule for oral consumption.Citation12 This was the first vaccine brought to human trials against UPEC (and more generally UTI) in 1986 and is extensively studied with 8 clinical trials.Citation13 These trials have predominantly recruited outpatient women enrolled following antibiotic treatment for acute UTI. Participants received oral capsules daily for 3 months. Primary endpoints for these trials included:
Incidence of recurrence, with varying definitions and criteria across trials, including symptomatic UTI/cystitis,Citation14–18 bacteriuria,Citation12,Citation13,Citation17,Citation19 dysuria,Citation13,Citation16,Citation17 or leukocyturia.Citation13,Citation16,Citation17 Uro-Vaxom significantly reduced (by a factor of 1.6 - 5.8) the recurrence of symptomatic UTI/cystitis,Citation15–18 as well as lowered the incidence of leukocyturiaCitation13,Citation17 and bacteriuria.Citation12,Citation13,Citation17,Citation19 Dysuria was significantly reduced in 2 of the 3 trials measuring that outcome.Citation13,Citation17 Bauer et al. found that 55% of treated patients had no recurrences compared to 42% in the control arm.Citation16
Concomitant antimicrobial therapy, defined as the number of days necessitating antimicrobial treatment,Citation18,Citation19 the average duration of treatment,Citation13 or the number of prescriptions written.Citation12,Citation16 All metrics were reduced across trials. However, the Hachen trial found that this effect was not sustained after halting daily administration.Citation12
Nonetheless, there are also significant limitations associated with these trials and the outlook for Uro-Vaxom. First, most of these studies included a post-treatment observational period of only 3 months,Citation12–14,Citation17,Citation19 and thus it is difficult to know the duration of the protective effect. This concern was partly addressed by TammenCitation18 and Kim et al.Citation15, whose trials extended the observational period to eight or 6 months after cessation, respectively, and found sustained protection against recurrence of cystitis and bacteriuria. However, Bauer et al.Citation16 found that a booster treatment between months six to nine was necessary to maintain protection through a 12-month trial.
Additionally, efficacy was only tested in patients with acute UTI and a history of recurrences. Given the incomplete protection against recurrence and the apparent lack of durable, pathogen-specific immunologic memory, the prophylactic potential of this vaccine to prevent UTI and its complications altogether remains unlikely. Nevertheless, Uro-Vaxom has been approved for use in various countries including Switzerland and the UK. Its application to complicated UTI, namely in patients with neurogenic bladder disorders, is being explored in clinical trials.Citation12,Citation14,Citation20,Citation21
2.1.2. Solco-Urovac
In contrast to the fractionated lysate used in Uro-Vaxom, Solco-Urovac (also called StroVac) is a heat-inactivated whole-cell vaccine derived from 10 strains of uropathogenic organisms, including six diverse E. coli strains, Proteus mirabilis, Morganella morganii, Klebsiella pneumoniae, and Enterococcus faecalis.Citation22 This vaccine has undergone 8 trials since 1987.Citation23
Three different routes of administration were tested for Solco-Urovac: vaginal suppositoryCitation14–22,Citation23–27 and intraglutealCitation23,Citation28 or intramuscularCitation29 injections, each with three doses. The following primary endpoints were analyzed:
Recurrence of UTI, measured by the interval until,Citation22,Citation25–27 and numberCitation27,Citation29 or frequencyCitation23,Citation28 of, recurrences. Both trials measuring frequency had significant reductions from Solco-Urovac treatment.Citation23,Citation28 Interval until recurrence had mixed outcomes with two of the four trials showing delays due to treatment.Citation25,Citation26 Number of recurrences was not reduced in Nestler et al.Citation29 or Uehling et al. (1997).Citation27
Induction of antibodies, measured with serum, urine, and/or vaginal IgG, IgM, and IgA titers or ELISA concentrations.Citation22,Citation24–28 Uehling et al. (1994)Citation24 observed a significant increase in total (nonspecific) IgG and vaginal IgA after Solco-Urovac administration. Urinary IgG and IgA also increased, both transientlyCitation24 and sustained with a booster.Citation28 Others reported no difference in total serumCitation25,Citation26 or vaginal and urinary IgCitation27. Further, these trials consistently failed to induce E. coli-specific Ig.Citation16,Citation22,Citation27 Despite nonspecific antibody responses, incidence of UPEC UTIs had greater reductions than other uropathogens.Citation22
These modest outcomes combined with questions about trial design may limit the vaccine’s prospects in the US. For example, three of the eight trials were not blinded and trials were consistently underpowered to detect primary endpoints. Antibiotic prophylaxis may also have confounded interpretation of the data.Citation27 Finally, a lack of E. coli-specific immune responses raises doubts about Solco-Urovac’s induction of durable, prophylactic immunity against UPEC.
2.1.3. Uromune
Uromune, also called MV140, is a heat-inactivated whole-cell sublingual spray containing E. coli, K. pneumoniae, E. faecalis, and P. vulgaris. The first studies of Uromune came in 2013Citation30 and 2015Citation31 with two retrospective cohort analyses showing that women with recurrent UTI who were treated with Uromune experienced significantly fewer recurrencesCitation30 and were less likely to experience any recurrence at all.Citation31 However, without randomization or placebo, no firm conclusions about efficacy could be drawn.
The first prospective clinical trial of Uromune partially addressed these limitations but was not placebo controlled. This trial compared the frequency of UTI recurrences for 12 months before and after a three-month treatment.Citation32 All participants had failed antibiotic prophylaxis and experienced ≥3 recurrences prior to treatment; 22% had a recurrence following treatment.Citation32 A follow-up double-blind, randomized, placebo-controlled trial published in 2022 evaluated three- or six-month treatments with a nine-month study period. Frequency of recurrences, the primary endpoint, was significantly greater in the placebo group than in either treatment course (median 3 vs. 0 recurrences over 9 months; p < .001). Interval until recurrence was also significantly delayed by treatment (median 48 vs. 275 days for those with recurrence(s)).Citation33
No data on antigen-specific adaptive immune responses were collected for these trials. However, in vitro treatment of human peripheral blood mononuclear cells (PBMCs) with Uromune activated dendritic cells (DCs) to stimulate differentiation of Th1 and Th17 cells.Citation34 This phenotype was recapitulated in mice.
2.2. Polysaccharide conjugate vaccines
Polysaccharide (PS) vaccines have been pursued for decades due to the ubiquity of PS structures at the surface of bacterial pathogens. However, extracellular PS are present in part to shield the pathogen from immune recognition. By themselves, PS are generally not strong immunogens because they do not stimulate CD4+ T cells, which are required for efficient expansion and differentiation of naïve B cells, antibody class switching, and affinity maturation. However, it was discovered that conjugating PS antigens to an immunogenic carrier protein can recruit CD4+ T cell help, leading to multiple PS-conjugate vaccines now being available, including for Haemophilus influenzae type b, Streptococcus pneumoniae, and meningococcus.
ExPEC strains exhibit a diverse array of PS antigens on their cell surface (). Among these, O and K antigens are the best characterized PS vaccine targets. Both antigens are polymerized chains of branched or linear sugar residue sequences (2–7 sugars in length) that decorate the exterior of the bacteria outer membrane. The unique sequence and structure for O and K antigens are determined by gene clusters encoding proteins that synthesize and modify sugar precursors, covalently link these sugars together, then translocate and polymerize them. O antigens are anchored to the outer membrane by attachment to lipid A and a PS core structure that together form lipopolysaccharide (LPS), an important structural component that stabilizes the outer membrane. K antigens sheath the bacteria with a superficial layer of LPS-anchored and unanchored PS units that collectively form the capsule. This structure protects bacteria from host defenses and environmental insults (e.g. desiccation). In total, there are 185 E. coli distinct O serogroups and over 80 distinct K serogroups.Citation35,Citation36
Figure 3. ExPEC vaccine antigen targets.
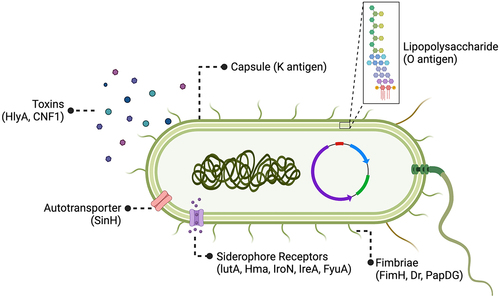
Early research demonstrated humoral immunity against these antigens in patients with ExPEC-associated pyelonephritis or bacteremia, with anti-O immunity being more common (Serological studies, ).Citation37,Citation38 Attempts with traditional vaccinology also suggest these antigens may be necessary and sufficient for adaptive humoral immune protection. Immunization with mutant E. coli deficient in O antigen and capsular PS was not protective against sepsis in mice despite strong induction of antibodies against surface protein targets.Citation9 However, vaccination with killed wild-type pathogen has produced mixed results for generating anti-O and anti-K immunity. For example, one study generated anti-O2/O6 and anti-K1/K13 immune responses with killed pathogen that were sufficient to protect against intraperitoneal (i.p.) challenge with homologous strains,Citation39 while another study failed to produce anti-K1 antibodies with this approach.Citation40
Beyond traditional vaccinology approaches, proof-of-principle preclinical research in animals has indicated that O and K antigens can be formulated into immunogenic subunit vaccines. For example, the K1 antigen that was poorly immunogenic in killed cell vaccines was administered as purified PS with or without a carrier protein. Although the unconjugated K1 antigen failed to generate anti-K1 immunity,Citation41 protein conjugation improved immune responses and partially protected against pyelonephritis in rats.Citation41,Citation42 Vaccination against individual O antigens also showed promise in animals. For example, the O25b antigen, representing a prevalent ExPEC serotype, induced robust IgG responses in both mice and cynomolgus macaques.Citation43–45 The functionality of these antibodies was demonstrated with in vitro opsonophagocytosis assays. However, validating the functionality of these antibodies in vivo through clinical trials is necessary to confirm efficacy. As such, these animal studies paved the way for human clinical trials, all of which target combinations of clinically relevant O antigens.
2.2.1. Clinical trials of O antigen vaccines
Six clinical trials have been conducted for four different PS conjugate vaccines against UPEC, starting as early as 1991.Citation46 All these trials have targeted O antigens of LPS. Cryz et al.Citation46 brought the first such PS vaccine into clinical trials with a monovalent O18 antigen conjugated to cholera toxin or Pseudomonas aeruginosa toxin A carrier proteins. Both formulations were immunogenic and generated functional antibodies eliciting PBMC clearance of homologous bacteria in vitro. Further, passive immunization with participant sera protected rabbits against fatal i.p. challenge with O18 E. coli.Citation46 This group later expanded the coverage of their PS-conjugate vaccine by isolating detoxified LPS from 12 serogroups of E. coli and conjugating the polyvalent polysaccharide mixture to the P. aeruginosa toxin A.Citation47 This vaccine also generated high titers of antigen-specific IgG that persisted over 6 months. Serum IgG against eight of the 12 serogroups induced robust (≥70%) bacterial clearance in vitro.
A Phase I dose escalation clinical trial with a different formulation, J5dLPS/OMP, was carried out by this group in 2003.Citation48 This included the conserved core saccharides from J5 strain LPS, detoxified and conjugated to group B meningococcus outer membrane protein (OMP). Each dose escalation induced significantly greater levels of IgG, IgA, and IgM specific for J5dLPS. This translated to significantly greater bacterial clearance in bacteremic rabbits receiving serum Ig from immunized human patients compared to pre-immunized control serum. Titers of anti-J5dLPS were also negatively associated with TNF, IL-6, and IL-10 cytokine secretion (markers of septic shock) in an in vitro whole blood infection assay. An additional Phase I trial by Cross et al. in 2015 explored J5dLPS/OMP vaccine immunogenicity for protection against Gram-negative bacteremia. Results trended toward sustained anti-LPS IgG and IgM responses after 180 but not 236 days.Citation49 However, the trial was underpowered due to recruitment ending prematurely.
Despite significant improvements in the designated endpoints produced by each of these trials, neither vaccine formulation advanced to efficacy studies. Nevertheless, these trials paved the way for the polysaccharide conjugate vaccine ExPEC4V. This vaccine includes four of the most prevalent O antigens in UTI clinical isolates conjugated to the P. aeruginosa exotoxin A carrier. Preclinical testing showed robust antigen-specific IgG responses across rabbit, mouse, and rat animal models.Citation50 Safety and immunogenicity in humans were evaluated in three trials. Two Phase I trials demonstrated tolerability and robust induction of serum IgG increased for all antigens. The vaccine also significantly reduced the number of UTIs experienced by participants despite not being powered for efficacy assessment.Citation51,Citation52 The Phase II trial by Frenck et al.Citation53 reported 80% of participants had a minimum two-fold increase in serotype-specific serum IgG.
An updated vaccine formulation, ExPEC9V, with a nine-valent conjugated PS pool is currently undergoing Phase III clinical trials (NCT04899336). This vaccine follows recently published Phase I and II safety and immunogenicity trials carried out in older adults, age 60–85, with a 10-valent formulation ExPEC10V. Similar to the four-valent formulation, this vaccine was well tolerated and induced antigen-specific antibodies in most of the participants despite their advanced age.Citation54 However, unlike the previous clinical trials, the Phase III study’s primary endpoints evaluate the number of first invasive E. coli disease events experienced by patients, in which blood or other sterile tissues are infected with E. coli that is microbiologically confirmed to match an O group contained within the vaccine.
Increasing the number of O antigens and testing efficacy against systemic infection may be seen as an attempt to overcome the significant antigenic heterogeneity of ExPEC, as fewer serogroups comprise the majority of invasive infections than for less invasive infections such as cystitis. For example, the 4 O-serotypes chosen for ExPEC4V cover only 30–35% of E. coli cystitis isolates, although the authors cite unpublished data implicating 12 O-serogroups as the predominant drivers of UTI (Microbiological studies, ).Citation55 They do not comment on the coverage of the O antigens in invasive E. coli disease (infecting blood or sterile tissue), which is the endpoint for their current Phase III ExPEC9V trial. However, a recent and comprehensive study of thousands of ExPEC bacteremia cases across four continents reported that the nine most prevalent O-serotypes represent 64.6% of E. coli blood isolates.Citation56 Assuming O antigens were selected on the basis of their prevalence in E. coli blood isolates (constituting invasive disease), these nine serogroups account for 34–58% of E. coli cystitis and 47–66% of E. coli pyelonephritis isolates observed in two smaller studies.Citation57,Citation58 Thus, vaccination against O antigens may not be fully protective, even against invasive disease (although the trial will analyze only invasive E. coli disease events with strains of the same O groups as those in the ExPECV9 vaccine).
2.3. Protein subunit vaccines
Recombinant protein vaccines direct the adaptive immune response against specific antigens important for microbial pathogenesis. For ExPEC, protein virulence factors (VFs) mediate diverse functions such as host cell adherence, motility, micronutrient acquisition, and toxin delivery ().Citation59 However, identifying ExPEC VF targets has been tricky due to complex interactions between factors such as: (1) the genetic diversity of strains; (2) the immense number of putative VFs that act in a context-dependent way (e.g. mediating virulence only in combination with other factors); (3) horizontal gene transfer of mobile gene elements. This complexity yields both independent and redundant pathways for ExPEC pathogenesis.Citation60
Identifying relevant antigen targets for subunit vaccines therefore requires some deconvolution. This effort has been accelerated by reverse vaccinology, an innovative approach that screens pathogen genomes to identify potential VFs. Indeed, comparative genomics has already been used to identify pathogenicity islands specific to pathogenic E. coli strains.Citation61,Citation62 Our group recently mapped the conservation of the E. coli virulome (encompassing all genes with known pathogenic roles) across all strains with published genomes to identify targets specific to – and broadly conserved among – pathogenic E. coli.Citation63 The autotransporter invasin-like SinH VF identified in this study was broadly protective against ExPEC phylogroups in a murine model of sepsis, including mortality and dissemination to liver, spleen, and kidneys. This vaccine also protected against cystitis in mice challenged with select strains.Citation64
These genomic technologies can further be bolstered by RNA-Seq and proteomic studies to validate expression of these genes and explore their interactions with the host.Citation65,Citation66 Large datasets emerging from these multi-omics approaches have seen the parallel rise in the suite of bioinformatics tools encompassing predictive models for antigen structure,Citation67 epitope mapping,Citation68,Citation69 and host cross-reactivity.Citation70 Although nascent, these models have already been used to engineer chimeric vaccines linking various immunodominant epitopes from ExPEC VFs into minimal peptide vaccines,Citation62,Citation71,Citation72 including a notable multi-epitope vaccine against immunodominant peptides from the siderophore receptor IutA and the fimbrial adhesin protein FimH. This spliced peptide vaccine generated a durable (>180 days) immunologic memory response that was protective against UPEC bladder colonization (three-log reduction) in mice.Citation72
Despite the impressive antigen engineering in this study, this vaccine was not the first to target FimH or IutA antigens. These targets were selected on the basis of prior studies and their distinct mechanisms promoting pathogen survival in the bladder. FimH is an adhesin protein at the tip of the type 1 pilus that mediates attachment to the urothelium through binding of host uroplakins,Citation73 glycosylated membrane proteins that form large plaques spanning >70% of the urothelial surface. It has been shown that FimH is necessary for binding to murine and human bladder tissue in vitro and that binding can be blocked with antisera from vaccinated mice.Citation74 Further preclinical animal testing of FimH vaccines demonstrated immunogenicityCitation75–78 and protection against murine cystitis,Citation74 and against bacteriuria and leukocyturia in cynomolgus monkeys.Citation79 On the basis of these results, the FimH adhesin became the first ExPEC subunit vaccine in clinical trials in 1999 (unpublished), but the vaccine did not advance beyond Phase II trials due to a reported lack of efficacy.Citation80 Potential explanations for this lack of efficacy include: (1) lower FimH expression in the human bladder than in model systems,Citation81 (2) biphasic transcriptional regulation allowing expression to be shut off,Citation82–84 and (3) induction of antibodies that do not prevent FimH adhesion (or even enhance binding).Citation85 A key lesson from this vaccine attempt therefore is that animal and in vitro studies do not necessarily produce results predictive of protective human responses. Nonetheless, interest in a FimH-based vaccine has been renewed with a Phase I dose escalation study recently published. This vaccine seroconverted 93% of participants with no severe adverse eventsCitation86 and Phase II clinical trials are planned. Other adhesin vaccines have also been explored in preclinical studies, including a novel adhesin FdeC identified by comparative genomics,Citation87 and the well-studied Dr fimbriae and PapDG tip of P fimbriae.Citation88,Citation89 Animal studies demonstrated immune responses with reduced UTI colonization and mortality in mice immunized with Dr fimbriaeCitation88 and protection from pyelonephritis in mice vaccinated against FdeCCitation90 or mature cynomolgus monkeys immunized with PapDG.Citation89 However, as seen with the FimH vaccine attempt, it will be necessary to study these antigens’ efficacy in human clinical trials to determine their protective effect.
IutA, by contrast, is a receptor that binds siderophores (iron-chelating molecules secreted by ExPEC for iron sequestration). Siderophore receptors such as IutA traffic sequestered iron through the bacterial membrane. These and other iron uptake systems are highly upregulated during UTICitation91,Citation92 and are key mediators of UPEC pathogenesis. Indeed, iron acquisition may be required for kidney colonizationCitation93 and intracellular invasion of urothelial cells, which contributes to epithelial barrier destruction. Specifically, UPEC iron uptake increases the bacterial load of acute, highly proliferative intracellular bacterial communitiesCitation91 and facilitates formation of persistent quiescent intracellular reservoirs.Citation94
IutA was one of six iron acquisition genes identified as promising vaccine targets in a multi-omic screen of 5,379 genes with genomic, transcriptomic, and immunoproteomic datasets pertaining to the prototypic and virulent UPEC strain CFT073.Citation95 Although all six antigens induced systemic and mucosal adaptive immunity following intranasal immunization in mice, only IutA demonstrated dual protection against mouse bladder and kidney colonization by this strain (Two other iron acquisition antigens upregulated in UTI, IreA siderophore receptor and Hma heme receptor, induced a greater magnitude of organ-specific protection in kidney or bladder, respectively.Citation95). Peptides of 30 amino acids taken from conserved, surface-exposed regions of IutA also yielded two-log reductions in murine kidney colonization. Interestingly, this suggests the IutA extracellular epitope is sufficient to recapitulate the whole-antigen vaccine’s protection in the kidneys but not in the bladder.
This study also tested an extracellular epitope of the IroN antigen, a salmochelin siderophore receptor, which yielded protection against pyelonephritis comparable to the IutA epitope in these mice.Citation95 The efficacy of IroN vaccination was elaborated with whole-antigen immunization protecting against mortality in a murine lethal challenge model.Citation96 This vaccine also reduced kidney but not bladder colonization in a murine UTI model (perhaps owing to a lack of IgA responses).Citation97 However, another preclinical mouse study failed to replicate protection with whole-antigen IroN vaccination despite the induction of serum IgG, and instead identified the yersiniabactin siderophore receptor FyuA as protective against pyelonephritis in these mice.Citation98 Despite a lack of protection against cystitis in this model, others have reported FyuA vaccination reduced both bladder and kidney colonization in addition to protecting against lethal challenge in a murine host.Citation99
Similar to the chimeric FimH adhesin and IutA siderophore receptor multi-epitope vaccine, a spliced peptide vaccine containing immunodominant surface epitopes from iron acquisition receptors was attempted. This vaccine included eight epitopes from six iron acquisition genes (including FyuA, IroN, and IreA) administered as both recombinant peptide with adjuvantCitation71 or via the Salmonella type 3 secretion system (T3SS) for intracellular delivery.Citation100 Interestingly, the recombinant peptide induced both humoral and cellular immunity in mice, whereas the T3SS delivery system only induced cellular immunity. Nonetheless, only the T3SS vaccine reduced both liver and spleen colonization by UPEC following i.p. challenge, whereas the recombinant peptide only protected against liver colonization.Citation71,Citation100 This finding suggests that T cell-mediated immunity (the authors do not differentiate between CD8+ and CD4+ T cell subtypes) may constitute a correlate of protection for some ExPEC vaccine formulations in this mouse model.
Vaccines against secreted VFs have also been explored. For example, iron-chelating siderophores Ybt and Aer were conjugated to immunogenic carrier proteins and tested in a transurethral murine challenge with UPEC. A bivalent vaccine achieved two-log reduction in kidney colonization, but a more marginal reduction in bladder colonization and no reduction in bacteriuria.Citation101 This result is unsurprising because antibodies against secreted factors do not bind to bacteria to induce clearance, but instead target secreted factors that otherwise drive invasive phenotypes. Beyond siderophores, inactivated toxoids have generated neutralizing antibody responses against the α-hemolysin (HlyA) and cytotoxic necrotizing factor 1 (CNF1) toxins, which are associated with highly invasive strains of ExPECCitation63 and directly mediate cytotoxic effects.Citation102 Mice immunized against these toxins were protected against bladder pathology during UTI challenge. Interestingly, the HlyA toxoid also significantly reduced bacterial load in both urine and bladder tissue in these mice,Citation103 perhaps by limiting efficient colonization. Taken together, these results show promise for targeting secreted factors to limit the pathogenic potential of ExPEC and protect against disease severity. However, a lack of any clinical trials on this approach limits the interpretability of these data for human efficacy.
3. Immune responses or host responses to ExPEC vaccines
To dissect why past vaccine attempts have had limited success, here we summarize current knowledge of the protective immune responses for ExPEC vaccines and host immune factors that may complicate ExPEC vaccine design. We also consider approaches that have been tested to circumvent these challenges by modulating the immune response to vaccination ().
Figure 4. Considerations for adjuvant selection and delivery method.
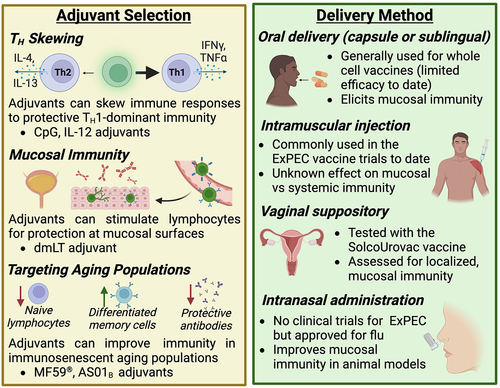
3.1. Antibodies as correlates of protection
Like any exogenous antigens, vaccines are first recognized by the host innate immune system, which then elicits the appropriate cell-mediated and/or humoral immune responses to confer protection against future infections and diseases.Citation104–106 Understanding these immunological correlates of protection (CoP) is thus crucial to develop an effective vaccine. So far, functional antibodies have been the most common CoP for available bacterial vaccines,Citation106,Citation107 including those against tetanus, diphtheria, and pertussis.Citation108 However, in rare cases, CD4+ T cell subsets have been identified as the key CoP, as with the tuberculosis BCG vaccine.Citation109,Citation110
Although no CoP has been established for immunological memory against ExPECs, previous research provided clues. According to a murine study focused on UPEC, infection evoked antigen-specific serum IgG and IgM increases and antigen-specific T cell proliferation.Citation111 Additionally, naïve mice that received adoptive transfer of serum, T cells, or splenocytes from infected mice were protected from subsequent challenge, suggesting the sufficiency of either humoral or cellular immunity against UPEC infection.Citation111 Nonetheless, most ExPEC vaccine studies to date have homed in on antibodies as potential CoP. Early work on O-antigen conjugate vaccines showed that passive immunization of mice with total serum IgG from vaccinated human volunteers conferred serospecific protection in an E. coli sepsis model.Citation46 Similarly, FimH-based vaccines produced functional antibodies that inhibit UPEC binding to human bladder epithelial cellsCitation79 and passively protect mice from bladder colonization.Citation74 One group examined iron receptor-based vaccines in mice and suggested urinary IgA, degree of antibody class switching (IgG/IgM),Citation95 and antigen-specific serum IgGCitation98 as potential CoP for UTI. In summary, although no universal ExPEC vaccine has been established, the different formulations of ExPEC vaccines to date seem to induce humoral antibody response in human and animal subjects as CoP.
3.2. Bladder TH polarization
In response to intracellular invasion of UPEC, the bladder sheds luminal epithelial cells as part of its innate immune response, a phenomenon termed exfoliation.Citation112–114 Exfoliation is a double-edged sword because although it rapidly clears infected cells, it further exposes the deeper and less mature urothelium to invasion, leading to quiescent intracellular reservoirs and recurrent infections.Citation115 This pathogenic mechanism requires a delicate balance of immune responses to clear intracellular bacteria while maintaining the barrier integrity of the epithelium. Recent research indicates that bladder TH1 immune responses improve UPEC clearance, especially during reinfections.Citation116 Nevertheless, some evidence suggests that the bladder launches TH2-biased immunity in both primary and secondary infections to restore epithelial integrity after exfoliation.Citation116 Therefore, designing a UPEC vaccine that stimulates the TH1 compartment may improve efficacy. For example, in a murine model of UTI, the addition of TH1-polarizing adjuvants CpG and IL-12 to lysate or FimH-based vaccines significantly promoted TH1 responses and decreased bladder colonization compared to antigen-only vaccines ().Citation117 Importantly, the TH2 tissue repair response remained unaffected by these adjuvants.Citation117 Taken together, these new findings suggest that reprograming CD4+ immunity in the bladder through the use of adjuvants may be a promising approach to enhance ExPEC vaccine efficacy.
3.3. T cell-mediated immune memory
Immune memory is a fundamental part of vaccines: the immune system launches a more rapid and vigorous attack at a pathogen that it has encountered before, thus protecting the host from re-infections.Citation118 Although immune memory develops in response to UPEC infections, it fails to induce sterilizing immunity, which partially contributes to UTI recurrence.Citation119 An effective vaccine may achieve sterilizing immunity by amplifying the protective memory response. A recent study showed that, in a mouse model of recurrent UTI, bladder tissue-resident memory T cells (Trm) are both necessary and sufficient for developing immune memory.Citation120 Interestingly, the authors found no TH subset cell skewing among CD4+ T cells in bladder and draining lymph nodes post infection. Instead, a mixture of TH1, TH2, TH17, and Treg cells was detected over 7 days of infection.Citation120 It remains unclear how each of these TH subsets participate in the formation of the Trm reservoir, an area worth future investigation.Citation120 Additionally, mice that lack mature B cells achieved a level of bacterial clearance comparable to wild-type mice during reinfection, suggesting that B cells are dispensable for UTI memory.Citation120 Another mouse study also pointed out that TH1 cells instead of serum IgG are responsible for the increased bacterial clearance induced by vaccination.Citation117 As most past vaccine studies have considered B cell antibody response as a hallmark for protection, these findings question the paradigm and highlight the protective role of T cells in immune memory. Although it remains unknown how vaccine immune response can be programmed toward the production of Trm cells,Citation120 more research on cellular memory response will provide insights into a better vaccine design.
3.4. Mucosal vaccination
The delivery method of a vaccine plays an important role in governing the type of immune response elicited (). Since the urogenital tract is the most common infection site for ExPEC,Citation4 there have been many attempts in developing mucosal vaccines to induce strong humoral and cellular responses at this site. Although all currently licensed human mucosal vaccines are based on live-attenuated or whole-cell components,Citation121 this approach has not been successful for ExPEC vaccines. In a randomized, placebo-controlled phase II clinical trial, the whole-cell vaccine SolcoUrovac was administered as vaginal suppositories in women with recurrent UTI.Citation25–27 However, the vaccine was not universally immunogenic despite causing an initial delay to reinfection. Another whole-cell vaccine taken as oral tablets also induced poor immune response: increase in saliva antibody titers were observed in <50% patients.Citation122 More recently, researchers explored intranasal and intravesical subunit vaccines with cholera toxin or other adjuvants for UPEC.Citation95,Citation98,Citation101,Citation123 These vaccines showed promising immunogenicity and efficacy in animal models, though their effect in human remains unknown. Together, these past vaccine attempts suggest that, when delivered mucosally, whole-cell antigens may not be sufficient to elicit strong and functional immune response, whereas adjuvanted subunit vaccines are a more promising solution. Novel mucosal vaccine designs for other pathogens may shed light into how ExPEC vaccines can be improved. For example, the enterotoxigenic E. coli vaccine ETVAX utilizes inactivated whole-cell bacteria to overexpress colonization antigens on cell surfaces.Citation124 ETVAX in combination with the mucosal adjuvant dmLT induced strong mucosal antibody responses in both mice and human subjects.Citation124,Citation125 The combination of whole cells, targeted antigens, and adjuvants allows the vaccine to be highly immunogenic and may be useful for designing ExPEC vaccines as well.
3.5. The aging disease population
Older adults are at a higher risk for ExPEC infections: 1) the majority of patients hospitalized for ExPEC-caused bacteremia are aged >65 yearsCitation126,Citation127; 2) UTI occurrence in women doubles in those >65 years compared to the overall populationCitation128; 3) for men 65–74 years of age, the incidence rate of UTI increases fivefold when compared with men in their 20s.Citation129 One major reason for the increased susceptibility to infection in older adults is immunosenescence, which refers to the functional decline of the immune system as part of the natural aging process.Citation130 Characteristics of immunosenescence include decreased production of naïve lymphocytes, increased accumulation of terminally differentiated memory cells, and declines in antibody quantity and qualityCitation131–133 (). In addition to poor response to various infections, immunosenescence also leads to impaired vaccine responses. For example, studies of influenza vaccines have shown that older adults have a lower seroconversion rate,Citation134 decreased vaccine-specific neutralizing antibody level,Citation135 and declined CD4+ and CD8+ T cell expansionCitation136 after vaccination compared to younger adults. The efficacy of herpes zoster vaccine in people over 60 years old also decreases rapidly from 68% in the first year to merely 4% in the eighth year,Citation137 which is likely due to age-related waning of cellular immunity. Considering the dramatic impact of aging on vaccine responses, it is therefore imperative to tailor ExPEC vaccine design to the needs of this target population. In other vaccine formulations designed for older adults, the addition of adjuvants is useful for boosting immune responses. For instance, the influenza vaccine FLUAD™ formulated with the adjuvant MF59® has significantly improved vaccine effectiveness compared to non-adjuvanted formulations in adults larger than or equal to 65 years old.Citation138 Similarly, the herpes zoster vaccine Shingrix™ containing the AS01B adjuvant confers protection in over 96% vaccinated older adultsCitation139 and elicits virus-specific antibodies that persists for at least 9 years.Citation140 So far, ExPEC vaccine studies have been focused on women or adults in general, while studies that recruit older adults are lacking, with ExPEC9V (NCT04899336) being the only clinical trial conducted solely in older adults. Understanding what immune correlates are weakened in ExPEC-infected older adults and testing different adjuvant and antigen combinations stand as unexplored area of ExPEC vaccine research.
4. New vaccine technologies for ExPEC
Other than traditional vaccine approaches and delivery methods (), novel vaccine technologies have been explored by researchers to improve ExPEC vaccine immunogenicity and efficacy. Here, we focus on 3 of the new vaccine strategies that have improved upon ExPEC vaccination in preclinical models: encapsulated vaccines, nanofiber vaccines, and DNA vaccines. For a full overview of these emerging vaccine technologies, we refer to the following dedicated reviews on the subject.Citation141,Citation142
4.1. Encapsulated vaccines
During the course of infection, the immune system is stimulated with microbial antigens for an extended period of time. However, traditional non-live vaccines deliver antigens in single injections. This has prompted vaccine research to focus on antigen kinetics, specifically the slow or extended delivery of antigens to more closely mimic natural infections.Citation143 Prolonged vaccine antigen availability can boost germinal center formation in draining lymph nodes and improve antibody responses by increasing the abundance of neutralizing antibodies and T follicular helper cells.Citation143–145 Encapsulation of whole cell and subunit antigens is one such way that creates a depot effect to effectively control antigen kinetics.Citation146 Common methods of encapsulation include liposomes, virosomes, nanoparticles, and microspheres.Citation146
A recent study demonstrated better immunogenicity of nanoparticle-encapsulated subunit vaccines against UPEC in a mouse model.Citation147 Here, the researchers developed 2 vaccines, each containing B- or T-cell epitopes of previously explored antigens FdeC (adherence factor), Hma (adhesion autotransporter), and UpaB (iron receptor) with the cholera toxin subunit B adjuvant, encapsulated in chitosan nanoparticles.Citation147 When intranasally delivered to mice, the B cell construct generated high levels of IgG1 and IL-4 characteristic of TH2 response, while the T cell construct elicited high levels of IgG2a and IFNγ characteristic of TH1 response.Citation147 Although mice immunized with either nanoparticle coated or non-coated vaccines were protected from UPEC colonization in bladder, the coated vaccines produced significantly stronger antibody- and cell-mediated immune responses.Citation147
Another useful trait of nanoparticle encapsulation is providing stability for subunit antigens. For instance, OmpAVac, a recombinant vaccine based on outer membrane protein A of the neonatal bacterial meningitis-causing strain E. coli K1, induced TH1, TH2, and TH17 responses and showed effective protection in mice,Citation148 but was impeded from downstream applications due to poor in vitro and in vivo stability.Citation149 By coating OmpAVac in chitosan-modified poly (lactic-co-glycolic acid) (PLGA) nanoparticles, researchers demonstrated that not only are the antigens more slowly released, but they also preserved immune protection in mice after 180 days of storage.Citation149
Since classic vaccine encapsulation vehicles work better with small proteins and antigens, additional technologies are required for larger cargos such as whole bacterial cells.Citation150 In one study, a biomimetic network called Zeolitic Imidazolate Framework (ZIF) was used to encapsulate and inactivate the urosepsis strain CFT073 as a whole-cell vaccine.Citation150 Similar to nanoparticles, ZIF slowed the dissipation of vaccine, which remained at the injection site in mice for 4 days longer than the uncoated version.Citation150 Mice immunized with ZIF-coated CFT073 exhibited significant increases in humoral response (anti-CFT073 IgG1, IgG2a and IgG2b), cellular response (CD3+, CD4+, CD8+ cell numbers in the spleen), and cytokine response (TNF-α, IFN-γ, IL-4, and IL-17).Citation150 In a mouse model of urosepsis, the ZIF-encapsulated bacteria vaccine protected over 85% of vaccinated mice, while less than 20% animals survived in the non-encapsulated vaccine group.Citation150 Unlike standard whole-cell inactivation methods, ZIF encapsulation preserved the native structures of CFT073 surface epitopes,Citation150 which may be another explanation for the superior immunogenicity and efficacy of this vaccine.
4.2. Nanofiber vaccines
Similar to encapsulated vaccines, nanofiber vaccine is another approach that utilizes biomaterials to engineer antigen delivery. This vaccine platform allows the supramolecular co-assembly of selected epitopes with Q11 nanofibers conjugated with polyethylene glycol (PEG), a hydrophilic polymer.Citation151 The supramolecular assembly has been shown to be superior in immunogenicity compared to PEGylated peptides directly delivered as vaccines.Citation151 Additionally, the mucus-penetrating ability of PEG-modified nanofibers is ideal for delivering mucosal vaccines.Citation151,Citation152 Recently, one research group developed a sublingual nanofiber vaccine effective against UPEC strain CFT073 in mice.Citation152 In this vaccine, UPEC B cell epitopes from siderophore receptors (IreA, lutA, IroN) and helper T cell epitopes (VAC or PADRE) are supramolecularly co-assembled with PEGylated Q11 nanofibers.Citation152 After mice were immunized with this nanofiber vaccine, strong UPEC-specific antibody response was observed both in serum and urine. The vaccine demonstrated great efficacy in protecting mice from urosepsis and in reducing UTI.Citation152 Notably, vaccination had minimal impact on the microbiome, especially when compared to mice treated with antibiotics.Citation152 Despite these positive results, this study did not include a group that receives non-assembled vaccine, which would be a critical control group that indicates the immunogenic benefit of this novel vaccine technology.
4.3. DNA vaccines
DNA-based vaccines are an increasingly attractive platform because of improved safety compared to live vaccines and induction of both humoral and cellular immunity.Citation153 Specifically, DNA vaccines were shown to stimulate cytotoxic T lymphocyte production, which is essential for the elimination of intracellular pathogens.Citation153,Citation154 In general, DNA vaccines work by utilizing host cellular machinery to produce the antigens of interest endogenously, which then get presented to stimulate adaptive immunity.Citation153 Since UPEC is a facultative intracellular pathogen, developing DNA vaccines have the potential to overcome current challenges including failure to induce cellular responses and prevent recurrent infections. One group developed UPEC FimH-based vector constructs as DNA vaccines.Citation154 In this study, mice immunized with two doses of the DNA vaccine displayed heightened IFN-γ, IL-12, and IL-17 levels compared to animals in the control and protein vaccine groups.Citation154 These mice also had significantly higher UPEC clearance from bladder post-challenge than the control animals.Citation154 A later study employed a similar vaccine construct design based on the iron receptor IutA.Citation155 Although vaccine efficacy was not tested in this study, mice that received the DNA vaccine had increased IFN-γ level than control mice, indicating TH1 polarization.Citation155
5. Evolutionary considerations for E. coli vaccine development
While much of this review has focused on identifying antigens and immune responses to protect against ExPEC, it also must be considered that E. coli is a remarkably diverse organism with the potential to promote health as well as to cause devastating disease. The line that separates these commensal and pathogenic lifestyles can be difficult to determine, and indeed even pathogenic strains colonize some people without causing disease. Because the goal of vaccination is to promote protection against infection without compromising the health of the host, the challenge of creating a vaccine against ExPEC is in targeting pathogenic E. coli while leaving its commensal relatives unperturbed. This requires a closer look at the phylogenetics of the organism.
E. coli is thought to have evolved from an ancestral microbe into what are now recognized to be 8 genetically distinct phylogroups: A, B1, B2, C, D, E, F, and G. Broadly speaking, these phylogroups can be resolved into two main clusters: one containing phylogroups B2, D, F, and G, and the other containing A, B1, C, and E.Citation156 This clustering closely mirrors the type of polysaccharide capsule each phylogroup carries.Citation63 The A/B1/C/E branch, which is associated with the intestinal lifestyle, almost always (>97%) carries the group 4 capsule (G4C).Citation63,Citation157 By contrast, the B2/D/F branch almost always (>90%) carries the group 2 capsule (G2C) and is associated with extraintestinal pathogenicity. The only exception here is the minor G phylogroup, which is closely related to B2 strains but whose ancestor may have acquired G4C genes independently. The G2C likely enabled the B2/D/F cluster’s divergence into a more distally invasive phenotype since it mimics host sugar structures and thus cloaks the bacterium from the host immune system.Citation63,Citation157 Indeed, cross-reactivity with host ganglioside sugars has been reported after murine vaccination with the G2C K1 antigen,Citation158 although in vivo evidence of autoreactivity is lacking. This may help explain the observation that anti-K antibodies are less commonly induced by ExPEC infection.Citation37 Thus, despite being enriched in pathogenic strains, G2C antigens may not represent optimal vaccine candidates.
While a strain’s preferred niche tends to be determined by the type of capsule it carries, its virulence factors (VFs) and virulence potential is determined by its phylogroup.Citation63,Citation156,Citation159 Each of the 8 phylogroups have a distinct set of VFs and a propensity to cause disease, although each phylogroup also includes commensals.Citation63,Citation156,Citation159 Broadly speaking, phylogroups A and B1 are more likely to follow a commensal lifestyle, while B2 and D follow a more pathogenic lifestyle in humans.Citation156,Citation159 The C phylogroup, on the other hand, often causes infections in avians (APEC) but rarely causes disease in humans.Citation160 The E phylogroup is considered a minor phylogroup because it is less prevalent than major phylogroups. However, it is still of concern because many diarrheal strains including the famed O157:H7 serotype, which causes deadly EHEC outbreaks, belong to this phylogroup.Citation161 Less is known about phylogroups F and G, since these appear to be rarer and have only been discovered recently.Citation162,Citation163 Nonetheless, some phylogroup F and G sequence types are known to cause ExPEC infection.Citation63,Citation156,Citation163
The most common phylogroup implicated in ExPEC infections is by far the B2 phylogroup.Citation164 Clinically, B2 strains are the largest E. coli contributors to UTIs, septicemia, and neonatal meningitis. ExPEC strains of the well-known pandemic ST131 sequence type belong to the B2 phylogroup, as well as UPEC and NMEC-associated sequence types.Citation63,Citation156,Citation165–168 This makes B2 an attractive phylogroup for study in vaccinology. Indeed, all B2 strains carry many of the adherence factors, toxins, and protectins associated with infections outside the intestines. However, many apparently commensal strains also express these factors. As mentioned previously, some groups have applied genomics to deconvolute VFs that are highly conserved among pathogenic strains but minimally encoded among commensals.Citation61–63 However, the high prevalence of VFs among the B2 lineage makes it difficult to classify strains as pathogenic or commensal using genomic analysis alone.Citation63 For example, the ST131 strain SE15 appears to be a harmless commensal, but has lost only four VFs when compared to virulent members of the same fimH41 subtype.Citation63,Citation169 Another example is the well-known E. coli probiotic strain, Nissle 1917, which belongs to the normally highly pathogenic and VF-laden sequence-type ST73.Citation170–172 It has apparently lost only six VFs compared to closely related virulent strains.Citation63,Citation173 Without detailed virulence information about closely related strains, both SE15 and Nissle 1917 would likely be predicted as pathogenic based on the VFs they retain. This suggests that any successful method to classify strains as either commensal or pathogenic will need to consider clonal-level information about VFs.
Phylogroup D is also associated with ExPEC pathotypes, and it is the second most isolated phylogroup in this category behind B2 phylogroup.Citation156 When found to be pathogenic, strains from the D lineage are usually either members of InPEC or UPEC pathotypes.Citation156,Citation174 Phylogroup D strains can exist as harmless commensals, but like B2 strains, their pathogenicity can be difficult to predict using just genomic analysis because even commensals often carry proteins known to impact extraintestinal virulence.Citation63
In , a heatmap that cross-references a database of 396 known virulence genes against 1,348 complete E. coli genomes shows the diversity of the E. coli pan-virulome. The diversity highlighted here makes it difficult to find vaccine targets with broad efficacy against multiple types of pathogenic E. coli without having off-target effects on commensal E. coli. Some clear trends can be seen between the phylogroups associated with ExPEC infections (B2, D, and sometimes F) compared to phylogroups that are considered more commensal-like (A and B1). The former group has an overabundance of iron acquisition genes, capsule genes, fimbriae, autotransporters, and toxins (). However, many of these genes have low conservation and low prevalence among ExPEC strains, making them ineligible as potential vaccine targets. For the rational design of an ExPEC vaccine, it is crucial for researchers to screen through not only the genome, but transcriptome and proteome for ExPEC-enriched targets that are absent or less abundant in commensal E. coli (Computational Approaches, ). This approach, combined with in vitro experiments and animal studies, will be promising for developing an effective and specific vaccine.
Figure 5. Pan-Virulome of Escherichia coli.
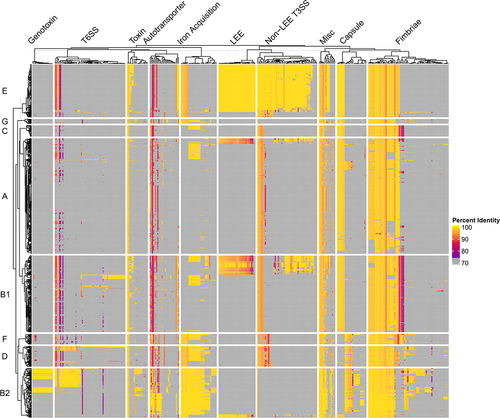
6. Concluding remarks
Despite nearly four decades of research since the initial ExPEC vaccine trial,Citation13 an FDA-approved vaccine for ExPEC remains elusive. Developing an effective vaccine has been challenging due to several intrinsic characteristics of the organism, including: (1) extensive strain diversity, (2) functional redundancy of virulence factors, (3) challenges in differentiating commensal and pathogenic strains, (4) intracellular reservoirs, and (5) immune resistance conferred by polysaccharide capsules and other mechanisms. Notwithstanding, advancements in vaccine technology and an improved understanding of the immunologic response to ExPEC infection may help overcome these challenges. For example, the basic vaccine development approach for ExPEC has shifted from traditional whole-cell vaccine formulations toward subunit vaccines that target specific antigens. This shift has been fuelled by expanded serotype surveillanceCitation56 and the power of genomics for identifying conserved virulence factors.Citation63 The subunit approach is currently being evaluated in two vaccine candidates undergoing clinical trials: the ExPEC9V vaccine currently in Phase III trials (NCT04899336) and the FimH vaccine planned for Phase II.Citation77 Further preclinical research has yielded significant strides in optimizing subunit vaccine immunogenicity and selectively activating immune compartments that confer protection. In light of this progress, this review aimed to synthesize the current body of literature on ExPEC vaccine development and to critically evaluate the long history of ExPEC vaccine trials.
Acknowledgments
All figures except were created with Biorender.com.
Disclosure statement
No potential conflict of interest was reported by the author(s).
Data availability statement
Data sharing is not applicable to this manuscript because no new data was created.
Additional information
Funding
References
- Rojas-Lopez M, Monterio R, Pizza M, Desvaux M, Rosini R. Intestinal pathogenic Escherichia coli: insights for vaccine development. Front Microbiol. 2018;9:440. doi:10.3389/fmicb.2018.00440.
- Poolman JT, Wacker M. Extraintestinal pathogenic Escherichia coli, a common human pathogen: challenges for vaccine development and progress in the field. J Infect Dis. 2016;213(1):6–25. doi:10.1093/infdis/jiv429.
- Dale AP, Woodford N. Extra-intestinal pathogenic Escherichia coli (ExPEC): disease, carriage and clones. J Infect. 2015;71(6):615–626. doi:10.1016/j.jinf.2015.09.009.
- Russo TA, Johnson JR. Medical and economic impact of extraintestinal infections due to Escherichia coli: focus on an increasingly important endemic problem. Microbes Infect. 2003;5(5):449–456. doi:10.1016/S1286-4579(03)00049-2.
- Foxman B, Brown P. Epidemiology of urinary tract infections: transmission and risk factors, incidence, and costs. Infect Dis Clin North Am. 2003;17(2):227–241. doi:10.1016/S0891-5520(03)00005-9.
- Klein RD, Hultgren SJ. Urinary tract infections: microbial pathogenesis, host–pathogen interactions and new treatment strategies. Nat Rev Microbiol. 2020;18(4):211–226. doi:10.1038/s41579-020-0324-0.
- Al-Qassimi MA, Al Amad M, Anam L, Almoayed K, Al-Dar A, Ezzadeen F. Circulating vaccine derived polio virus type 1 outbreak, Saadah governorate, Yemen, 2020. BMC Infect Dis. 2022;22(1):414. doi:10.1186/s12879-022-07397-0.
- Billips BK, Yaggie RE, Cashy JP, Schaeffer AJ, Klumpp DJ. A live-attenuated vaccine for the treatment of urinary tract infection by uropathogenic Escherichia coli. J Infect Dis. 2009;200(2):263–272. doi:10.1086/599839.
- Russo TA, Beanan JM, Olson R, Genagon SA, MacDonald U, Cope JJ, Davidson BA, Johnston B, Johnson JR. A killed, genetically engineered derivative of a wild-type extraintestinal pathogenic E. coli strain is a vaccine candidate. Vaccine. 2007;25(19):3859–3870. doi:10.1016/j.vaccine.2007.01.100.
- Russo TA, Beanan JM, Olson R, MacDonald U, Cope JJ. Capsular polysaccharide and the O-specific antigen impede antibody binding: a potential obstacle for the successful development of an extraintestinal pathogenic Escherichia coli vaccine. Vaccine. 2009;27(3):388–395. doi:10.1016/j.vaccine.2008.10.082.
- Magistro G, Stief CG. Vaccine development for urinary tract infections: where do we stand? European Urology Focus. 2019;5(1):39–41. doi:10.1016/j.euf.2018.07.034.
- Hachen HJ. Oral immunotherapy in paraplegic patients with chronic urinary tract infections: a double-blind, placebo-controlled trial. J Urol. 1990;143(4):759–762. discussion 762-763. doi:10.1016/s0022-5347(17)40084-x.
- Frey C, Obolensky W, Wyss H. Treatment of recurrent urinary tract infections: efficacy of an orally administered biological response modifier. Urol Int. 1986;41(6):444–446. doi:10.1159/000281253.
- Wade DT, Cooper J, Peckham N, Belci M. Immunotherapy to reduce frequency of urinary tract infections in people with neurogenic bladder dysfunction; a pilot randomised, placebo-controlled trial. Clin Rehabil. 2020;34(12):1458–1464. doi:10.1177/0269215520946065.
- Kim KS, Kim JY, Jeong IG, Paick J-S, Son H, Lim DJ, Shim HB, Park WH, Jung HC, Choo M-S. et al. A prospective multi-center trial of Escherichia coli extract for the prophylactic treatment of patients with chronically recurrent cystitis. J Korean Med Sci. 2010;25(3):435–439. doi:10.3346/jkms.2010.25.3.435.
- Bauer HW, Alloussi S, Egger G, Blümlein H-M, Cozma G, Schulman CC. A long-term, multicenter, double-blind study of an Escherichia coli extract (OM-89) in female patients with recurrent urinary tract infections. Eur Urol. 2005;47(4):542–548. discussion 548. doi:10.1016/j.eururo.2004.12.009.
- Magasi P, Pánovics J, Illés A, Nagy M. Uro-Vaxom and the management of recurrent urinary tract infection in adults: a randomized multicenter double-blind trial. Eur Urol. 1994;26(2):137–140. doi:10.1159/000475363.
- Tammen H. Immunobiotherapy with Uro-Vaxom in recurrent urinary tract infection. The German urinary tract infection study group. Br J Urol. 1990;65(1):6–9. doi:10.1111/j.1464-410x.1990.tb14649.x.
- Schulman CC, Corbusier A, Michiels H, Taenzer HJ. Oral immunotherapy of recurrent urinary tract infections: a double-blind placebo-controlled multicenter study. J Urol. 1993;150(3):917–921. doi:10.1016/s0022-5347(17)35648-3.
- Krebs J, Stoyanov J, Wöllner J, Valido E, Pannek J. Immunomodulation for primary prevention of urinary tract infections in patients with spinal cord injury during primary rehabilitation: protocol for a randomized placebo-controlled pilot trial (UROVAXOM-pilot). Trials. 2021;22(1):677. doi:10.1186/s13063-021-05630-w.
- Krebs J, Fleischli S, Stoyanov J, Pannek J. Effects of oral immunomodulation therapy on urinary tract infections in individuals with chronic spinal cord injury-A retrospective cohort study. Neurourol Urodyn. 2019;38(1):346–352. doi:10.1002/nau.23859.
- Hopkins WJ, Elkahwaji J, Beierle LM, Leverson GE, Uehling DT. Vaginal mucosal vaccine for recurrent urinary tract infections in women: results of a phase 2 clinical trial. J Urol. 2007;177(4):1349–1353. doi:10.1016/j.juro.2006.11.093.
- Grischke EM, Rüttgers H. Treatment of bacterial infections of the female urinary tract by immunization of the patients. Urol Int. 1987;42(5):338–341. doi:10.1159/000281988.
- Uehling DT, Hopkins WH, Dahmer LA, Balish E. Phase I clinical trial of vaginal mucosal immunization for recurrent urinary tract infection. J Urol. 1994;152(6 Part 2):2308–2311. doi:10.1016/S0022-5347(17)31664-6.
- Uehling DT, Hopkins WJ, Beierle LM, Kryger JV, Heisey DM. Vaginal mucosal immunization for recurrent urinary tract infection: extended phase II clinical trial. J Infect Dis. 2001;183(Supplement 1):S81–S83. doi:10.1086/318839.
- Uehling DT, Hopkins WJ, Elkahwaji JE, Schmidt DM, Leverson GE. Phase 2 clinical trial of a vaginal mucosal vaccine for urinary tract infections. J Urol. 2003;170(3):867–869. doi:10.1097/01.ju.0000075094.54767.6e.
- Uehling DT, Hopkins WJ, Balish E, Xing Y, Heisey DM. Vaginal mucosal immunization for recurrent urinary tract infection: phase II clinical trial. J Urol. 1997;157(6):2049–2052. doi:10.1016/S0022-5347(01)64671-8.
- Nayir A, Emre S, Sirin A, Bulut A, Alpay H, Tanman F. The effects of vaccination with inactivated uropathogenic bacteria in recurrent urinary tract infections of children. Vaccine. 1995;13(11):987–990. doi:10.1016/0264-410x(95)00022-s.
- Nestler S, Peschel C, Horstmann AH, Vahlensieck W, Fabry W, Neisius A. Prospective multicentre randomized double-blind placebo-controlled parallel group study on the efficacy and tolerability of StroVac® in patients with recurrent symptomatic uncomplicated bacterial urinary tract infections. Int Urol Nephrol. 2023;55(1):9–16. doi:10.1007/s11255-022-03379-y.
- Lorenzo-Gómez MF, Padilla-Fernández B, García-Criado FJ, Mirón-Canelo JA, Gil-Vicente A, Nieto-Huertos A, Silva-Abuin JM. Evaluation of a therapeutic vaccine for the prevention of recurrent urinary tract infections versus prophylactic treatment with antibiotics. Int Urogynecol J. 2013;24(1):127–134. doi:10.1007/s00192-012-1853-5.
- Lorenzo-Gómez MF, Padilla-Fernández B, García-Cenador MB, Virseda-RodrÃguez ÃJ, MartÃn-GarcÃa I, Sánchez-Escudero A, Vicente-Arroyo MJ, Mirón-Canelo JA. Comparison of sublingual therapeutic vaccine with antibiotics for the prophylaxis of recurrent urinary tract infections. Front Cell Infect Microbiol. 2015;5:50. doi:10.3389/fcimb.2015.00050.
- Yang B, Foley S. First experience in the UK of treating women with recurrent urinary tract infections with the bacterial vaccine Uromune®. BJU Int. 2018;121(2):289–292. doi:10.1111/bju.14067.
- Lorenzo-Gómez MF, Foley S, Nickel JC, García-Cenador M-B, Padilla-Fernández B-Y, González-Casado I, Martínez-Huélamo M, Yang B, Blick C, Ferreira F. et al. Sublingual MV140 for prevention of recurrent urinary tract infections. NEJM Evid. 2022;1(4). doi:10.1056/EVIDoa2100018.
- Benito-Villalvilla C, Cirauqui C, Diez-Rivero CM, Casanovas M, Subiza JL, Palomares O. MV140, a sublingual polyvalent bacterial preparation to treat recurrent urinary tract infections, licenses human dendritic cells for generating Th1, Th17, and IL-10 responses via Syk and MyD88. Mucosal Immunol. 2017;10(4):924–935. doi:10.1038/mi.2016.112.
- Liu B, Furevi A, Perepelov AV, Guo X, Cao H, Wang Q, Reeves PR, Knirel YA, Wang L, Widmalm G. et al. Structure and genetics of Escherichia coli O antigens. FEMS Microbiol Rev. 2020;44(6):655–683. doi:10.1093/femsre/fuz028.
- Whitfield C. Structure and assembly of Escherichia coli capsules. EcoSal Plus. 2009;3(2). doi:10.1128/ecosalplus.4.7.3.
- Kaijser B, Jodal U, Hanson LÅ. Studies on antibody response and tolerance to E. coli K antigens in immunized rabbits and in children with urinary tract infection. Int Arch Allergy Immunol. 2009;44(2):260–273. doi:10.1159/000230935.
- Henriksen AZ, Maeland JA. Serum antibodies to outer membrane proteins of Escherichia coli in healthy persons and patients with bacteremia. J Clin Microbiol. 1987;25(11):2181–2188. doi:10.1128/jcm.25.11.2181-2188.1987.
- Kaijser B, Ahlstedt S. Protective capacity of antibodies against Escherichia coli and K antigens. Infect Immun. 1977;17(2):286–289. doi:10.1128/iai.17.2.286-289.1977.
- Kaijser B. Studies on the K antibody response in rabbits immunized with a pool of five different K antigen-containing Escherichia coli. Int Arch Allergy Appl Immunol. 1981;65(3):300–303. doi:10.1159/000232769.
- Straube E, Nimmich W, Broschewitz U, Naumann G, Hacker K. Effect of immunization with Escherichia coli K1 antigen on the course of experimental infection of the urinary tract of the rat. Zentralblatt für Bakteriologie, Mikrobiologie und Hygiene Series A: Med Microbiol, Infect Dis, Virol, Parasitol. 1987;265(3):408–419. doi:10.1016/S0176-6724(87)80260-2.
- Kaijser B, Larsson P, Schneerson R. Protection against acute, ascending pyelonephritis caused by E.Coli in rats using isolated capsular antigen conjugated to a carrier substance. Pediatr Res. 1980;14(8):980–980. doi:10.1203/00006450-198008000-00040.
- Chorro L, Li Z, Chu L, Singh S, Gu J, Kim J-H, Dutta K, Pan R, Kodali S, Ndreu D. et al. Preclinical immunogenicity and efficacy of optimized O25b O-Antigen glycoconjugates to prevent MDR ST131 E. coli infections. Infect Immun. 2022;90(4):e00022–22. doi:10.1128/iai.00022-22.
- Naini A, Bartetzko MP, Sanapala SR, Broecker F, Wirtz V, Lisboa MP, Parameswarappa SG, Knopp D, Przygodda J, Hakelberg M. et al. Semisynthetic glycoconjugate vaccine candidates against Escherichia coli O25B induce functional IgG antibodies in mice. JACS Au. 2022;2(9):2135–2151. doi:10.1021/jacsau.2c00401.
- Kowarik M, Wetter M, Haeuptle MA, Braun M, Steffen M, Kemmler S, Ravenscroft N, De Benedetto G, Zuppiger M, Sirena D. et al. The development and characterization of an E. coli O25B bioconjugate vaccine. Glycoconj J. 2021;38(4):421–435. doi:10.1007/s10719-021-09985-9.
- Cryz SJ Jr, Cross AS, Sadoff JC, Wegmann A, Que JU, Fürer E. Safety and immunogenicity of Escherichia coli 018 O-Specific polysaccharide (O-PS)-toxin a and O-PS-cholera toxin conjugate vaccines in humans. Journal Of Infectious Diseases. 1991;163(5):1040–1045. doi:10.1093/infdis/163.5.1040.
- Cross A, Artenstein A, Que J, Fredeking T, Furer E, Sadoff JC, Cryz SJ Jr. Safety and immunogenicity of a polyvalent Escherichia coli vaccine in human volunteers. J Infect Dis. 1994;170(4):834–840. doi:10.1093/infdis/170.4.834.
- Cross AS, Opal SM, Palardy JE, Drabick JJ, Warren HS, Huber C, Cook P, Bhattacharjee AK. Phase I study of detoxified Escherichia coli J5 lipopolysaccharide (J5dLPS)/group B meningococcal outer membrane protein (OMP) complex vaccine in human subjects. Vaccine. 2003;21(31):4576–4587. doi:10.1016/s0264-410x(03)00483-3.
- Cross AS, Greenberg N, Billington M, Zhang L, DeFilippi C, May RC, Bajwa KK. Phase 1 testing of detoxified LPS/group B meningococcal outer membrane protein vaccine with and without synthetic CPG 7909 adjuvant for the prevention and treatment of sepsis. Vaccine. 2015;33(48):6719–6726. doi:10.1016/j.vaccine.2015.10.072.
- van den Dobbelsteen GPJM, Faé KC, Serroyen J, van den Nieuwenhof IM, Braun M, Haeuptle MA, Sirena D, Schneider J, Alaimo C, Lipowsky G. et al. Immunogenicity and safety of a tetravalent E. coli O-antigen bioconjugate vaccine in animal models. Vaccine. 2016;34(35):4152–4160. doi:10.1016/j.vaccine.2016.06.067.
- Inoue M, Ogawa T, Tamura H, Hagiwara Y, Saito Y, Abbanat D, van den Dobbelsteen G, Hermans P, Thoelen S, Poolman J. et al. Safety, tolerability and immunogenicity of the ExPEC4V (JNJ-63871860) vaccine for prevention of invasive extraintestinal pathogenic Escherichia coli disease: A phase 1, randomized, double-blind, placebo-controlled study in healthy Japanese participants. Hum Vaccines Immunother. 2018;14(9):2150–2157. doi:10.1080/21645515.2018.1474316.
- Huttner A, Hatz C, van den DG, Abbanat D, Hornacek A, Frölich R, Dreyer AM, Martin P, Davies T, Fae K. et al. Safety, immunogenicity, and preliminary clinical efficacy of a vaccine against extraintestinal pathogenic Escherichia coli in women with a history of recurrent urinary tract infection: a randomised, single-blind, placebo-controlled phase 1b trial. Lancet Infect Dis. 2017;17(5):528–537. doi:10.1016/S1473-3099(17)30108-1.
- Frenck RW, Ervin J, Chu L, Abbanat D, Spiessens B, Go O, Haazen W, van den Dobbelsteen G, Poolman J, Thoelen S. et al. Safety and immunogenicity of a vaccine for extra-intestinal pathogenic Escherichia coli (ESTELLA): a phase 2 randomised controlled trial. Lancet Infect Dis. 2019;19(6):631–640. doi:10.1016/S1473-3099(18)30803-X.
- Fierro CA, Sarnecki M, Doua J, Spiessens B, Go O, Davies TA, van den Dobbelsteen G, Poolman J, Abbanat D, Haazen W. et al. Safety, reactogenicity, immunogenicity, and dose selection of 10-valent extraintestinal pathogenic Escherichia coli bioconjugate vaccine (VAC52416) in adults aged 60–85 years in a randomized, multicenter, interventional, first-in-human, phase 1/2a study. Open Forum Infect Dis. 2023;10(8):ofad417. doi:10.1093/ofid/ofad417.
- Huttner A, Gambillara V. The development and early clinical testing of the ExPEC4V conjugate vaccine against uropathogenic Escherichia coli. Clin Microbiol Infect. 2018;24(10):1046–1050. doi:10.1016/j.cmi.2018.05.009.
- Weerdenburg E, Davies T, Morrow B, Zomer AL, Hermans P, Go O, Spiessens B, van den Hoven T, van Geet G, Aitabi M. et al. Global distribution of O serotypes and antibiotic resistance in extraintestinal pathogenic Escherichia coli collected from the blood of patients with bacteremia across multiple surveillance studies. Clin Infect Dis. 2023;76(3):e1236–e1243. doi:10.1093/cid/ciac421.
- Lindberg U, Hanson LA, Jodal U, Lidin-Janson G, Lincoln K, Olling S. Asymptomatic bacteriuria in schoolgirls. Acta Paediatr. 1975;64(3):432–436. doi:10.1111/j.1651-2227.1975.tb03860.x.
- Blanco M, Blanco JE, Alonso MP, Blanco J. Virulence factors and O groups of Escherichia coli isolates from patients with acute pyelonephritis, cystitis and asymptomatic bacteriuria. Eur J Epidemiol. 1996;12(2):191–198. doi:10.1007/BF00145506.
- Pokharel P, Dhakal S, Dozois CM. The diversity of Escherichia coli pathotypes and vaccination strategies against this versatile bacterial pathogen. Microorganisms. 2023;11(2):344. doi:10.3390/microorganisms11020344.
- Lloyd AL, Rasko DA, Mobley HLT. Defining genomic islands and uropathogen-specific genes in uropathogenic Escherichia coli. J Bacteriol. 2007;189(9):3532–3546. doi:10.1128/JB.01744-06.
- Moriel DG, Tan L, Goh KGK, Phan M-D, Ipe DS, Lo AW, Peters KM, Ulett GC, Beatson SA, Schembri MA. et al. A novel protective vaccine antigen from the core Escherichia coli genome. mSphere. 2016;1(6):e00326–16. doi:10.1128/mSphere.00326-16.
- Soltan MA, Behairy MY, Abdelkader MS, Albogami S, Fayad E, Eid RA, Darwish KM, Elhady SS, Lotfy AM, Alaa Eldeen M. et al. In silico designing of an epitope-based vaccine against common E. coli pathotypes. Front Med. 2022;9:829467. doi:10.3389/fmed.2022.829467.
- Clark JR, Maresso AM, Monack D. Comparative pathogenomics of Escherichia coli: Polyvalent vaccine target identification through virulome analysis. Infect Immun. 2021;89(8):e0011521. doi:10.1128/IAI.00115-21.
- Xing Y, Clark JR, Chang JD, Chirman DM, Green S, Zulk JJ, Jelinski J, Patras KA, Maresso AW. Broad protective vaccination against systemic Escherichia coli with autotransporter antigens. PloS Pathog. 2023;19(2):e1011082. doi:10.1371/journal.ppat.1011082.
- Cotugno N, Ruggiero A, Santilli V, Manno EC, Rocca S, Zicari S, Amodio D, Colucci M, Rossi P, Levy O. et al. OMIC technologies and vaccine development: from the identification of vulnerable individuals to the formulation of invulnerable vaccines. J Immunol Res. 2019;2019:1–10. doi:10.1155/2019/8732191.
- Adamczyk-Poplawska M, Markowicz S, Jagusztyn-Krynicka EK. Proteomics for development of vaccine. J Proteomics. 2011;74(12):2596–2616. doi:10.1016/j.jprot.2011.01.019.
- Jumper J, Evans R, Pritzel A, Green T, Figurnov M, Ronneberger O, Tunyasuvunakool K, Bates R, Žídek A, Potapenko A. et al. Highly accurate protein structure prediction with AlphaFold. Nature. 2021;596(7873):583–589. doi:10.1038/s41586-021-03819-2.
- Reynisson B, Alvarez B, Paul S, Peters B, Nielsen M. NetMHCpan-4.1 and NetMHCIIpan-4.0: improved predictions of MHC antigen presentation by concurrent motif deconvolution and integration of MS MHC eluted ligand data. Nucleic Acids Res. 2020;48(W1):W449–W454. doi:10.1093/nar/gkaa379.
- Nielsen M, Lundegaard C, Blicher T, Lamberth K, Harndahl M, Justesen S, Røder G, Peters B, Sette A, Lund O. et al. NetMHCpan, a method for quantitative predictions of peptide binding to any HLA-A and -B locus protein of known sequence. PLOS ONE. 2007;2(8):e796. doi:10.1371/journal.pone.0000796.
- Fonseca AF, Antunes DA. CrossDome: an interactive R package to predict cross-reactivity risk using immunopeptidomics databases. Front Immunol. 2023;14:1142573. doi:10.3389/fimmu.2023.1142573.
- Wieser A, Romann E, Magistro G, Hoffmann C, Nörenberg D, Weinert K, Schubert S. A multiepitope subunit vaccine conveys protection against extraintestinal pathogenic Escherichia coli in mice. Infect Immun. 2010;78(8):3432–3442. doi:10.1128/iai.00174-10.
- Hasanzadeh S, Habibi M, Shokrgozar MA, Ahangari Cohan R, Ahmadi K, Asadi Karam MR, Bouzari S. In silico analysis and in vivo assessment of a novel epitope-based vaccine candidate against uropathogenic Escherichia coli. Sci Rep. 2020;10(1):16258. doi:10.1038/s41598-020-73179-w.
- Wu XR, Sun TT, Medina JJ. In vitro binding of type 1-fimbriated Escherichia coli to uroplakins Ia and Ib: relation to urinary tract infections. Proc Natl Acad Sci. 1996;93(18):9630–9635. doi:10.1073/pnas.93.18.9630.
- Langermann S, Palaszynski S, Barnhart M, Auguste G, Pinkner JS, Burlein J, Barren P, Koenig S, Leath S, Jones CH. et al. Prevention of mucosal Escherichia coli infection by FimH-adhesin-based systemic vaccination. Science. 1997;276(5312):607–611. doi:10.1126/science.276.5312.607.
- Asadi Karam MR, Habibi M, Bouzari S. Use of flagellin and cholera toxin as adjuvants in intranasal vaccination of mice to enhance protective immune responses against uropathogenic Escherichia coli antigens. Biologicals. 2016;44(5):378–386. doi:10.1016/j.biologicals.2016.06.006.
- Asadi Karam MR, Oloomi M, Mahdavi M, Habibi M, Bouzari S. Vaccination with recombinant FimH fused with flagellin enhances cellular and humoral immunity against urinary tract infection in mice. Vaccine. 2013;31(8):1210–1216. doi:10.1016/j.vaccine.2012.12.059.
- Karam MRA, Oloomi M, Mahdavi M, Habibi M, Bouzari S. Assessment of immune responses of the flagellin (FliC) fused to FimH adhesin of Uropathogenic Escherichia coli. Mol Immunol. 2013;54(1):32–39. doi:10.1016/j.molimm.2012.11.002.
- Savar NS, Jahanian-Najafabadi A, Mahdavi M, Shokrgozar MA, Jafari A, Bouzari S. In silico and in vivo studies of truncated forms of flagellin (FliC) of enteroaggregative Escherichia coli fused to FimH from uropathogenic Escherichia coli as a vaccine candidate against urinary tract infections. J Biotechnol. 2014;175:31–37. doi:10.1016/j.jbiotec.2014.01.037.
- Langermann S, Möllby R, Burlein JE, Palaszynski S, Auguste C, DeFusco A, Strouse R, Schenerman M, Hultgren S, Pinkner J. et al. Vaccination with FimH adhesin protects cynomolgus monkeys from colonization and infection by uropathogenic Escherichia coli. J Infect Dis. 2000;181(2):774–778. doi:10.1086/315258.
- Brumbaugh AR, Mobley HLT. Preventing urinary tract infection: progress toward an effective Escherichia coli vaccine. Expert Rev Vaccines. 2012;11(6):663–676. doi:10.1586/erv.12.36.
- Hagan EC, Lloyd AL, Rasko DA, Faerber GJ, Mobley HLT, Cheung A. Escherichia coli global gene expression in urine from women with urinary tract infection. PloS Pathog. 2010;6(11):e1001187. doi:10.1371/journal.ppat.1001187.
- Lim JK, Gunther NW, Zhao H, Johnson DE, Keay SK, Mobley HLT. In vivo Phase Variation of Escherichia coli type 1 fimbrial genes in women with urinary tract infection. Infect Immun. 1998;66(7):3303–3310. doi:10.1128/iai.66.7.3303-3310.1998.
- Eisenstein BI. Phase variation of type 1 fimbriae in Escherichia coli is under transcriptional control. Science. 1981;214(4518):337–339. doi:10.1126/science.6116279.
- Nowicki B, Rhen M, VäVäIsänenänen-Rhen V, Pere A, Korhonen TK. Immunofluorescence study of fimbrial phase variation in Escherichia coli KS71. J Bacteriol. 1984;160(2):691–695. doi:10.1128/jb.160.2.691-695.1984.
- Tchesnokova V, Aprikian P, Kisiela D, Gowey S, Korotkova N, Thomas W, Sokurenko E. Type 1 fimbrial adhesin FimH elicits an immune response that enhances cell adhesion of Escherichia coli. Infect Immun. 2011;79(10):3895–3904. doi:10.1128/iai.05169-11.
- Eldridge GR, Hughey H, Rosenberger L, Martin SM, Shapiro AM, D’Antonio E, Krejci KG, Shore N, Peterson J, Lukes AS. et al. Safety and immunogenicity of an adjuvanted Escherichia coli adhesin vaccine in healthy women with and without histories of recurrent urinary tract infections: results from a first-in-human phase 1 study. Hum Vaccin Immunother. 2021;17(5):1262–1270. doi:10.1080/21645515.2020.1834807.
- Moriel DG, Bertoldi I, Spagnuolo A, Marchi S, Rosini R, Nesta B, Pastorello I, Corea VAM, Torricelli G, Cartocci E. et al. Identification of protective and broadly conserved vaccine antigens from the genome of extraintestinal pathogenic Escherichia coli. Proc Natl Acad Sci USA. 2010;107(20):9072–9077. doi:10.1073/pnas.0915077107.
- Goluszko P, Goluszko E, Nowicki B, Nowicki S, Popov V, Wang HQ. Vaccination with purified dr Fimbriae reduces mortality associated with chronic urinary tract infection due to Escherichia coli bearing dr adhesin. Infect Immun. 2005;73(1):627–631. doi:10.1128/IAI.73.1.627-631.2005.
- Roberts JA, Kaack MB, Baskin G, Chapman MR, Hunstad DA, Pinkner JS, Hultgren SJ. Antibody responses and protection from pyelonephritis following vaccination with purified Escherichia coli PapDG protein. J Urol. 2004;171(4):1682–1685. doi:10.1097/01.ju.0000116123.05160.43.
- Nesta B, Spraggon G, Alteri C, Gomes Moriel D, Rosini R, Veggi D, Smith S, Bertoldi I, Pastorello I, Ferlenghi I. et al. FdeC, a novel broadly conserved Escherichia coli adhesin eliciting protection against urinary tract infections. mBio. 2012;3(2): doi:10.1128/mbio.00010-12.
- Reigstad CS, Hultgren SJ, Gordon JI. Functional genomic studies of uropathogenic Escherichia coli and host urothelial cells when intracellular bacterial communities are assembled. J Biol Chem. 2007;282(29):21259–21267. doi:10.1074/jbc.M611502200.
- Snyder JA, Haugen BJ, Buckles EL, Lockatell CV, Johnson DE, Donnenberg MS, Welch RA, Mobley HLT. Transcriptome of uropathogenic Escherichia coli during urinary tract infection. Infect Immun. 2004;72(11):6373–6381. doi:10.1128/iai.72.11.6373-6381.2004.
- Hagan EC, Mobley HLT. Haem acquisition is facilitated by a novel receptor Hma and required by uropathogenic Escherichia coli for kidney infection. Mol Microbiol. 2009;71(1):79–91. doi:10.1111/j.1365-2958.2008.06509.x.
- Bauckman KA, Mysorekar IU. Ferritinophagy drives uropathogenic Escherichia coli persistence in bladder epithelial cells. Autophagy. 2016;12(5):850–863. doi:10.1080/15548627.2016.1160176.
- Alteri CJ, Hagan EC, Sivick KE, Smith SN, Mobley HLT, Seifert HS. Mucosal immunization with iron receptor antigens protects against urinary tract infection. PLOS Pathog. 2009;5(9):e1000586. doi:10.1371/journal.ppat.1000586.
- Durant L, Metais A, Soulama-Mouze C, Genevard JM, Nassif X, Escaich S. Identification of candidates for a subunit vaccine against extraintestinal pathogenic Escherichia coli. Infect Immun. 2007;75(4):1916–1925. doi:10.1128/IAI.01269-06.
- Russo TA, McFadden CD, Carlino-MacDonald UB, Beanan JM, Olson R, Wilding GE. The siderophore receptor IroN of extraintestinal pathogenic Escherichia coli is a potential vaccine candidate. Infect Immun. 2003;71(12):7164–7169. doi:10.1128/IAI.71.12.7164-7169.2003.
- Brumbaugh AR, Smith SN, Mobley HLT, Payne SM. Immunization with the yersiniabactin receptor, FyuA, protects against pyelonephritis in a murine model of urinary tract infection. Infect Immun. 2013;81(9):3309–3316. doi:10.1128/iai.00470-13.
- Habibi M, Asadi Karam MR, Bouzari S. Evaluation of prevalence, immunogenicity and efficacy of FyuA iron receptor in uropathogenic Escherichia coli isolates as a vaccine target against urinary tract infection. Microb Pathog. 2017;110:477–483. doi:10.1016/j.micpath.2017.07.037.
- Wieser A, Magistro G, Nörenberg D, Hoffmann C, Schubert S. First multi-epitope subunit vaccine against extraintestinal pathogenic Escherichia coli delivered by a bacterial type-3 secretion system (T3SS). Int J Med Microbiol. 2012;302(1):10–18. doi:10.1016/j.ijmm.2011.09.012.
- Mike LA, Smith SN, Sumner CA, Eaton KA, Mobley HLT. Siderophore vaccine conjugates protect against uropathogenic Escherichia coli urinary tract infection. Proc Natl Acad Sci. 2016;113(47):13468–13473. doi:10.1073/pnas.1606324113.
- Wiles TJ, Kulesus RR, Mulvey MA. Origins and virulence mechanisms of uropathogenic Escherichia coli. Exp Mol Pathol. 2008;85(1):11–19. doi:10.1016/j.yexmp.2008.03.007.
- Smith MA, Weingarten RA, Russo LM, Ventura CL, O’Brien AD, Payne SM. Antibodies against hemolysin and cytotoxic necrotizing factor type 1 (CNF1) reduce bladder inflammation in a mouse model of urinary tract infection with toxigenic uropathogenic Escherichia coli. Infect Immun. 2015;83(4):1661–1673. doi:10.1128/IAI.02848-14.
- Zimmermann P, Curtis N. Factors that influence the immune response to vaccination. Clin Microbiol Rev. 2019;32(2): doi:10.1128/cmr.00084-18.
- Pulendran B, Ahmed R. Immunological mechanisms of vaccination. Nat Immunol. 2011;12(6):509–517. doi:10.1038/ni.2039.
- Siegrist CA. 2 - vaccine immunology. In: Plotkin S, Orenstein W, Offit P, and Edwards K. editors. Plotkin’s Vaccines. 7th ed. Philadelphia, PA: Elsevier. 2018. p. 16–34.e7. doi:10.1016/B978-0-323-35761-6.00002-X.
- Plotkin SA, Gilbert P. 3 - correlates of protection. In: Plotkin S, Orenstein W, Offit P, and Edwards K. editors. Plotkin’s Vaccines. 7th ed. Philadelphia, PA: Elsevier. 2018. p. 35–40.e4. doi:10.1016/B978-0-323-35761-6.00003-1.
- Diphtheria T. And pertussis: recommendations for vaccine use and other preventive measures. Recommendations of the immunization practices advisory committee (ACIP). MMWR Recomm Rep. 1991;40(RR–10):1–28.
- Dijkman K, Sombroek CC, Vervenne RAW, Hofman SO, Boot C, Remarque EJ, Kocken CHM, Ottenhoff THM, Kondova I, Khayum MA. et al. Prevention of tuberculosis infection and disease by local BCG in repeatedly exposed rhesus macaques. Nat Med. 2019;25(2):255–262. doi:10.1038/s41591-018-0319-9.
- Gideon HP, Phuah J, Myers AJ, Bryson BD, Rodgers MA, Coleman MT, Maiello P, Rutledge T, Marino S, Fortune SM. et al. Variability in tuberculosis granuloma T cell responses exists, but a balance of pro- and anti-inflammatory cytokines is associated with sterilization. PLOS Pathog. 2015;11(1):e1004603. doi:10.1371/journal.ppat.1004603.
- Thumbikat P, Waltenbaugh C, Schaeffer AJ, Klumpp DJ. Antigen-specific responses accelerate bacterial clearance in the Bladder1. J Immunol. 2006;176(5):3080–3086. doi:10.4049/jimmunol.176.5.3080.
- Mulvey MA, Schilling JD, Hultgren SJ, O’Brien AD. Establishment of a persistent Escherichia coli reservoir during the acute phase of a bladder infection. Infect Immun. 2001;69(7):4572–4579. doi:10.1128/iai.69.7.4572-4579.2001.
- Lukens JR, Anand PK. Adapt(ed) to repair — TH2 immune responses in the bladder promote recurrent infections. Nat Immunol. 2020;21(6):597–599. doi:10.1038/s41590-020-0689-2.
- Sharma K, Thacker VV, Dhar N, Clapés Cabrer M, Dubois A, Signorino-Gelo F, Mullenders J, Knott GW, Clevers H, McKinney JD. Early invasion of the bladder wall by solitary bacteria protects UPEC from antibiotics and neutrophil swarms in an organoid model. Cell Rep. 2021;36(3):109351. doi:10.1016/j.celrep.2021.109351.
- Blango MG, Ott EM, Erman A, Veranic P, Mulvey MA, Beloin C. Forced resurgence and targeting of intracellular uropathogenic Escherichia coli reservoirs. PLoS One. 2014;9(3):e93327. doi:10.1371/journal.pone.0093327.
- Wu J, Hayes BW, Phoenix C, Macias GS, Miao Y, Choi HW, Hughes FM, Todd Purves J, Lee Reinhardt R, Abraham SN. et al. A highly polarized TH2 bladder response to infection promotes epithelial repair at the expense of preventing new infections. Nat Immunol. 2020;21(6):671–683. doi:10.1038/s41590-020-0688-3.
- Wu J, Bao C, Reinhardt RL, Abraham SN. Local induction of bladder Th1 responses to combat urinary tract infections. Proc Natl Acad Sci. 2021;118(10):e2026461118. doi:10.1073/pnas.2026461118.
- Sallusto F, Lanzavecchia A, Araki K, Ahmed R. From vaccines to memory and back. Immunity. 2010;33(4):451–463. doi:10.1016/j.immuni.2010.10.008.
- Mora-Bau G, Platt AM, van RN, Randolph GJ, Albert ML, Ingersoll MA, Gause WC. Macrophages subvert adaptive immunity to urinary tract infection. PloS Pathog. 2015;11(7):e1005044. doi:10.1371/journal.ppat.1005044.
- Rousseau M, Lacerda Mariano L, Canton T, Ingersoll MA. Tissue-resident memory T cells mediate mucosal immunity to recurrent urinary tract infection. Sci Immunol. 2023;8(83):eabn4332. doi:10.1126/sciimmunol.abn4332.
- Lavelle EC, Ward RW. Mucosal vaccines — fortifying the frontiers. Nat Rev Immunol. 2022;22(4):236–250. doi:10.1038/s41577-021-00583-2.
- Marinova S, Nenkov P, Markova R, Nikolaeva S, Kostadinova R, Mitov I, Vretenarska M. Cellular and humoral systemic and mucosal immune responses stimulated by an oral polybacterial immunomodulator in patients with chronic urinary tract infections. Int J Immunopathol Pharmacol. 2005;18(3):457–473. doi:10.1177/039463200501800306.
- Forsyth VS, Himpsl SD, Smith SN, Sarkissian CA, Mike LA, Stocki JA, Sintsova A, Alteri CJ, Mobley HLT. et al. Optimization of an experimental vaccine to prevent Escherichia coli urinary tract infection. mBio. 2020;11(2): doi:10.1128/mbio.00555-20.
- Qadri F, Akhtar M, Bhuiyan TR, Chowdhury MI, Ahmed T, Rafique TA, Khan A, Rahman SIA, Khanam F, Lundgren A. et al. Safety and immunogenicity of the oral, inactivated, enterotoxigenic Escherichia coli vaccine ETVAX in Bangladeshi children and infants: a double-blind, randomised, placebo-controlled phase 1/2 trial. Lancet Infect Dis. 2020;20(2):208–219. doi:10.1016/S1473-3099(19)30571-7.
- Holmgren J, Bourgeois L, Carlin N, Clements J, Gustafsson B, Lundgren A, Nygren E, Tobias J, Walker R, Svennerholm A-M. et al. Development and preclinical evaluation of safety and immunogenicity of an oral ETEC vaccine containing inactivated E. coli bacteria overexpressing colonization factors CFA/I, CS3, CS5 and CS6 combined with a hybrid LT/CT B subunit antigen, administered alone and together with dmLT adjuvant. Vaccine. 2013;31(20):2457–2464. doi:10.1016/j.vaccine.2013.03.027.
- Kennedy KJ, Roberts JL, Collignon PJ. Escherichia coli bacteraemia in Canberra: incidence and clinical features. Med J Aust. 2008;188(4):209–213. doi:10.5694/j.1326-5377.2008.tb01586.x.
- Baudron CR, Panhard X, Clermont O, MENTRÉ F, Fantin B, Denamur E, Lefort A. Escherichia coli bacteraemia in adults: age-related differences in clinical and bacteriological characteristics, and outcome. Epidemiol Infect. 2014;142(12):2672–2683. doi:10.1017/S0950268814000211.
- Chu CM, Lowder JL. Diagnosis and treatment of urinary tract infections across age groups. Am J Obstet Gynecol. 2018;219(1):40–51. doi:10.1016/j.ajog.2017.12.231.
- Rowe TA, Juthani-Mehta M. Urinary tract infection in older adults. Aging Health. 2013;9(5):519–528. doi:10.2217/ahe.13.38.
- Crooke SN, Ovsyannikova IG, Poland GA, Kennedy RB. Immunosenescence and human vaccine immune responses. Immun Ageing. 2019;16(1):25. doi:10.1186/s12979-019-0164-9.
- Lazuardi L, Jenewein B, Wolf AM, Pfister G, Tzankov A, Grubeck-Loebenstein B. Age-related loss of naïve T cells and dysregulation of T-cell/B-cell interactions in human lymph nodes. Immunology. 2005;114(1):37–43. doi:10.1111/j.1365-2567.2004.02006.x.
- Bulati M, Buffa S, Candore G, Caruso C, Dunn-Walters DK, Pellicanò M, Wu Y-C, Colonna Romano G. B cells and immunosenescence: a focus on IgG+IgD−CD27− (DN) B cells in aged humans. Ageing Res Rev. 2011;10(2):274–284. doi:10.1016/j.arr.2010.12.002.
- Listì F, Candore G, Modica MA, Russo M, Lorenzo GD, Esposito‐Pellitteri M, Colonna‐Romano G, Aquino A, Bulati M, Lio D. et al. A study of serum immunoglobulin levels in elderly persons that provides new insights into B cell immunosenescence. Ann N Y Acad Sci. 2006;1089(1):487–495. doi:10.1196/annals.1386.013.
- Goodwin K, Viboud C, Simonsen L. Antibody response to influenza vaccination in the elderly: a quantitative review. Vaccine. 2006;24(8):1159–1169. doi:10.1016/j.vaccine.2005.08.105.
- Sasaki S, Sullivan M, Narvaez CF, Holmes TH, Furman D, Zheng N-Y, Nishtala M, Wrammert J, Smith K, James JA. et al. Limited efficacy of inactivated influenza vaccine in elderly individuals is associated with decreased production of vaccine-specific antibodies. J Clin Invest. 2011;121(8):3109–3119. doi:10.1172/JCI57834.
- Deng Y, Jing Y, Campbell AE, Gravenstein S. Age-related impaired type 1 T cell responses to influenza: reduced activation ex vivo, decreased expansion in CTL culture in vitro, and blunted response to influenza vaccination in vivo in the elderly. J Immunol. 2004;172(6):3437–3446. doi:10.4049/jimmunol.172.6.3437.
- Tseng HF, Harpaz R, Luo Y, Hales, CM, Sy, LS, Tartof, SY, Bialek, S, Hechter, RC, Jacobsen, SJ. et al. Declining effectiveness of herpes zoster vaccine in adults aged ≥60 years. J Infect Dis. 2016;213(12):1872–1875. doi:10.1093/infdis/jiw047.
- Coleman BL, Sanderson R, Haag MDM, McGovern I. Effectiveness of the MF59-adjuvanted trivalent or quadrivalent seasonal influenza vaccine among adults 65 years of age or older, a systematic review and meta-analysis. Influenza Other Respi Viruses. 2021;15(6):813–823. doi:10.1111/irv.12871.
- Lal H, Cunningham AL, Godeaux O, Chlibek R, Diez-Domingo J, Hwang S-J, Levin MJ, McElhaney JE, Poder A, Puig-Barberà J. et al. Efficacy of an adjuvanted herpes zoster subunit vaccine in older adults. N Engl J Med. 2015;372(22):2087–2096. doi:10.1056/NEJMoa1501184.
- Schwarz TF, Volpe S, Catteau G, Chlibek R, David MP, Richardus JH, Lal H, Oostvogels L, Pauksens K, Ravault S. et al. Persistence of immune response to an adjuvanted varicella-zoster virus subunit vaccine for up to year nine in older adults. Hum Vaccin Immunother. 2018;14(6):1370–1377. doi:10.1080/21645515.2018.1442162.
- Gebre MS, Brito LA, Tostanoski LH, Edwards DK, Carfi A, Barouch DH. Novel approaches for vaccine development. Cell. 2021;184(6):1589–1603. doi:10.1016/j.cell.2021.02.030.
- Brisse M, Vrba SM, Kirk N, Liang Y, Ly H. Emerging Concepts and technologies in vaccine development. Front Immunol. 2020;11:11. doi:10.3389/fimmu.2020.583077.
- Tam HH, Melo MB, Kang M, Pelet JM, Ruda VM, Foley MH, Hu JK, Kumari S, Crampton J, Baldeon AD. et al. Sustained antigen availability during germinal center initiation enhances antibody responses to vaccination. Proc Natl Acad Sci. 2016;113(43):E6639–E6648. doi:10.1073/pnas.1606050113.
- Cirelli KM, Crotty S. Germinal center enhancement by extended antigen availability. Curr Opin Immunol. 2017;47:64–69. doi:10.1016/j.coi.2017.06.008.
- Cirelli KM, Carnathan DG, Nogal B, Martin JT, Rodriguez OL, Upadhyay AA, Enemuo CA, Gebru EH, Choe Y, Viviano F. et al. Slow delivery immunization enhances HIV neutralizing antibody and germinal center responses via modulation of immunodominance. Cell. 2019;177(5):1153–1171.e28. doi:10.1016/j.cell.2019.04.012.
- Byrd W, de Lorimier A, Zheng ZR, Cassels FJ. Microencapsulated subunit vaccine approach to enterotoxigenic Escherichia coli and other mucosal pathogens. Adv Drug Deliv Rev. 2005;57(9):1362–1380. doi:10.1016/j.addr.2005.01.014.
- Rezaei M, Esmaeili F, Reza Asadi Karam M, Ehsani P, Abbasnezhad Farsangi Z, Bouzari S. In silico design and in vivo evaluation of two multi-epitope vaccines containing build-in adjuvant with chitosan nanoparticles against uropathogenic Escherichia coli. Int Immunopharmacol. 2023;117:109999. doi:10.1016/j.intimp.2023.109999.
- Gu H, Liao Y, Zhang J, Wang Y, Liu Z, Cheng P, Wang X, Zou Q, Gu J. Rational design and evaluation of an artificial Escherichia coli K1 protein vaccine candidate based on the structure of OmpA. Front Cell Infect Microbiol. 2018 [accessed 2023 Oct 16]. 8. https://www.frontiersin.org/articles/10.3389/fcimb.2018.00172.
- Zhang J, Sun H, Gao C, Wang Y, Cheng X, Yang Y, Gou Q, Lei L, Chen Y, Wang X. et al. Development of a chitosan‐modified PLGA nanoparticle vaccine for protection against Escherichia coli K1 caused meningitis in mice. J Nanobiotechnology. 2021;19(1):69. doi:10.1186/s12951-021-00812-9.
- Luzuriaga MA, Herbert FC, Brohlin OR, Gadhvi J, Howlett T, Shahrivarkevishahi A, Wijesundara YH, Venkitapathi S, Veera K, Ehrman R. et al. Metal–organic framework encapsulated whole-cell vaccines enhance humoral immunity against bacterial infection. Acs Nano. 2021;15(11):17426–17438. doi:10.1021/acsnano.1c03092.
- Kelly SH, Wu Y, Varadhan AK, Curvino EJ, Chong AS, Collier JH. Enabling sublingual peptide immunization with molecular self-assemblies. Biomaterials. 2020;241:119903. doi:10.1016/j.biomaterials.2020.119903.
- Kelly SH, Votaw NL, Cossette BJ, Wu Y, Shetty S, Shores LS, Issah LA, Collier JH. A sublingual nanofiber vaccine to prevent urinary tract infections. Sci Adv. 2022;8(47). doi:10.1126/sciadv.abq4120.
- Kutzler MA, Weiner DB. DNA vaccines: ready for prime time? Nat Rev Genet. 2008;9(10):776–788. doi:10.1038/nrg2432.
- Imani Fooladi AA, Bagherpour G, Khoramabadi N, Fallah Mehrabadi J, Mahdavi M, Halabian R, Amin M, Izadi Mobarakeh J, Einollahi B. Cellular immunity survey against urinary tract infection using pVAX/fimH cassette with mammalian and wild type codon usage as a DNA vaccine. Clin Exp Vaccine Res. 2014;3(2):185–193. doi:10.7774/cevr.2014.3.2.185.
- Bakhtiari R, Ahmadian S, Fallah Mehrabadi J. Rising cellular immune response after injection of pVax/iutA: A genetic DNA cassette as candidate vaccine against urinary tract infection. Iran J Public Health. 2016;45(7):890–896.
- Denamur E, Clermont O, Bonacorsi S, Gordon D. The population genetics of pathogenic Escherichia coli. Nat Rev Microbiol. 2021;19(1):37–54. doi:10.1038/s41579-020-0416-x.
- Whitfield C. Biosynthesis and assembly of capsular polysaccharides in Escherichia coli. Annu Rev Biochem. 2006;75(1):39–68. doi:10.1146/annurev.biochem.75.103004.142545.
- Picard B, Garcia JS, Gouriou S, Duriez P, Brahimi N, Bingen E, Elion J, Denamur E. The link between phylogeny and virulence in Escherichia coli extraintestinal infection. Infect Immun. 1999;67(2):546–553. doi:10.1128/IAI.67.2.546-553.1999.
- Escobar-Páramo P, Clermont O, Blanc-Potard AB, Bui H, Le Bouguénec C, Denamur E. A specific genetic background is required for acquisition and expression of virulence factors in Escherichia coli. Mol Biol Evol. 2004;21(6):1085–1094. doi:10.1093/molbev/msh118.
- Mehat JW, van Vliet AHM, La Ragione RM. The avian pathogenic Escherichia coli (APEC) pathotype is comprised of multiple distinct, independent genotypes. Avian Pathol. 2021;50(5):402–416. doi:10.1080/03079457.2021.1915960.
- Eichhorn I, Heidemanns K, Semmler T, Kinnemann B, Mellmann A, Harmsen D, Anjum MF, Schmidt H, Fruth A, Valentin-Weigand P. et al. Highly virulent non-O157 Enterohemorrhagic Escherichia coli (EHEC) serotypes reflect similar phylogenetic lineages, providing new insights into the evolution of EHEC. Appl Environ Microbiol. 2015;81(20):7041–7047. doi:10.1128/AEM.01921-15.
- Tenaillon O, Skurnik D, Picard B, Denamur E. The population genetics of commensal Escherichia coli. Nat Rev Microbiol. 2010;8(3):207–217. doi:10.1038/nrmicro2298.
- Clermont O, Dixit OVA, Vangchhia B, Condamine B, Dion S, Bridier‐Nahmias A, Denamur E, Gordon D. Characterization and rapid identification of phylogroup G in Escherichia coli, a lineage with high virulence and antibiotic resistance potential. Environ Microbiol. 2019;21(8):3107–3117. doi:10.1111/1462-2920.14713.
- Vangchhia B, Abraham S, Bell JM, Collignon P, Gibson JS, Ingram PR, Johnson JR, Kennedy K, Trott DJ, Turnidge JD. et al. Phylogenetic diversity, antimicrobial susceptibility and virulence characteristics of phylogroup F Escherichia coli in Australia. Microbiol. 2016;162(11):1904–1912. doi:10.1099/mic.0.000367.
- Escobar-Páramo P, Grenet K, Le Menac’h A, Rode L, Salgado E, Amorin C, Gouriou S, Picard B, Rahimy MC, Andremont A. et al. Large-scale population structure of human commensal Escherichia coli isolates. Appl Environ Microbiol. 2004;70(9):5698–5700. doi:10.1128/AEM.70.9.5698-5700.2004.
- Duriez P, Clermont O, Bonacorsi S, Bingen E, Chaventré A, Elion J, Picard B, Denamur E. Commensal Escherichia coli isolates are phylogenetically distributed among geographically distinct human populations. Microbiol. 2001;147(6):1671–1676. doi:10.1099/00221287-147-6-1671.
- Gomez A, Petrzelkova KJ, Burns MB, Yeoman C, Amato K, Vlckova K, Modry D, Todd A, Jost Robinson C, Remis M. et al. Gut microbiome of coexisting BaAka pygmies and bantu reflects gradients of traditional subsistence patterns. Cell Rep. 2016;14(9):2142–2153. doi:10.1016/j.celrep.2016.02.013.
- Lescat M, Clermont O, Woerther PL, Glodt J, Dion S, Skurnik D, Djossou F, Dupont C, Perroz G, Picard B. et al. Commensal Escherichia coli strains in Guiana reveal a high genetic diversity with host-dependant population structure. Environ Microbiol Rep. 2013;5(1):49–57. doi:10.1111/j.1758-2229.2012.00374.x.
- Touchon M, Perrin A, de SJ, Vangchhia B, Burn S, O’Brien CL, Denamur E, Gordon D, Rocha EP. Phylogenetic background and habitat drive the genetic diversification of Escherichia coli. PLOS Genet. 2020;16(6):e1008866. doi:10.1371/journal.pgen.1008866.
- Arimizu Y, Kirino Y, Sato MP, Uno K, Sato T, Gotoh Y, Auvray F, Brugere H, Oswald E, Mainil JG. et al. Large-scale genome analysis of bovine commensal Escherichia coli reveals that bovine-adapted E. coli lineages are serving as evolutionary sources of the emergence of human intestinal pathogenic strains. Genome Res. 2019;29(9):1495–1505. doi:10.1101/gr.249268.119.
- Massot M, Daubié AS, Clermont O, Jauréguy F, Couffignal C, Dahbi G, Mora A, Blanco J, Branger C, Mentré F. et al. Phylogenetic, virulence and antibiotic resistance characteristics of commensal strain populations of Escherichia coli from community subjects in the Paris area in 2010 and evolution over 30 years. Microbiol. 2016;162(4):642–650. doi:10.1099/mic.0.000242.
- Mercat M, Clermont O, Massot M, Ruppe E, de Garine-Wichatitsky M, Miguel E, Valls Fox H, Cornelis D, Andremont A, Denamur E. et al. Escherichia coli population structure and antibiotic resistance at a buffalo/cattle interface in Southern Africa. Appl Environ Microbiol. 2015;82(5):1459–1467. doi:10.1128/AEM.03771-15.
- Coura FM, de A Diniz S, Silva MX, Mussi JMS, Barbosa SM, Lage AP, Heinemann MB. Phylogenetic group determination of Escherichia coli Isolated from animals samples. The Scientific World Journal. 2015;2015:1–4. doi:10.1155/2015/258424.
- Clermont O, Condamine B, Dion S, Gordon DM, Denamur E. The E phylogroup of Escherichia coli is highly diverse and mimics the whole E. coli species population structure. Environ Microbiol. 2021;23(11):7139–7151. doi:10.1111/1462-2920.15742.