ABSTRACT
The tight and coordinated regulation of virulence gene expression is crucial to ensure the survival and persistence of bacterial pathogens in different contexts within their hosts. Considering this, bacteria do not express virulence factors homogenously in time and space, either due to their associated fitness cost or to their detrimental effect at specific infection stages. To efficiently infect and persist into their hosts, bacteria have thus to monitor environmental cues or chemical cell-to-cell signaling mechanisms that allow their transition from the external environment to the host, and therefore adjust gene expression levels, intrinsic biological activities, and appropriate behaviors. Listeria monocytogenes (Lm), a major Gram-positive facultative intracellular pathogen, stands out for its adaptability and capacity to thrive in a wide range of environments. Because of that, Lm presents itself as a significant concern in food safety and public health, that can lead to potentially life-threatening infections in humans. A deeper understanding of the intricate bacterial virulence mechanisms and the signals that control them provide valuable insights into the dynamic interplay between Lm and the host. Therefore, this review addresses the role of some crucial signals behind Lm pathogenic virulence mechanisms and explores how the ability to assimilate and interpret these signals is fundamental for pathogenesis, identifying potential targets for innovative antimicrobial strategies.
Introduction
Pathogen adaptability is pivotal to the infection outcome and is determined by bacterial factors allowing the establishment and progression of the infection. Importantly, the tight and coordinated regulation of these virulence genes is crucial and strategic for their expression in specific time-frames, within particular tissues, or by bacteria subsets. Moreover, the untimely expression of virulence factors represents a major fitness burden that can be detrimental to a successful survival strategy, once bacteria do not express virulence factors homogenously in time and space. Infections caused by Gram-positive bacteria are challenging and still remain a major public health. Listeria monocytogenes (Lm) is a major Gram-positive intracellular foodborne pathogen which causes a life-threatening infection, listeriosis, acquired from the ingestion of contaminated food. It is the most severe human systemic infection among zoonotic diseases under EU surveillance. It can be particularly severe in specific high-risk groups, including pregnant women, elderly and immunocompromised people. Despite the availability of antibiotics-based treatment, listeriosis remains the most frequent cause of death due to the consumption of contaminated food in Europe, with the higher proportion of hospitalized cases (~96%) and a case mortality rate of ~18.1%.Citation1,Citation2 Lm is ubiquitously found in a wide range of environments, such as soil, water, animal feces and vegetation, being constantly exposed to a number of stress adaptations both throughout its saprophytic life and transiting to infect human tissues. Once inside the host, it is capable to invade and colonize the gut, transverse the intestinal barrier and disseminate, establishing a systemic infection, and replicating within target organs.Citation3,Citation4 Importantly, the expression of virulence factors is directly dependent on the Lm ability to detect and process signals from the surrounding environment adjusting its physiology accordingly.
The coordinated phenotypic expression of Lm virulence factors and the underlying mechanisms of their regulation provide a competitive advantage for the bacterial community in different contexts, such as biofilm formation, host infectionor antibiotic resistance. This review focuses on the role of different signals behind Lm virulence gene expression and how they impact bacterial communication throughout host infection and therefore pathogenesis. Since the successful host colonization by pathogens is conditioned by the signals they integrate, it is crucial to understand how these signals act to further modulate bacterial colonization and therefore control disease. Importantly, most of the signals required to control virulence mechanisms are transversal to different pathogens. This review provides an overview of both the signaling molecules generated by Lm itself for its own profit and the nutritional and physical external signals that control bacterial virulence ().
Table 1. Summary of signals sensed by Lm to modulate virulence.
Listeria monocytogenes signaling molecules regulating virulence
Pathogenic bacteria have developed several strategies to survive and persist within host cells and tissues. In Lm, most of these strategies are controlled and/or influenced by cell-to-cell communication, a process mediated by molecules produced and secreted by bacteria themselves. These signaling molecules are part of a dialogue between bacteria, and can be either chemical and cell-population density related (e.g quorum-sensing, pheromones) or generated by bacteria at different infection stages (e.g RNAs, second messengers, and glutathione), being both essential to ensure the successful establishment of infection.Citation90
Quorum sensing
Quorum sensing (QS) is a microbial cell-to-cell communication process that relies on the production, secretion, detection, and group-wide accumulation of extracellular signaling molecules named autoinducers. Throughout this process, bacteria share information concerning the population density to accordingly regulate the expression of genes involved in virulence, competition, stress response, and resistance.Citation91,Citation92
Pathogenic Gram-positive bacteria typically produce autoinducing peptides (AIPs) as autoinducers, being initially synthesized as precursor peptides (pro-AIPs), modified, and then exported through a specialized protein transport system.Citation93,Citation94 AIPs are generally sensed and recognized by a two-component signal transduction system. High cell density communities accumulate higher concentrations of extracellular AIP, which binds to a membrane-bound histidine kinase receptor triggering its own phosphorylation and transferring the phosphate group to a cognate cytoplasmic response-regulator, that ultimately regulate the expression of QS related-genes.Citation93,Citation95,Citation96 Moreover, AIPs can be transported back to the bacterial cytoplasm where they are capable to interact with cytoplasmic transcriptional factors, thus modulating their activity and gene expression pattern.Citation97,Citation98 The Agr system and its orthologs are the most well-studied circuits of bacterial QS, mainly in Staphylococcus aureus, and play a fundamental role in the spatiotemporal expression of virulence mechanisms of Gram-positive human pathogens, including Enterecoccus faecalis, Clostridium perfringens and Lm.Citation5,Citation99-102
Lm QS systems have been associated with bacterial communication and survival providing competitive advantages in adhesion to surfaces, biofilm formation, invasion of mammalian cells, mouse infection, and changes in gene expression ().Citation5,Citation99,Citation107,Citation108 Two QS systems, Lux, and Agr, widespread among different bacterial species, were already established for Lm. The Lux system () involves the conversion of S-adenosylhomocysteine (SAH) into S-ribosylhomocysteine (SRH) that is further processed to give rise to both homocysteine and 4,5-dihydroxy-2,3-pentanedione (DPD). This latter reaction is achieved by two Lm enzymes, Pfs (S-adenosylhomocysteine nucleosidase) and LuxS (S-ribosylhomocysteinase). Thereon, DPD rearranges into a furanone derivative called autoinducer AI-2, which is then released to the extracellular media.Citation6,Citation12 AI-2 was found to be important only for the detoxification of SAH, while its precursor SRH is important for biofilm formation.Citation12 Importantly, both SAH and SRH are by-products generated in the activated methyl cycle (AMC) from S-adenosylmethionine (SAM) processing. SAM is an intermediate metabolite generated from methionine in the MetK synthase cascade pathway, and is recognized as a sentinel metabolite capable of bind to 5’ UTRs riboswitches controlling the transcription of their downstream genes, mainly involved in the biosynthesis, transport, and utilization of amino acids, oligopeptides and SAM itself: between them, the adenosylmethionine synthetase (metK) and the methionine (lmo2417) and cysteine (lm0135) transporters.Citation6-9
Figure 1. Signalling of Lm virulence gene expression during the infection of the gastrointestinal (GI) tract. (a) The temperature within the mammalian host is key for Lm to modulate the expression of virulence genes. This thermal cue is sensed both by the Lm pleiotropic regulator prfA, whose ribosome binding region becomes accessible enabling the translation of downstream mRNA,Citation25 and by the anti-repressor GmaR that suffers a conformational shift, allowing the expression of MogR and consequently the repression of genes involved in flagellum biosynthesis. Moreover, the Anti0677 is capable of inducing the transcription of mogR by generating a longer anti0677 transcript, positioned on the opposite strand of the flagellum operon coding sequence (fliN, fliP and fliQ).Citation41 within the gastrointestinal tract, Lm encounters bile salts. It uses Bsh, which is crucial for Lm resistance to bile and virulence, to catalyze the hydrolysis of GDCA- and TDCA-conjugated bile salts into free cholic acids, that are exported by the MdrT efflux pump of the bacterium.Citation66 Consequently, in the presence of cholic acids, the bile sensor BrtA, which controls mdrT, is no longer able to repress Lm MdrT.Citation67 (b) the ability of Lm to internalize intestinal epithelial cells relies, in part, on its capacity to form biofilms at the surface of the intestinal villi, which is dependent on MouR that positively regulates the agr operon by binding to its promoter region.Citation103 AgrD is processed by AgrB into a mature AIP, that is subsequently secreted to the extracellular medium. The secreted AIP is then recognized by the sensor kinase AgrC present within the neighboring bacteria, triggering a phosphorylation cascade that culminates in the activation of the response regulator AgrA. In turn, AgrA induces the auto-activation circuit. Therefore, the Agr system induces chitinase activity by the down-regulation of the ncRNA lhrA, which blocks translation by binding itself to chitinase mRNA (chiA). In turn, chitinase represses the inducible nitric oxide synthase (iNOS), known to be part of the immune response.Citation27,Citation104 (c) the activated methyl cycle (AMC) also plays a role in biofilm formation by the generation of the S-ribosylhomocysteine (SHR) by-product, through the processing of S-adenosylmethionine (SAM). Concomitantly, SAM is used to bind and activate SreA riboswitch, that in turn increases the expression of agrD.Citation5,Citation8,Citation10 Simultaneously, the activation of Lm SreA riboswitch by SAM inhibits the prfA transcription in the intestine.Citation3,Citation10 (d) Additionally, within the GI Lm comes across ethanolamine, a metabolite derived from the phospholipids of eukaryotic cell membranes, using it as carbon source to support bacterium growth.Citation105 in presence of ethanolamine, the sensor kinase EutW induces the phosphorylation and activation of the anti-terminator EutV, that together with Rli55 repression, upregulate the expression of the ethanolamine operon eut. The repression of Rli55 is dependent on a B12-riboswitch, located upstream of the eut operon, which in the presence of vitamin B12 blocks the transcription of Rli55 allowing the expression of eut genes. Vitamin B12 is also used as cofactor in propanediol metabolism: in the presence of propanediol, B12 riboswitch ends prematurely the transcription of AsPocR, allowing the transcription of pocR, which is important to activate the pdu and cob operons, respectively involved in propanediol utilization and B12 biosynthesis respectively.Citation106
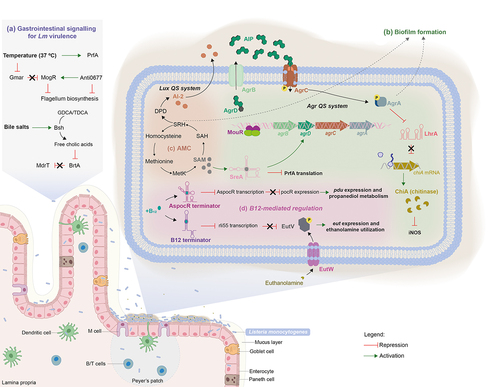
The Lm Agr QS is encoded by the agr locus agrBDCA (). agrD encodes a ribosomal peptide precursor that is proteolytic processed into a mature AIP by the integral membrane-bound peptidase AgrB.Citation13 At this point, in Staphylococcus aureus, it is described the formation of an intermediate enzyme-bound thiolactone, which is then transported across the membrane.Citation109 However, in Lm there is no evidence about the formation of such intermediate. On the other hand, a recent study presented strong evidences that the native Lm AIP might be an homodetic pentamer peptide arising from thiolactone.Citation110 Once the processed AIP is out of the cell, it binds the sensor membrane-bound histidine kinase AgrC, triggering the phosphorylation of the response regulator AgrA, together acting as a two-component system for downstream gene regulation.Citation13 The Lm Agr QS system was found to be positively regulated by MouR, a transcriptional regulator belonging to the VanR class of the GntR superfamily of regulatory proteins.Citation103 The GntR family consists of helix-turn-helix transcriptional regulators that control several metabolic and biological processes in bacteria. These proteins share a conserved DNA-binding domain at the N-terminal, allowing them to modulate gene expression in response to signals, and an effector binding/oligomerization domain at the C-terminal The diversity of the C-terminal domain is used to divide GntR proteins in different sub-classes, where the proteins composed by six α-helices in the C-terminal are allocated to the VanR class.Citation111–113 MouR binds directly to the agr promoter region, modulating both chitinase activity and biofilm formation, thus favoring Lm virulence.Citation103
Previous findings have demonstrated a cooperation between the Agr QS and the SAM signal in the regulation of biofilm formation. The generation of SAM induces the expression of the agr locus, with agrD appearing as the most expressed gene. In contrast, the absence of the Agr QS system significantly impairs SAM-enhanced biofilm formation, but does not affect the expression of the genes involved in SAM biosynthesis.Citation8
Recent efforts have been done to modulate QS and virulence outcome with the goal of designing novel antimicrobial therapeutics. Chemical antagonists able to diminish Lm Agr activity and thus leading to the reduction of biofilm formation by over 90% were recently discovered.Citation114 Moreover, in silico analysis revealed different proteins secreted by lactic acid bacteria working as a promising anti-QS strategy, by inhibiting the translocation of cyclic AIP via AgrB and its recognition by the AgrC active site.Citation115
Of note, some Lm strains like the well-studied laboratory reference strain 10403S harbor mutation in agr genes, being still virulent in mouse infection models.Citation116
Peptide-pheromone pPplA
In addition to the Agr system discussed above, an additional peptide-signaling system has been associated with Lm virulence. Lm displays a small peptide pheromone, pPplA, that is used to signal its presence within the host cell vacuole, facilitating its vacuolar escape possibly by promoting PrfA activation. pPplA is generated via the proteolytic processing of the PplA lipoprotein secretion signal peptide, been then secreted through the general secretory pathway. When confined into the vacuole, Lm is able to sense and import previously produced and accumulated pPlpA, thus initiating a signaling cascade that favors vacuolar disruption and bacterial escape to the host cytosol. This pheromone signaling appears to be linked to PrfA activation.Citation14
RNA-mediated regulation
RNAs have emerged as prime regulators of adaptive response and pathogenesis. This type of regulation is quite present in pathogenic bacteria and provides new information not only about how bacteria can regulate their virulence gene expression but also about their survival associated-phenotypes.Citation117-119 RNA molecules can regulate stress response, metabolism and virulence post-transcriptionally, both through translation and stability of mRNAs encoding genes or interacting with specific proteins.Citation118,Citation120 Importantly, the RNA-mediated regulation presents an advantage for the bacteria throughout infection, since it provides quick responses with minimal consumption of energy.Citation121 Non-coding RNAs (ncRNAs) are functional RNA molecules not translated into a protein. They are crucial gene regulatory elements for Lm, both within or outside the host, and were found to play essential roles in many biological processes including signaling, RNA processing, gene regulation and protein synthesis, which condition virulence and host-immune response.Citation3 Transcriptomics studies of Lm have already identified 46 cis-acting riboswitches, 104 antisense RNAs and 154 putative small trans-acting RNAs.Citation122 In this section, we will focus on the most relevant categories of Lm ncRNAs governing virulence.
The first category of Lm ncRNAs comprises the regulatory elements, usually located in the 5’-untranslated regions (UTRs), and capable to control the expression of virulence genes at the post-transcriptional level.Citation123 Their secondary structure generally suffers a conformational change in response to cellular and/or environmental signals including temperature (thermosensors) or ligand binding (riboswitches).Citation124 The thermosensors regulation depends on temperature to generate an alternative conformation in which the ribosome-binding site is hidden by the thermosensing stretch of the RNA element, thus modulating protein translation.Citation125,Citation126 The most prominent example of this type of regulation in Lm, that signals its presence in mammalian hosts allowing the activation of most virulence mechanisms, is the 5’-UTR of prfA, the major transcriptional activator of Lm adhesins, internalins, phagosome-escaping factors and immune modulating factors.Citation127–130 At low temperatures (<30°C), the prfA-UTR forms a secondary structure that masks the ribosomal region of prfA, avoiding the ribosome binding and consequently inhibiting the translation of PrfA and the expression of PrfA-regulated virulence genes. Temperature rise (>37°C) induces a structural change in the prfA 5’-UTR, allowing ribosome access, the translation of prfA and the expression of PrfA-dependent genes.Citation130 Nonetheless, this thermocontrol appears to act in parallel with other PrfA-activating mechanisms since the temperature increase to 37°C per se is not sufficient to induce PrfA-dependent gene expression.Citation131 In that regard, the regulation of PrfA is also dependent on two SAM riboswitches, SreA and SreB. Interestingly, these riboswitches, due to their high complementary with prfA-5’UTR, may function as trans-ncRNAs, inhibiting the transcription of prfA mRNA.Citation10 Likewise, the absence of SreA decreases the amount of AgrD,Citation8,Citation10 which is crucial for invasion of intestinal Caco2-epithelial cells and biofilm formation.Citation5 SAM riboswitches being usually activated by the binding of their effector molecule S-adenosylmethionine, allow the formation of a termination structure that ends up gene transcription and inhibits the synthesis of downstream mRNA.Citation9,Citation11 However, the SreA-prfA-5’UTR interaction is possible only at temperatures permissive for infection (i.e., 37°C), and does not necessarily require the presence of SAM.Citation10 Altogether, these findings pointed for a role of SAM riboswitches in Lm virulence, downregulating prfA expression in the intestine, where it seems to be less critical than in the blood, keeping active other regulatory pathways such as the Agr system.Citation3,Citation10 Recently, a chimeric antisense oligonucleotide (ASO) was designed as a novel antibacterial agent targeting SAM riboswitch.Citation132
Another regulation dependent on a riboswitch () concerns the ncRNA Rli55, nearby the eut operon involved in the consumption of ethanolamine, an abundant molecule in the human intestine that contributes to Lm mouse infection. Vitamin B12, also named cobalamin, binds to the B12 riboswitch that controls Rli55 expression by ending prematurely its transcription.Citation21 Rli55 functions by binding and sequestering the two-component response regulator EutV. Defects in ethanolamine utilization, or in its regulation by Rli55, significantly attenuate Lm virulence in mice.Citation3,Citation21 The availability of vitamin B12 also regulates the Rli39 riboswitch to control the transcription of AspocR, an antisense RNA. Truncated transcripts of aspocR arise whenever vitamin B12 binds to the riboswitch; contrarily, a full-length transcript is generated in the absence of vitamin B12, inhibiting pocR mRNA translation, through an antisense mechanism. In turn, PocR up-regulate the expression of the pdu genes, which are essential for the propanediol metabolism involved in the pathogenesis of many intestinal pathogens.Citation22 PocR was also proposed to control the expression of Lm cob and cbi genes, that are involved in vitamin B12 de novo biosynthesis, in an attempt to maintain the production of vitamin B12 derived cofactors.Citation23,Citation24
The second category of Lm ncRNAs comprises the antisense RNAs (asRNAs), including long antisense RNAs (lasRNAs), that act as major negative regulators of gene expression and are encoded on the opposite strand in relation with the genes they regulate.Citation123 These type of ncRNAs have full complementary sequence with the mRNA, providing not only strong and specific bindings, but also more efficient action, thus rapidly conditioning mRNA stability and translational activity.Citation120,Citation133 Anti0677 is a lasRNA, named excludon, crucial for Lm infection, that negatively regulates the flagellum biosynthesis, in particular through the control of the MogR transcriptional repressor of flagellum genes. mogR transcription occurs from two alternative promoters: the P1 promoter is located upstream the mogR start codon and produces a shorter and constitutive transcript, whereas the P2 promoter is σB-dependent and generates a longer anti0677 transcript that contains mogR and originates on the opposite strand to the coding sequence of the fliNPQ flagellum operon.Citation3 The expression of anti0677 leads to higher transcription of mogR and decreased levels of fliNPQ transcripts, in turn inducing a strong repression of flagellum genes and reduced Lm motility.Citation26
The third category of ncRNAs involved in Lm virulence embraces the trans regulatory RNAs (trans-ncRNAs), which are encoded in trans in relation to the genes they regulate, and are capable both to regulate multiple mRNAs playing with their translation and stability, and to interact with proteins.Citation123 Trans-ncRNAs can act either as antisense or sense RNAs, and this regulation may happen by two different ways: either the trans-ncRNA and its target mRNA are encoded on the same DNA locus, but transcribed in opposite directions leading to the formation of extended base-pairings or, the trans-ncRNA is encoded in a different location of the target mRNA, generating a weaker, short and imperfect complementarity with the mRNA, but allowing to control more than one target mRNA.Citation133-136 Lm displays several trans-ncRNA involved in its pathogenicity, namely LhrA, LhrC1–5, Rli33–1 (LhrC6), Rli22 (LhrC7), RliB, Rli47 (SbrE), Rli27, Rli31, Rli32, Rli38 and Rli50.Citation3
LhrA is a trans-ncRNA with a role in the transition from exponential to stationary phase of Lm growth, whose stability is dependent on the presence of the Hfq chaperoneCitation137 Transcriptomic studies clearly showed that lhrA inactivation alters the expression levels of more than 300 Lm genes, including the chiA gene that encodes Lm chitinase A important for Lm pathogenesis in mice, possibly by targeting host proteins involved in immune signaling.Citation27,Citation138,Citation139 Lm LhrA may modulate host immune response throughout infection by inducting interferon-beta (IFN-β) production.Citation28 In addition, the QS Agr system promotes chitinase activity through negative regulation of this short ncRNA lhrA, which itself binds to chitinase mRNA (chiA) blocking ribosome access and translation.Citation104 In turn, ChiA secretion by Lm downregulates the inducible nitric oxide synthase (iNOS) activity in infected mice, enhancing bacterial survival.Citation27 MouR, a transcriptional activator of the Lm Agr system, was also shown to repress the expression of lhrA.Citation103
trans-ncRNAs belonging to the LhrC family, including LhrC1–5, Rli33–1 and Rli22, were shown to be induced when Lm reaches the human bloodstream.Citation3 Such is the case of LhrC1–5, which is upregulated when bacteria are exposed to the heme group of the human hemoglobulin and positively activated by the response regulator LisR, part of the two-component system LisRK.Citation29,Citation30 Moreover, LhrCs were shown to repress, at the post-transcriptional level, different Lm virulence-associated genes (oppA, tcsA and lapB), acting by base pairing to the Shine-Dalgarno (SD) region, thus decreasing mRNA levels or inhibiting translation. This regulation by LhrC1–5 is a competitive advantage throughout human blood infection under heme-rich conditions. The downregulation of these surface exposed proteins prevents immune recognition and enhances bacterial survival within the host.Citation29–31 Additionally, in the presence of the β-lactam antibiotic cefuroxime, LhrC1–5 downregulates the expression of a heme oxygenase-like protein Lmo0484, by base paring to the ribosomal binding site and consequently inhibiting its translation. This repression was found to protect Lm against this cell wall-acting antibiotic.Citation29,Citation30 Other examples of ncRNAs belonging to the LhrC family and playing a role in Lm virulence are Rli33–1 and Rli22. The lack of rli33–1 has been found to significantly impair Lm survival within macrophages and virulence both in murine and insect models of infection, suggesting a critical role for this ncRNA to favor Lm persistence within host cells.Citation42 The expression of rli22 was found to be upregulated in bacteria inside the intestinal lumen of mice or upon bacteria exposure to cell envelope stress in a LisRK two-component system manner.Citation3,Citation36
Furthermore, there is a significant correlation between the ncRNA RliB and Lm virulence, as demonstrated by the upregulation of this ncRNA in key infection sites such as the mouse intestinal lumen and the blood.Citation3 RliB plays a role in the adhesion and invasion ability of Lm but mechanisms underlying its upregulation and functional role during infection require further research.Citation34
Rli47, also named SbrE, is a σ-dependent ncRNA highly induced in the intestinal lumen and in macrophages.Citation3,Citation42 In silico and in vivo analysis of SbrE structure reveal its role in binding the SD region of ilvA mRNA, encoding a threonine deaminase involved in the biosynthesis of branched-chain amino acids.Citation35 This interaction occludes the ilvA ribosome binding site, thus repressing its translation. Consequently, the threonine deaminase enzymatic activity is repressed and the initial step of the L-isoleucine biosynthetic pathway blocked. These observations highlight the role of SbrE in the isoleucine biosynthesis repression under harsh conditions, inhibiting Lm growth and impacting virulence.Citation35
Rli27, which is upregulated in the mouse intestinal lumen and in blood,Citation3 was implicated in the post-transcriptional regulation of lmo0514 during the infection process.Citation37 Lmo0514 is an internalin-like protein covalently linked to the bacteria cell wall by a LPXTG motif. It is upregulated during Lm colonization of eukaryotic cells in a PrfA and σB-independent manner,Citation140 and is required for bacterial survival in plasma, having a role in virulence at early infection stages.Citation141 Rli27 interacts with lmo0514 long 5’-UTR, inducing its translation and modulating the cell wall surface protein levels during intracellular infection. Within the eukaryotic niche, this regulation depends on the activity of two promoters of the target gene, generating a short and a long 5′-UTR transcripts, whose relative abundance varies from extra- to intra-cellular bacteria. Importantly, the sRNA binding site is only present in intracellular bacteria, once they are enriched with the long 5’-UTR version.Citation37
The transcription of both rli31 and rli32 ncRNAs increases significantly during Lm infection of macrophages.Citation42 These ncRNAs were also found to play a role in Lm virulence.Citation28,Citation38,Citation39 The absence of rli31 renders Lm more susceptible to the action of lysozyme, leading to a significant attenuation of Lm virulence upon intravenous mice infection. These findings highlight the critical role of Rli31 in protecting Lm from the enzymatic activity of lysozyme present in the bloodstream.Citation38 In addition, in vitro experiments confirmed that Rli31 is capable to bind to the 5’-UTR of spoVG mRNA, a regulator implied in lysozyme resistance, motility and virulence. However, the absence of rli31 does not impact spoVG mRNA or protein levels. Curiously, the absence of both Rli31 and SpoVG induces a contrary effect on the regulation of lysozyme resistance and virulence, probably implying an antagonistic regulatory relationship between Rli31 and SpoVG, that allows bacteria to fine-tune their growth in different environments.Citation39 Rli32, which also binds to SpoVG protein, was found to be secreted from bacteria to the host cytosol upon infection, triggering the expression of β-interferon.Citation28,Citation39 Additionally, the transcription of rli32 was found to be dependent on VirR, a transcriptional regulator of Lm virulence, suggesting that Rli32 plays a role in virulence and/or resistance to cell envelope stress underscoring its importance in bacterial adaptation and survival strategies.Citation40 The absence of rli32 leads to a decrease in Lm ability to survive within macrophages and promotes the downregulation of the ncRNA Rli60 and genes responsible for the tryptophan biosynthesis (lmo1627–33). Conversely, overexpression of rli32 favors Lm intracellular growth and renders the bacteria more susceptible to the action of cefuroxime. The Rli32 overexpression induces Lm resistance to H2O2-induced stress and also increases the expression of the ncRNAs LhrC1–4, genes encoding the ferrichrome ABC transport (lmo1958 and lmo1960), and the heme-binding proteins Hbp1 and Hbp2.Citation28 These intricate findings highpoint the multifaceted roles of Rli32 in Lm, impacting various cellular processes, including host interactions, stress responses, and antibiotic susceptibility.
Rli38 was shown to be associated with Lm colonization of mouse organs.Citation3,Citation41 This trans-ncRNA is capable to target mRNAs of three different genes, including the global iron uptake regulator Fur, related with bacterial adaptation in blood, the membrane associated lipoprotein Lmo0460 that belongs to the internalin family, and the ABC transporter ATP-binding protein Lmo2752. During an oral infection of mice, the absence of Rli38 attenuates Lm virulence.Citation3
Another example of a trans-ncRNAs impacting Lm virulence is Rli50 which exhibits higher transcription levels intracellularly. The absence of rli50 not only impairs the bacterium capacity to proliferate within macrophages but also results in virulence attenuation in both insects and mice.Citation28,Citation42 These findings highlight the role of Rli50 to promote Lm intracellular survival, pathogenicity and an adequate host immune response.
The last category of ncRNAs is composed by the cis-regulatory RNAs (cis-ncRNAs). Unlike trans-ncRNAs which can act on genes located at different genomic locus, cis-ncRNAs mostly exert their regulatory effects on the same genomic region where they are transcribed from. These ncRNAs can adopt two alternative RNA structures, leading either to transcription termination or transcription antitermination of downstream genes.Citation118,Citation120 Such is the case of rli60, a cis-ncRNA located upstream of an operon responsible for the biosynthesis of branched-chain amino acids (ilvD), that is transcribed whenever levels of these amino acids are limited.Citation32 Rli60 was implicated in Lm virulence in a mouse model, especially due to the capability of Lm to invade and proliferate within the liver, spleen and macrophages.Citation33 Interestingly, the absence of rli60 was correlated with increased adhesiveness to macrophages and decreased ability to form biofilms.Citation33,Citation142 It was also suggested that Lm Rli60 is able to sense the available branched-chain amino acids, that are essential for its optimal growth, downregulating downstream genes accordingly. This regulation favors bacterial pathogenicity by ensuring the proper levels of internal branched-chain amino acids, which are per se a signal for the CodY regulator to bind prfA, thus allowing the expression of a number of major Lm virulence factors.Citation32
Second messengers
Interpreting extracellular information also requires signal transduction across the bacterial cell membrane. Therefore, intracellular small molecules such cyclic dinucleotides were found to participate in gene transduction through the transmission of signals/stimulus to effector molecules.Citation143,Citation144 Importantly, second messenger systems can assimilate several sensory inputs modulating the microorganism response output.Citation143 Lm uses two major types of intracellular second messengers to modulate its virulence: the cyclic di-adenosine monophosphate (c-di-AMP) and the cyclic di-guanosine monophosphate (c-di-GMP) ().
Figure 2. The interplay between host nutritional signals and Lm signaling molecules in virulence. Within the host, Lm imports G6P via the PrfA-regulated permease Hpt, to support its intracellular growth.Citation79 Additionally, Lm uses host L-glutamine whose uptake is dependent on the ABC transporter GlnPQ, to further induce the expression of PrfA-controlled virulence genes. Lm is also capable to synthesize L-glutamine, even not being enough to induce virulence genes, in the presence of ammonia and glutamate by using the glutamate synthase GnlA.Citation77 the expression of prfA is also dependent on glutathione (GSH), which is synthesized from the host cytosol imported L-cysteine, via the glutathione synthase GshF. L-cysteine uptake is mediated by three different transport systems: the substrate-binding CtaP, the Opp transporter and the ABC transporter TcyKLMN. Once synthesized, GSH binds PrfA, inducing a conformational change that enables its binding to the the PrfA box within the promoter regions of PrfA-regulated genes, thereby activating their expression.Citation106 Importantly, prfA-transcription is enhanced in response to the low availability of branched-chain amino-acids (BCAAs) through the binding of CodY to its coding sequence.Citation73 Under high concentrations of BCAAs, CodY binds to isoleucine repressing the expression of both ilv-leu operon and purH gene, involved in BCAA and purine biosynthesis respectively, and also rli60. In contrast, isoleucine-unbound CodY can bind to DNA, allowing its own expression and inducing the expression of prfA and consequently PrfA-regulated genes. When challenged with low BCAA levels rli60 is transcribed forming a ribosome-mediated attenuator that restricts the expression of genes belonging to the ilv-leu operon, in turn increasing Lm virulence through CodY expression.Citation32 CodY-mediated regulation is also dependent on c-di-AMP levels. c-di-AMP is synthesized from two ATP molecules via DacA and degraded by PDEs, PdeA and PgpH, giving rise to pApA. High c-di-AMP concentrations induce the up-regulation of CodY regulon, via CbpB-mediated inhibition of RelA activity.Citation16 This inhibition ensures the maintenance of GTP levels derived from the degradation of c-di-GMP, that induce the expression of CodY.Citation145
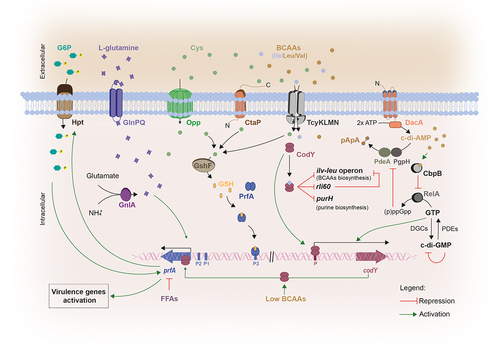
The synthesis of c-di-AMP is required for full Lm viability both in in vitro conditions and within the host.Citation15,Citation146 This process is catalyzed by a diadenylate cyclase, DacA, from two molecules of ATP and degraded by two phosphodiesterases (PDEs), PdeA and PgpH.Citation147 Mutants lacking the dacA gene have been found to exhibit reduced infection capability in a mouse model, increased susceptibility to cell wall antibiotics and inability to growth in rich media without salt supplementation.Citation146,Citation148,Citation149 Moreover, a decrease in c-di-AMPs levels upon DacA depletion or PDEs overexpression results in decreased bacterial replication into primary bone marrow-derived macrophages.Citation146 DacA has been associated with the induction of IFN-β expression during infection, triggering the innate immune response thereby leading to the activation of host cellular defense mechanisms to control infection.Citation150 The c-di-AMP binding protein CbpB acts as a c-di-AMP sensor and activates the synthesis of the stringent response regulator (p)ppGpp (guanosine penta-phosphate) by binging to the bifunctional (p)ppGpp synthetase/hydrolase RelA. High c-di-AMP concentrations preventes RelA activation by binding and sequestering CbpB. (p)ppGpp is also a second messenger that binds and inhibits c-di-AMP phosphodiesterases, resulting in an increase in c-di-AMP. CodY is a pleiotropic transcriptional regulator that represses nutrient uptake and amino acid biosynthesis genes in response to the levels of GTP and branched-chain amino acids. c-di-AMP appears essential to regulate (p)ppGpp levels because accumulated (p)ppGpp altered GTP concentrations, thereby inactivating CodY.Citation15,Citation16 c-di-AMP was also shown to bind and regulate the enzyme pyruvate carboxylase allowing bacterial proliferation and subsistence within the host.Citation17
c-di-GMP synthesis is accomplished by diguanylate cyclases (DGCs) from two GTP molecules. Beyond being degraded by PDEs, c-di-GMP is able to inhibit its own expression by binding itself to its inhibitory site in order to avoid an excessive GTP consumption.Citation151-153 Low c-di-GMP levels were found to be required for bacteria to induce an acute infection, while high levels of c-di-GMP are necessary for a long-term host colonization (chronic infections).Citation154 By binding to effector molecules, c-di-GMP regulates several cellular processes including virulence, QS systems, cell morphology, differentiation, cell–cell communication, exopolysaccharide matrix production, flagellum biosynthesis and pilus assembly.Citation155-158 Specifically, for its activity at the gene expression level, c-di-GMP interacts with RNAs such as riboswitches and DNA-binding proteins.Citation159 In Lm, it was demonstrated that high c-di-GMP levels play a negative role on virulence, by inducing the synthesis of an exopolysaccharide that was associated with motility inhibition, cell aggregation and enhancement of tolerance to disinfectants and desiccation. Moreover, high levels of c-di-GMP were found to alter the expression of internalin genes by downregulating the entire virulence regulon, including the master regulator PrfA, thus diminishing not only enterocyte invasiveness in vitro but also the bacterial load in the liver upon oral mice infection.Citation145,Citation158 It was proposed that elevated c-di-GMP levels induce lower GTP levels, decreasing the activity of CodY, the coordinator of metabolism and virulence, in turn suppressing Lm virulence via decreased PrfA expression.Citation145
Additionally, the degradation of both c-di-AMP and c-di-GMP can result on the formation of two linear dinucleotides, pApA and pGpG, respectively, that are important products of the RNA metabolism.Citation151,Citation160,Citation161 Both molecules can also be hydrolyzed by the oligoribonucleotidase NrnA, generating the mononucleotides AMP and GMP, respectively. Concomitantly, the nrnA gene was also found to be up-regulated during Lm biofilms formation.Citation162
Two-component systems
Beyond the signaling molecules previously discussed, Lm employs other regulatory mechanisms that contribute to establish infection. Among them, two-component transduction systems (TCSs) stand as paramount players enabling bacteria adaptation to the conditions encounter within the host. These bacterial systems are typically composed by a transmembrane sensor histidine kinase and a cytoplasmic response regulator, both working together to sense and process external stimuli including nutrients, metal ions, host-derived metabolites and gut specific conditions. External cues usually trigger the activation and autophosphorylation of the histidine kinase, and the phosphoryl group is transferred to the cognate response regulator, which in turn is activated to work as a transcription factor that regulates gene expression.Citation163,Citation164 So far, among the 14 TCSs of Lm, four have been related to its pathogenicity: CesRK, LisRK, ViRS, and PieRS ().
Figure 3. Schematic representation of the two-component transduction systems governing virulence gene expression in Lm.
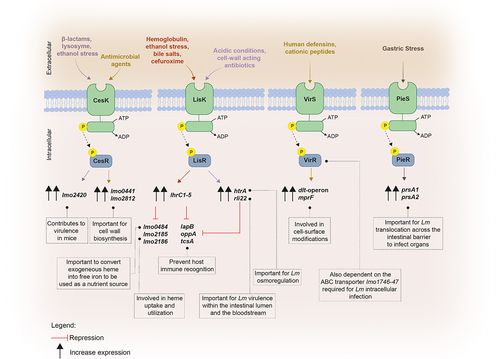
CesRK, which is composed by the cephalosporin sensitivity response regulator CesR and its sensor histidine kinase CesK, is implicated in sensing and responding to changes in the cell wall integrity and in the expression of cell envelope-related genes.Citation43,Citation44 In the absence of cesR, Lm becomes less virulent in mice upon intragastric infection, but not after intraperitoneal inoculation, thus suggesting a prime role for CesRK TCS in Lm pathogenicity.Citation43 Furthermore, CesRK is capable to sense not only the presence of β-lactam antibiotics typically used to treat Lm infections, but also ethanol and lysozyme, further inducing the transcription of the downstream small open reading frame orf2420 (lmo2420), also important for bacterial pathogenesis.Citation43 Lm CesK perceives cell wall damages caused by antimicrobial agents, generating a faster response by the bacteria that activate a cascade of events that culminate in the upregulation of key genes involved in cell wall biosynthesis, such as lmo0441 and lmo2812.Citation44
LisRK was found to be implicated in the transcription regulation of the htrA gene, that encodes a HtrA-like serine protease involved in Lm osmoregulation and pathogenesis,Citation45 and conferring protection against some β-lactams antibiotics such ampicillin.Citation46,Citation47 It was suggested that Lm LisRK is able to sense perturbations in the surrounding environment, such as the acidic conditions or the presence of cell wall-acting antibiotics.Citation45 LisRK also plays a role in the invasion capacity of the pathogen, mutant bacteria becoming significantly less virulence upon mice infection.Citation165,Citation166 Additionally, this TCS together with the trans-ncRNAs LhrC1–5, are important for intracellular replication of Lm into macrophage-like cells.Citation31
The VirRS system, which composes another TCS, acts as a positive transcriptional regulator of genes mainly involved in cell surface modifications, such as the dlt-operon and mprF, which are crucial for bacteria to fight human defensins and cationic peptides.Citation48 The absence of the virR gene is associated with the impairment of Lm cell invasion and infection capacity in mice. Interestingly, VirR activation was shown to be not totally dependent on its cognate kinase, since it also depends of an ABC transporter encoded by the genes lmo1746–47 shown to be required for optimal intracellular infection and virulence.Citation40,Citation48 Contrarily to others TCS where both genes encoding the sensor and the response regulator are contiguous on the chromosome, virR and virS are not adjacent but separated by three genes. Likewise, VirS appears to interact with other response regulators, regulating genes that are not affected by the absence of virR, thus suggesting a non-exclusive interaction between VirS and VirR.Citation48
The TCS PieRS plays a role in promoting Lm translocation across the intestinal barrier by regulating the secretion of two chaperones, PrsA1 and PrsA2, both found to be associated to the membrane and secreted in the extracellular medium.Citation49 PrsA2 plays a significant role in hemolytic and phospholipase activity, being important for Lm cell-to-cell spread and full virulence following both oral and intravenous mice infection,Citation49,Citation50,Citation167–170 while PrsA1 is required for Lm traffic from the intestine to the liver.Citation49 It was proposed that when Lm is faced with harsh conditions, such the gastric stress found in the gastrointestinal tract, PieS, the sensor kinase, triggers the activation of the response regulator PieR, leading to the activation of both prsA1 and prsA2, thus enabling bacterial survival and crossing the intestinal barrier to infect target organs.Citation49
Glutathione
In an attempt to mediate Lm transition to a pathogenic state, it can use the tripeptide glutathione (GSH) as a metabolic signaling molecule modulating its virulence (). This process relies on the allosteric binding of GSH to the major Lm transcriptional regulator PrfA, inducing a conformational change on PrfA and therefore its binding to DNA. Consequently, virulence genes regulated by PrfA are expressed, promoting infection.Citation18-20 Within bacteria, GSH levels are very limited.Citation63 Even so, Lm is able to synthesize GSH by making use of its glutathione synthase (gshF).Citation18,Citation64 GshF is a bifunctional enzyme able to synthesize a GSH intermediate, γ-glutamylcysteine, by binding the γ-carboxyl group of L-glutamate to L-cysteine, previously imported from the surrounding environment since Lm is not able to synthesized it. Subsequently, GshF mediates the fusion of this intermediate product with glycine to yield GSH (also known as L-γ-glutamyl-L-cysteinylglycine).Citation64,Citation171 On the other hand, inside mammalian cells Lm encounters abundant concentrations of GSH being able to import and use it as a signaling molecule to regulate the expression of virulence genes. Curiously, the host-synthesized GSH cannot completely replace the GSH produced by the bacteria itself to activate PrfA and PrfA-dependent genes. Even though Lm strain defective for gshF do not suffer a general loss of fitness, it exhibits a decrease in the expression levels of some virulence factors, such as the actin assembly-inducing protein ActA required for Lm intracellular motility, suggesting that extracellular GSH could function as a signal for bacteria into the host intracellular niche.Citation18,Citation172 Importantly, L-cysteine presents itself an amino acid rate-limiting in the synthesis of GSH, directly affecting the expression of prfA and PrfA-controlled genes, hence limiting Lm virulence.Citation171,Citation173 Three different transport systems were already described as being involved in the acquisition of cysteine for GSH biosynthesis: (i) CtaP, a substrate-binding component of a transport system for L-cysteine (ii) the OppABCDF transporter, capable to import exogenous cysteine-containing peptides and (iii) the ABC transporter TcyKLMN capable to collect both cysteine and its reduced form cystine from the extracellular medium.Citation63,Citation65,Citation174 Even being all implicated in the bacteria virulence, only the TcyKLMN system appears to be linked to Lm growth within the host cytosol by supplying L-cysteine for GSH synthesis.Citation63 The TcyKLMN system is controlled by three metabolic regulators CodY, CymR and CysK. When faced with limited availability of branched-chain amino acids, Lm up-regulates the TcyKLMN system to uptake cysteine from the extracellular medium, thereby promoting GSH biosynthesis and consequently PrfA activation.Citation63 A gshF mutant was shown to have a defect in growth in vitro, in the presence of diamide and copper ions stress. Moreover, it was shown that gshF is required for efficient invasion and proliferation both in macrophages and mice organs. GshF plays a role in Lm response to oxidative stress, inhibiting the transcription of the lmo1997-lmo2004 operon, that encodes a phosphoenolpyruvate-carbohydrate phosphotransferase system, being lmo2002 the most critical gene to resist to oxidative stress.Citation175
Additionally, Lm is able to use inorganic sulfur thiosulfate and H2S as L-cysteine sources, supporting its growth and virulence. This process occurs via the Lm two-step biosynthetic pathway of L-cysteine, that involves the conversion of L-serine into L-cysteine by the CysE/CysK enzymes.Citation176 Despite these observations, the mechanism by which Lm imports thiosulfate remains unclear.
Furthermore, the toxic by-product of cellular metabolism methylglyoxal, produced by both the host and the bacteria, was recently found to be associated with PrfA activation since it functions as a host cue for Lm to produce GSH. Lm displays a GSH-dependent methylglyoxal detoxification system composed by two enzymes, GloA (glyoxalase A) and GloB (glyoxalase B) that mediate the detoxification of methylglyoxal by conjugation with glutathione. During Lm growth within activated macrophages, it faces higher levels of methylglyoxal, which consequently triggers the activation of gshF, therefore resulting in increased levels of bacteria GSH and PrfA activation.Citation177
Host nutritional and physical signals triggering Listeria monocytogenes virulence
Environmental cues signaling the colonization of the bacteria into the host are implicated in the regulation of bacterial virulence factors. Among them, the host temperature, pH, iron availability, bile salts, nutrients and the oxidative stress play key roles on how Lm fine-tunes gene expression and protein translation.Citation178 This section will focus on the impact of these external signals in Lm pathogenesis.
Temperature
Temperature increase is sensed by bacteria when they shift from the external environment to mammalian hosts. Many bacteria are thermally regulated and thus may alter the expression of their virulence genes to promote infection.Citation179 Moreover, the temperature may not only influence the DNA topology, RNA structure and metabolism, as it may impact the activity and processing of proteins.Citation178
For Lm, the temperature increase to 37°C within the host is a signal to activate the expression of the PrfA thermosensor, triggering the expression of a number of major virulence genes (). At temperatures below 37°C, the mRNA 5’-UTR of prfA forms a secondary structure which masks the ribosome binding region, repressing the translation of the downstream mRNA. The temperature increase to 37°C makes impossible the formation of this inhibitory RNA structure, inducing the expression of prfA and PrfA-controlled virulence genes.Citation130,Citation180 Moreover, the temperature rise also triggers a conformational shift in the anti-repressor GmaR, allowing the expression of MogR and consequently, the repression of genes involved in flagellar-based motility (). While flagellar motility is indispensable for bacterial survival in the environment for nutrient acquisition and surface colonization, within the host, flagella is highly immunogenic working as a signal that activates the immune system, and thus detrimental for the virulence.Citation51,Citation52
pH
After entering the human body, Lm encounters resistance against colonization imposed either by the harsh conditions of the gastrointestinal tract or by the host immune system.
Acidic environments trigger the activation of the Lm stressosome, a stress sensor that induces a general adaptative stress response crucial to confer acid resistance.Citation53 Lm stressosome is a dynamic signaling multi-protein complex composed by RsbR1 (lmo0889) and its paralogues RsbR2 (lmo0161), RsbR3 (lmo1642), RsbR4 (lmo1842) and RsbL (lmo0799), the scaffold protein RsbS and the serine – threonine kinase RsbT. Stressosome activation is achieved by RsbR1 and RsbS that sense and transduce the acid stress signals. This sensing is dependent on the phosphorylation of RsbR1 (at residue T209) and RsbS (at residue S56), both mediated by the RsbT kinase. Subsequently, RsbT is release from the stressosome and interacts with the RsbU phosphatase, which consequently promotes the dissociation of the anti-sigma factor RsbW from σβ. These events culminate in the upregulation of genes involved in the general stress response and infectivity, thus favoring the host invasion.Citation53-56 Moreover, the activation of σβ by the stressosome is also required for the activation of the glutamate decarboxylase enzyme gadD3, that converts L-glutamate into γ-aminobutyrate (GABA) while consuming an intracellular proton, and of the arginine (arcA) and agmatine (aguA1) deiminases, which metabolize L-arginine into citrulline and ammonia to adjust the cytoplasmic pH.Citation54,Citation181 During the first process, the consumption of one proton itself is enough to increase the intracellular pH thus attenuating acidic stress.Citation182 GadD3 together with GadD1 and GadD2 enzymes and the glutamate/GABA antiporters (GadT1 and T2) form the glutamate decarboxylase (GAD) system which is key to maintain Lm pH homeostasis in acidic environments.Citation57,Citation183,Citation184 The GAD system comprises two subsystems that differ depending on the location of the required glutamate: the first involves the uptake of extracellular glutamate by antiporters, which undergoes subsequent decarboxylation resulting in the production of GABA. Subsequently, GABA is exporter back by the same antiporter mechanism while more glutamate is being imported; for the second subsystem, Lm uses and processes intracellular glutamate to regulate its intracellular pH, in a process dependent on GadD3 and GadD1.Citation184,Citation185 GAD is also involved in Lm survival under oxidative stress, namely by conferring resistance to hydrogen peroxide.Citation58
Macrophages are capable to recognize and phagocytose the bacteria, engulfing them into intracellular vacuoles termed phagosomes. When cells are infected by Lm, the phagosome where the bacterium is contained acidifies to a pH around 5.5 to 6. This acidification works as a switch to induce listeriolysin O (LLO) pore-forming activity per se mediating the vacuolar disruption and allowing bacteria release from the phagosome and spread.Citation59-62 The cytolytic activity of LLO involves the binding and subsequently oligomerization of LLO monomers within the intracellular vacuole membrane leading to the formation of pores and consequently vacuole disruption. Importantly, LLO activity is intricately linked to the pH environment and controlled by an acidic triad present in the transmembrane domain (D3) of LLO that functions as pH sensor. In stark contrast to the acidic environment encountered within the phagosome, inside the host cell cytosol Lm faces neutral pH that destabilizes the D3 domain of LLO promoting an inactivation of LLO by the formation of irreversible denatured monomers. This dual-regulation mechanism highlights the sophisticated interplay between pH dynamics and the functional modulation of LLO in the context of Lm infection.Citation61,Citation186,Citation187 Importantly, the pH-dependency also avoids uncontrolled LLO activity and consequently its insertion into the host cell membranes that would leave the bacteria constantly exposed to extracellular threats.Citation62
Iron
Iron, a vital element for the growth of almost all living cells, is involved in important cellular functions such as the transport and storage of oxygen, and plays a role on the bacteria toxicity and pathogenesis.Citation188-190 Although this element is abundant in the environment, the available free iron in the host is restricted. Therefore, the adaptation to shifts between high and low free iron environment is an important signal for bacteria to regulate their gene expression.Citation189 Lm needs iron for growth and survival during infection namely to establish itself in the gastrointestinal tract.Citation191 To acquire iron, Lm displays different mechanisms involving the use of iron reductases and ferric citrate uptake systems. Additionally, Lm can use siderophore like-uptake systems.Citation192,Citation193 Moreover, the host-derived heme found in hemoglobulin and other hemoproteins, also represents an important source for iron and can be used as metabolic cofactor for many bacteria including Lm.Citation82,Citation192 Into the host cytoplasm, Lm may use iron to support its growth and proliferation and to be protected from the ROS-mediated damage. Furthermore, to mitigate ROS-mediated damage in phagosomes, Lm may be also able to sequester iron.Citation80,Citation81 Some studies have reported Lm ability to use the iron available to assess its location and regulate its behavior in term of gene expression, accordingly. In the presence of higher iron concentrations found in the human gut,Citation194 the invasins InlA and InlB (both required for the invasion of non-phagocytic cells including intestinal epithelial cells)Citation195 are positively regulated, probably to enhance Lm internalization into intestinal cells.Citation83,Citation84 Contrary, when bacteria are under iron starvation conditions, the expression of both LLO and ActA is increased for Lm to escape from the vacuole, reach the host cytosol, and spread to neighboring cells.Citation83,Citation84,Citation196 Additionally, when Lm encounters an heme rich environment, it induces the upregulation of FrvA, a specific Fe(II) exporter ATPase part of the PerR (a peroxide-inducible stress response regulator) and Fur (a ferric uptake regulator) regulons.Citation85 The absence of frvA induces a significant attenuation of virulence in mice.Citation86
Lm is also using heme as a host-derived signaling molecule to control the expression of the Listeria adhesion protein (LAP)Citation82 a virulence factor that induces intestinal epithelial barrier dysfunction to promote Lm translocation.Citation197
Bile Salts
During the initial infection stage, Lm encounters bile salts in the gastrointestinal tract using them as signals to ascertain its location and to adapt to the surrounding environment.Citation67 Bile salts are synthesized in the liver, using cholesterol as precursor, being then conjugated with glycine or taurine and released into the small intestine from the gallbladder.Citation198 About 10% of the Lm genome was found to be regulated by bile. Among them, the bile sensor BrtA was found to be induced.Citation67 The bile salt hydrolase bsh is expressed when Lm is in the gastrointestinal tract to promote bile salts resistance catalyzing the hydrolysis of glycodeoxycholate (GDCA) and taurodeoxycholate (TDCA) conjugated bile salts into cholic acids ().Citation66 Consequently, BrtA exposure to cholic acids inhibits BrtA binding to the promoter of the multi-drug efflux pump mdrT, inducing MdrT expression and allowing the export of cholic acids.Citation67 Intriguingly, the overexpression of mdrT can be detrimental for Lm virulence in vivo,Citation68 highlighting the importance of Lm in controlling the bsh expression levels in the course of the infection. In this sense, Lm uses the transcriptional repressor CadC to repress bsh expression at late infection stages, avoiding the detrimental overexpression of mdrT during this step of the infection.Citation4,Citation69 The efflux pump MdrT is also capable to transport c-di-AMP,Citation199 inducing a type I IFN-β response which enhances the susceptibility to Lm infection,Citation200–202 reinforcing the importance of mdrT regulation by CadC.
Peptides
Bacterial pathogenicity is significantly influenced by the availability and acquisition of nutrients from the host environment. Different Lm auxotrophic mutants for several nutrients including uracil, phenylalanine, glycine, proline or nicotinic acid were found to retain full virulence, suggesting that Lm can acquire these nutrients from the host environment. Interestingly, these strains seem to be able to utilize other nutrient sources such as peptides.Citation203 Additionally, Lm is capable of exploiting exogeneous oligopeptides, an ubiquitous source of organic nitrogen, to sense the environment and control PrfA activity. Indeed, PrfA activation has been shown to be dependent on the presence of cysteine containing peptides (Cys/Cys-peptides) in the host environment, while being antagonized by oligopeptides lacking cysteine, such as leucine.Citation65,Citation78,Citation204 While the activation of PrfA requires occupancy of both GSH sites in the PrfA dimer, nonspecific peptide binding (such as leucine dipeptide) to one monomer is enough to change the symmetry of the two helix-turn-helix motifs, impairing DNA binding and consequently, the expression of virulence genes.Citation65
Branched-chain amino acids (BCAAs)
The availability of branched-chain amino acids (BCAAs), including isoleucine, leucine, and valine, constitutes another important metabolic signal sensed by Lm (). BCAAs represent an important source of nutrients for bacteria and are crucial to remodel their virulence gene expression, protein synthesis and to control bacterial adaptation to amino acid starvation.Citation70 The response to BCAAs is dependent of CodY, a Lm BCAA-responsive transcriptional regulator capable to directly bind isoleucine, inducing a conformational change that promotes binding to the DNA.Citation71,Citation72 This mostly happens under high concentrations of BCAAs, where CodY acts as a repressor of ilvC and purH genes, involved in BCAAs and purine biosynthesis, respectively.Citation32,Citation70 Interestingly, within mammalian cells, where BCAAs are deprived (in particular isoleucine), isoleucine-unbound CodY is capable to activate prfA and therefore, PrfA-regulated virulence genes, by directly binding to the first 15 nucleotide of the prfA-coding sequence. This suggests an additional functional conformation for CodY in such conditions and thus supports the idea that the gene regulation by CodY depends on its affinity to different sites and the levels of BCAAs available.Citation70,Citation73 To this end, rli60, a ncRNA encoding a ribosome-mediated transcription attenuator, cis-regulates downstream genes involved in BCAA biosynthesis. Under BCAAs limiting conditions, as occurring during infection, the ncRNA rli60 is transcribed creating a ribosome-mediator attenuator that restricts the expression of the ivl-leu operon and consequently the production of BCAA. This BCAA starvation allows Lm to control the internal levels of isoleucine thereby promoting virulence gene expression via CodY.Citation32
Free fatty acids (FFAs)
After ingestion, Lm needs to overcome different metabolomic landscapes throughout the mammalian gastrointestinal tract, with different metabolites produced either by the host itself or by commensal microorganisms or other microbial agents. The ability to sense and respond to these gut metabolites is therefore central for the successful colonization, since it allows bacteria to efficiently regulate gene expression accordingly. Free fatty acids (FFAs) are highly present in the gut metabolome, being important nutrient sources, not only for the resident microbiota but also for bacterial pathogens to signal gene expression by regulating DNA binding activity of virulence-related transcriptional factors.Citation205 Furthermore, FFAs act as antimicrobial agents displaying a bacteriostatic or bactericidal activity mainly by targeting the cell membrane.Citation206 In Lm it was found that the presence of long- or medium- FFAs impairs PrfA-dependent genes activation (). FFAs are capable to bind PrfA, inhibiting its DNA binding activity, and preventing the activation of PrfA-regulated virulence genes.Citation74,Citation75
Non-dependent phosphotransferase system host-derived sugars
The host intracellular environment displays different carbon sources that can be used by Lm. To import and use these carbon sources, Lm has evolved a non-dependent phosphotransferase system (non-dependent PTS) that allow the bacteria to make use of host phosphorylated sugars independently of the PTS system itself, thus offering a more efficient way for sugar utilization.Citation207,Citation208 These carbon sources include hexose phosphates, such as glucose-1-phosphate (G1P), glucose-6-phosphate (G6P), fructose-6-phosphate (F6P) and mannose-6-phosphate (M6P), and glycerol, being all signals sensed and rapidly metabolized by Lm to improve fitness and growing into the host.Citation79
G1P is mainly available intracellularly within the host, as a result of the primary degradation of glycogen.Citation209 This sugar can be used by Lm intracellularly as a growth substrate, under the positive regulation by PrfA and therefore co-expressed with PrfA-regulated genes in response to diverse host signals.Citation78 Beyond G1P, Lm is also capable to proficiently import host produced G6P by using Hpt, a non-PTS permease, whose expression is tightly regulated by PrfA (). The lack of Htp impairs Lm intracytosolic replication and proliferation within mammalian cells, and survival in mice.Citation79
Additionally, glycerol can be imported by the Lm uptake facilitator GlpF, sustaining its bacterial growth within the host cytosol. In the presence of glycerol, GlpF facilitates its transport across the bacterial cell membrane to be posteriorly metabolized by GlpK, a glycerol kinase that phosphorylates glycerol to produce glycerol-3-phosphate. The resulting product undergoes processing by DhaK kinase, to produce dihydroxyacetone phosphate (DHAP),Citation210 which is involved in the glycolysis and gluconeogenesis processes.Citation211 Furthermore, the use of glycerol not only provides an advantageous available source of energy, but also controls Lm virulence through the upregulation of PrfA-controlled virulence factors such InlC, LLO and ActA, crucial for the bacteria to invade and spread within host cells.Citation210
By contrast, other carbon sources such cellobiose and glucose, typically found outside the host, are transported by phosphoenolpyruvate-dependent phosphotransferase systems and may work as signals for Lm sensing the surrounding environment, where the PrfA-dependent gene expression is no longer required.Citation212–214
L-glutamine
L-glutamine, an amino acid abundant in mammalian serum and cells, is used by Lm as a nitrogen source and was found to be involved in Lm virulence modulation ().Citation76,Citation77 Lm can sense the presence of extracellular L-glutamine, using the ABC transporter GlnPQ for its recognition and uptake, GlnP being a substrate binding transmembrane protein, and GLnQ an ATPase.Citation77 In the presence of external high concentrations of L-glutamine, GlnPQ senses L-glutamine, inducing the expression of virulence genes (including hly, plcA, plcB and actA), and promoting macrophage and mouse infection.Citation77 Lm has the ability to synthetize L-glutamine itself when it access to both ammonia and glutamate (via glutamate synthase, GnlA).Citation76 Yet, the resulting L-glutamine is not enough to induce virulence genes expression. This highlights the ability of Lm to distinguish between bacterial or host signals, thus controlling its own localization within the host.Citation77
Reactive oxygen species
Within the human body, bacteria must cope with reactive oxygen species (ROS), such as superoxide (O2−), hydroxyl radicals (HO) and hydrogen peroxide (H2O2). Once exposed to ROS, Lm can activate specific regulatory pathways that contribute to its survival and virulence within the host. A regulator involved in ROS sensing and gene expression is the transcriptional factor PerR (peroxide-inducible stress response regulator). PerR represses the expression of genes involved in response to peroxide stress and metal homeostasis, including kat (hydrogen peroxide decomposition), fur (iron homeostasis regulator), hemA (heme biosynthesis), fri (iron-binding protein) and fvrA (iron efflux pump). The absence of perR is related with an increased Lm sensitivity to H2O2 in vitro, and decreased virulence in vivo. This strongly suggests the importance of PerR to both routine peroxide detoxification and response to ROS stress.Citation87
ROS generated by activated macrophages, which are fundamental to control Lm infection, seem to have also a role in the activation of Lm virulence genes prfA and hly. In activated macrophage-like cells, hly expression is enhanced. This increased hly expression can be abolished by the addition of superoxide dismutase and catalase, suggesting a role for reactive oxygen intermediates to control hly and prfA mRNAs levels.Citation88 Moreover, mutations leading to the constitutive activation of prfA enhance resistance to oxidative stress. Interestingly, the Lm resistance to the oxidative stress seems to be dependent on bacterial density.Citation89 Lm appears thus to be well equipped for both redox sensing and survival during the course of host infection.
Conclusions and Future Directions
The regulation of virulence factors in the intracellular pathogen Lm occurs in a complex and finetuned process, orchestrated by a network of regulatory systems that are able to sense and respond to various signals. These not only include signals secreted by Lm itself to promote its fitness and virulence, but also host cues that allow it to recognize the surrounding environment and modulate gene expression accordingly, to support its survival within the host and to facilitate the host colonization. The interplay between Lm signaling molecules and the host signals is also necessary to develop a proper response and to adapt to the different host environments.
Importantly, some of these signals that command virulence factors are common to several bacteria, both Gram-positive and Gram-negative. Therefore, deeply knowing and exploring these signals is certainly useful to modulate the expression of bacterial pathogen mechanisms throughout infection. Virulence genes can be essential and thus activated at specific host infection stages to promote virulence, but detrimental for infection at certain point. Furthermore, virulence factors are not expressed homogenously among the entire bacterial population, which per se also constitute a mechanism to optimize infection capacity. Controlling the signals behind these strategies can allow us to modulate pathogenesis, playing with the regulation of virulence genes in time and space, during infection.
Unravelling the bacterial and host signals controlling Lm virulence mechanisms facilitates the understanding of its pathogenesis and paves the way for the identification of new potential targets and the design next-generation therapeutic approaches to control Lm virulence.
Disclosure statement
No potential conflict of interest was reported by the author(s).
Additional information
Funding
References
- European Food Safety Authority and European Centre for Disease Prevention and Control (EFSA and ECDC). European centre for disease, and control, the european union one health 2022 zoonoses report. Efsa J. 2023;21(12):e8442.
- Lecuit M. Listeria monocytogenes, a model in infection biology. Cell Microbiol. 2020;22(4):e13186. doi:10.1111/cmi.13186.
- Toledo-Arana A, Dussurget O, Nikitas G, Sesto N, Guet-Revillet H, Balestrino D, Loh E, Gripenland J, Tiensuu T, Vaitkevicius K, et al. The Listeria transcriptional landscape from saprophytism to virulence. Nature. 2009;459(7249):950–27. doi:10.1038/nature08080.
- Camejo A, Buchrieser C, Couvé E, Carvalho F, Reis O, Ferreira P, Sousa S, Cossart P, Cabanes D. In vivo transcriptional profiling of Listeria monocytogenes and mutagenesis identify new virulence factors involved in infection. PLOS Pathog. 2009;5(5):e1000449. doi:10.1371/journal.ppat.1000449.
- Riedel CU, Monk IR, Casey PG, Waidmann MS, Gahan CGM, Hill C. AgrD-dependent quorum sensing affects biofilm formation, invasion, virulence and global gene expression profiles in Listeria monocytogenes. Mol Microbiol. 2009;71(5):1177–1189. doi:10.1111/j.1365-2958.2008.06589.x.
- Miller MB, Bassler BL. Quorum sensing in bacteria. Annual review of microbiology. Annu Rev Microbiol. 2001;55(1):165. doi:10.1146/annurev.micro.55.1.165.
- Garmyn D, Gal L, Lemaître J-P, Hartmann A, Piveteau P. Communication and autoinduction in the species Listeria monocytogenes: A central role for the agr system. Commun Integr Biol. 2009;2(4):371–374. doi:10.4161/cib.2.4.8610.
- Lee YJ, Wang C. Links between S-adenosylmethionine and Agr-based quorum sensing for biofilm development in Listeria monocytogenes EGD-e. Microbiol Open. 2020;9(5):e1015.
- Winkler WC, Nahvi A, Sudarsan N, Barrick JE, Breaker RR. An mRNA structure that controls gene expression by binding S-adenosylmethionine. Nat Struct Biol. 2003;10(9):701–707. doi:10.1038/nsb967.
- Loh E, Dussurget O, Gripenland J, Vaitkevicius K, Tiensuu T, Mandin P, Repoila F, Buchrieser C, Cossart P, Johansson J. A trans-acting riboswitch controls expression of the virulence regulator PrfA in Listeria monocytogenes. Cell. 2009;139(4):770–779. doi:10.1016/j.cell.2009.08.046.
- McDaniel BA, Grundy FJ, Artsimovitch I, Henkin TM. Transcription termination control of the S boxsystem: direct measurement of S-adenosylmethionineby the leader RNA. Proc Natl Acad Sci USA. 2003;100(6):3083–3088. doi:10.1073/pnas.0630422100.
- Challan Belval S, Gal L, Margiewes S, Garmyn D, Piveteau P, Guzzo J. Assessment of the roles of LuxS, S-ribosyl homocysteine, and autoinducer 2 in cell attachment during biofilm formation by Listeria monocytogenes EGD-e. Appl Environ Microbiol. 2006;72(4):2644–2650. doi:10.1128/AEM.72.4.2644-2650.2006.
- Rieu AL, Weidmann S, Garmyn D, Piveteau P, Guzzo J. agr system of Listeria monocytogenes EGD-e: Role in adherence and differential expression pattern. Appl Environ Microb. 2007;73(19):6125–6133. doi:10.1128/AEM.00608-07.
- Xayarath B, Alonzo III F, Freitag NE. Identification of a peptide-pheromone that enhances listeria monocytogenes escape from host cell vacuoles. PloS Pathog. 2015;11(3):e1004707. doi:10.1371/journal.ppat.1004707.
- Whiteley AT, Pollock AJ, Portnoy DA. The PAMP c-di-AMP Is essential for Listeria monocytogenes growth in rich but not minimal media due to a toxic increase in (p)ppGpp. Cell Host & Microbe; 2015;18(1).
- Peterson BN, Young MKM, Luo S, Wang J, Whiteley AT, Woodward JJ, Tong L, Wang JD, Portnoy DA. (p)ppGpp and c-di-AMP homeostasis is controlled by CbpB in Listeria monocytogenes. mBio. 2020;11(4).
- Sureka K, Choi P, Precit M, Delince M, Pensinger DA, Huynh T, Jurado A, Goo Y, Sadilek M, Iavarone A, et al. The cyclic dinucleotide c-di-AMP is an allosteric regulator of metabolic enzyme function. Cell. 2014;158(6):1389–1401. doi:10.1016/j.cell.2014.07.046.
- Reniere ML, Whiteley AT, Hamilton KL, John SM, Lauer P, Brennan RG, Portnoy DA. Glutathione activates virulence gene expression of an intracellular pathogen. Nature. 2015;517(7533):170–173. doi:10.1038/nature14029.
- Hall M, Grundström C, Begum A, Lindberg MJ, Sauer UH, Almqvist F, Johansson J, Sauer-Eriksson AE. Structural basis for glutathione-mediated activation of the virulence regulatory protein PrfA in Listeria. Proc Natl Acad Sci USA. 2016;113(51):14733–14738. doi:10.1073/pnas.1614028114.
- Wang Y, Feng H, Zhu Y, Gao P. Structural insights into glutathione-mediated activation of the master regulator PrfA in listeria monocytogenes. Protein Cell. 2017;8(4):308–312. doi:10.1007/s13238-017-0390-x.
- Mellin JR, Koutero M, Dar D, Nahori MA, Sorek R, Cossart P. Riboswitches. Sequestration of a two-component response regulator by a riboswitch-regulated noncoding RNA. Science. 2014;345(6199):940–943.
- Mellin JR, Tiensuu T, Bécavin C, Gouin E, Johansson J, Cossart P. A riboswitch-regulated antisense RNA in Listeria monocytogenes. Proc Natl Acad Sci USA. 2013;110(32):13132–13137. doi:10.1073/pnas.1304795110.
- Vasquez L, Parra A, Quesille-Villalobos AM, Gálvez G, Navarrete P, Latorre M, Toro M, González M, Reyes-Jara A. Cobalamin cbiP mutant shows decreased tolerance to low temperature and copper stress in Listeria monocytogenes. Biol Res. 2022;55(1):9. doi:10.1186/s40659-022-00376-4.
- Anast JM, Bobik TA, Schmitz-Esser S. The cobalamin-dependent gene cluster of listeria monocytogenes: implications for virulence, stress response, and food safety. Front Microbiol. 2020;11:601816. doi:10.3389/fmicb.2020.601816.
- Johansson J, Mandin P, Renzoni A, Chiaruttini C, Springer M, Cossart P. An RNA thermosensor controls expression of virulence genes in listeria monocytogenes. Cell. 2002;110(5):551–561. doi:10.1016/S0092-8674(02)00905-4.
- Gripenland J, Netterling S, Loh E, Tiensuu T, Toledo-Arana A, Johansson J. RNAs: regulators of bacterial virulence. Nat Rev Microbiol. 2010;8(12):857–866. doi:10.1038/nrmicro2457.
- Chaudhuri S, Gantner BN, Ye RD, Cianciotto NP, Freitag NE. The Listeria monocytogenes ChiA chitinase enhances virulence through suppression of host innate immunity. mBio. 2013;4(2): p. e00617–12. doi:10.1128/mBio.00617-12.
- Frantz R, Teubner L, Schultze T, La Pietra L, Müller C, Gwozdzinski K, Pillich H, Hain T, Weber-Gerlach M, Panagiotidis GD, et al. The secRnome of Listeria monocytogenes harbors small noncoding RNAs that are potent inducers of beta interferon. mBio. 2019;10(5).
- Sievers S, Sternkopf Lillebæk EM, Jacobsen K, Lund A, Mollerup MS, Nielsen PK, Kallipolitis BH. A multicopy sRNA of Listeria monocytogenes regulates expression of the virulence adhesin LapB. Nucleic Acids Res. 2014;42(14):9383–9398. doi:10.1093/nar/gku630.
- Dos Santos PT, Menendez-Gil P, Sabharwal D, Christensen J-H, Brunhede MZ, Lillebæk EMS, Kallipolitis BH. The small regulatory RNAs LhrC1–5 contribute to the response of listeria monocytogenes to heme toxicity. Front Microbiol. 2018;9:599. doi:10.3389/fmicb.2018.00599.
- Sievers S, Lund A, Menendez-Gil P, Nielsen A, Storm Mollerup M, Lambert Nielsen S, Buch Larsson P, Borch-Jensen J, Johansson J, Kallipolitis BH. The multicopy sRNA LhrC controls expression of the oligopeptide-binding protein OppA in Listeria monocytogenes. RNA Biol. 2015;12(9):985–997. doi:10.1080/15476286.2015.1071011.
- Brenner M, Lobel L, Borovok I, Sigal N, Herskovits AA. Controlled branched-chain amino acids auxotrophy in Listeria monocytogenes allows isoleucine to serve as a host signal and virulence effector. PLOS Genet. 2018;14(3):e1007283. doi:10.1371/journal.pgen.1007283.
- Peng YL, Meng Q-L, Qiao J, Xie K, Chen C, Liu T-L, Hu Z-X, Ma Y, Cai X-P, Chen C-F, et al. The roles of noncoding RNA Rli60 in regulating the virulence of Listeria monocytogenes. J Microbiol Immunol Infect. 2016;49(4):502–508. doi:10.1016/j.jmii.2014.08.017.
- Tian Y, Wu L, Zhu M, Yang Z, Pilar G, Bao H, Zhou Y, Wang R, Zhang H. Non-coding RNA regulates phage sensitivity in Listeria monocytogenes. PLOS One. 2021;16(12):e0260768. doi:10.1371/journal.pone.0260768.
- Marinho CM, Dos Santos PT, Kallipolitis BH, Johansson J, Ignatov D, Guerreiro DN, Piveteau P, O’Byrne CP. The σ B-dependent regulatory sRNA Rli47 represses isoleucine biosynthesis in Listeria monocytogenes through a direct interaction with the ilvA transcript. RNA Biol. 2019;16(10):1424–1437. doi:10.1080/15476286.2019.1632776.
- Mollerup MS, Ross JA, Helfer A-C, Meistrup K, Romby P, Kallipolitis BH. Two novel members of the LhrC family of small RNAs in Listeria monocytogenes with overlapping regulatory functions but distinctive expression profiles. RNA Biol. 2016;13(9):895–915. doi:10.1080/15476286.2016.1208332.
- Quereda JJ, Ortega ÁD, Pucciarelli MG, García-Del Portillo F. The listeria small RNA Rli27 regulates a cell wall protein inside eukaryotic cells by targeting a long 5′-UTR variant. PLOS Genet. 2014;10(10):e1004765. doi:10.1371/journal.pgen.1004765.
- Burke TP, Loukitcheva A, Zemansky J, Wheeler R, Boneca IG, Portnoy DA. Listeria monocytogenes is resistant to lysozyme through the regulation, not the acquisition, of cell wall-modifying enzymes. J Bacteriol. 2014;196(21):3756–3767. doi:10.1128/JB.02053-14.
- Burke TP, Portnoy DA. SpoVG Is a Conserved RNA-Binding protein that regulates listeria monocytogenes lysozyme resistance, virulence, and swarming motility. mBio. 2016;7(2):e00240.
- Grubaugh DR, Regeimbal JM, Ghosh P, Zhou Y, Lauer P, Dubensky Jr TW, Higgins DE. The VirAB ABC transporter is required for virr regulation of listeria monocytogenes virulence and resistance to nisin. Infect And Immun. 2018;86(3):e00901–17. doi:10.1128/IAI.00901-17.
- Krawczyk-Balska A, Ładziak M, Burmistrz M, Ścibek K, Kallipolitis BH. RNA-Mediated control in listeria monocytogenes: insights into regulatory mechanisms and roles in metabolism and virulence. Front Microbiol. 2021;12:622829. doi:10.3389/fmicb.2021.622829.
- Mraheil MA, Billion A, Mohamed W, Mukherjee K, Kuenne C, Pischimarov J, Krawitz C, Retey J, Hartsch T, Chakraborty T, et al. The intracellular sRNA transcriptome of Listeria monocytogenes during growth in macrophages. Nucleic Acids Res. 2011;39(10):4235–4248. doi:10.1093/nar/gkr033.
- Kallipolitis BH, Ingmer H, Gahan CG, Hill C, Søgaard-Andersen L. CesRK, a two-component signal transduction system in listeria monocytogenes , responds to the presence of cell wall-acting antibiotics and affects β-lactam resistance. Antimicrob Agents Chemother. 2003;47(11):3421–3429. doi:10.1128/AAC.47.11.3421-3429.2003.
- Gottschalk S, Bygebjerg-Hove I, Bonde M, Nielsen PK, Nguyen TH, Gravesen A, Kallipolitis BH. The two-component system CesRK controls the transcriptional induction of cell envelope-related genes in Listeria monocytogenes in response to cell wall-acting antibiotics. J Bacteriol. 2008;190(13):4772–4776. doi:10.1128/JB.00015-08.
- Stack HM, Sleator RD, Bowers M, Hill C, Gahan CGM. Role for HtrA in stress induction and virulence potential in Listeria monocytogenes. Appl Environ Microbiol. 2005;71(8):4241–4247. doi:10.1128/AEM.71.8.4241-4247.2005.
- Aslan H, Petersen ME, De Berardinis A, Zacho Brunhede M, Khan N, Vergara A, Kallipolitis B, Meyer RL. Activation of the two-component system lisrk promotes cell adhesion and high ampicillin tolerance in listeria monocytogenes. Front Microbiol. 2021;12:618174. doi:10.3389/fmicb.2021.618174.
- Sleator RD, Hill C. A novel role for the LisRK two-component regulatory system in listerial osmotolerance. Clin Microbiol Infect. 2005;11(8):599–601. doi:10.1111/j.1469-0691.2005.01176.x.
- Mandin P, Fsihi H, Dussurget O, Vergassola M, Milohanic E, Toledo‐Arana A, Lasa I, Johansson J, Cossart P. VirR, a response regulator critical for Listeria monocytogenes virulence. Mol Microbiol. 2005;57(5):1367–1380. doi:10.1111/j.1365-2958.2005.04776.x.
- Cahoon LA, Alejandro‐Navarreto X, Gururaja AN, Light SH, Alonzo F, Anderson WF, Freitag NE. Listeria monocytogenes two component system PieRS regulates secretion chaperones PrsA1 and PrsA2 and enhances bacterial translocation across the intestine. Mol Microbiol. 2022;118(3):278–293. doi:10.1111/mmi.14967.
- Forster BM, Zemansky J, Portnoy DA, Marquis H. Posttranslocation chaperone PrsA2 regulates the maturation and secretion of Listeria monocytogenes proprotein virulence factors. J Bacteriol. 2011;193(21):5961–5970. doi:10.1128/JB.05307-11.
- T L-WMDEC, Domann E, Chakraborty T. The expression of virulence genes in Listeria monocytogenes is thermoregulated. J Bacteriol. 1992;174(3):947–952. doi:10.1128/jb.174.3.947-952.1992.
- Kamp HD, Higgins DE, Blanke SR. A protein thermometer controls temperature-dependent transcription of flagellar motility genes in Listeria monocytogenes. PLOS Pathog. 2011;7(8):e1002153. doi:10.1371/journal.ppat.1002153.
- Guerreiro DN, Pucciarelli MG, Tiensuu T, Gudynaite D, Boyd A, Johansson J, García-Del Portillo F, O’Byrne CP. Acid stress signals are integrated into the σB-dependent general stress response pathway via the stressosome in the food-borne pathogen Listeria monocytogenes. PLOS Pathog. 2022;18(3):e1010213. doi:10.1371/journal.ppat.1010213.
- Kazmierczak MJ, Mithoe SC, Boor KJ, Wiedmann M. Listeria monocytogenes σ B regulates stress response and virulence functions. J Bacteriol. 2003;185(19):5722–5734. doi:10.1128/JB.185.19.5722-5734.2003.
- Shin JH, Brody MS, Price CW. Physical and antibiotic stresses require activation of the RsbU phosphatase to induce the general stress response in Listeria monocytogenes. Microbiol (Read). 2010;156(Pt 9):2660–2669. doi:10.1099/mic.0.041202-0.
- Dessaux C, Pucciarelli MG, Guerreiro DN, O’Byrne CP, García-Del Portillo F. Activation of the Listeria monocytogenes stressosome in the intracellular eukaryotic environment. Appl Environ Microbiol. 2021;87(12):e0039721. doi:10.1128/AEM.00397-21.
- Feehily C, Karatzas KA. Role of glutamate metabolism in bacterial responses towards acid and other stresses. J Appl Microbiol. 2013;114(1):11–24. doi:10.1111/j.1365-2672.2012.05434.x.
- Boura M, Brensone D, Karatzas KAG. A novel role for the glutamate decarboxylase system in Listeria monocytogenes; protection against oxidative stress. Food Microbiol. 2020;85:103284. doi:10.1016/j.fm.2019.103284.
- Glomski IJ, Gedde MM, Tsang AW, Swanson JA, Portnoy DA. The Listeria monocytogenes hemolysin has an acidic pH optimum to compartmentalize activity and prevent damage to infected host cells. J Cell Biol. 2002;156(6):1029–1038. doi:10.1083/jcb.200201081.
- Beauregard KEL, D K, Collier RJ, Swanson JA. pH-dependent perforation of macrophage phagosomes by listeriolysin O from Listeria monocytogenes. The J Exp Med. 1997;186(7):1159–1163. doi:10.1084/jem.186.7.1159.
- Podobnik M, Marchioretto M, Zanetti M, Bavdek A, Kisovec M, Cajnko MM, Lunelli L, Serra MD, Anderluh G. Plasticity of listeriolysin O pores and its regulation by pH and unique histidine [corrected]. Sci Rep. 2015;5(1):9623. doi:10.1038/srep09623.
- Hamon MA, Ribet D, Stavru F, Cossart P. Listeriolysin O: The Swiss army knife of Listeria. Trends Microbiol. 2012;20(8):360–368. doi:10.1016/j.tim.2012.04.006.
- Brenner MF, Haber S, Livnat-Levanon A, Borovok N, Sigal I, Lewinson N, Herskovits O, A A. Listeria monocytogenes TcyKLMN Cystine/Cysteine LOransporter facilitates glutathione synthesis and virulence gene expression. mBio. 2022;13(3):e0044822. doi:10.1128/mbio.00448-22.
- Gopal S, Borovok I, Ofer A, Yanku M, Cohen G, Goebel W, Kreft J, Aharonowitz Y. A multidomain fusion protein in Listeria monocytogenes catalyzes the two primary activities for glutathione biosynthesis. J Bacteriol. 2005;187(11):3839–3847. doi:10.1128/JB.187.11.3839-3847.2005.
- Krypotou E, Scortti M, Grundström C, Oelker M, Luisi BF, Sauer-Eriksson AE, Vázquez-Boland J. Control of bacterial virulence through the peptide signature of the habitat. Cell Rep. 2019;26(7):1815–1827 e5. doi:10.1016/j.celrep.2019.01.073.
- Dussurget O, Cabanes D, Dehoux P, Lecuit M, Buchrieser C, Glaser P, Cossart P. Listeria monocytogenes bile salt hydrolase is a PrfA-regulated virulence factor involved in the intestinal and hepatic phases of listeriosis. Mol Microbiol. 2002;45(4):1095–1106. doi:10.1046/j.1365-2958.2002.03080.x.
- Quillin SJ, Schwartz KT, Leber JH. The novel Listeria monocytogenes bile sensor BrtA controls expression of the cholic acid efflux pump MdrT. Mol Microbiol. 2011;81(1):129–142. doi:10.1111/j.1365-2958.2011.07683.x.
- Schwartz KT, Carleton JD, Quillin SJ, Rollins SD, Portnoy DA, Leber JH. Hyperinduction of host beta interferon by a Listeria monocytogenes strain naturally overexpressing the multidrug efflux pump MdrT. Infect Immun. 2012;80(4):1537–1545. doi:10.1128/IAI.06286-11.
- Pombinho R, Vieira A, Camejo A, Archambaud C, Cossart P, Sousa S, Cabanes D. Virulence gene repression promotes Listeria monocytogenes systemic infection. Gut Microbes. 2020;11(4):868–881. doi:10.1080/19490976.2020.1712983.
- Lobel L, Sigal N, Borovok I, Ruppin E, Herskovits AA. Integrative genomic analysis identifies isoleucine and CodY as regulators of Listeria monocytogenes virulence. PLOS Genet. 2012;8(9):e1002887. doi:10.1371/journal.pgen.1002887.
- Levdikov VM, Blagova E, Joseph P, Sonenshein AL, Wilkinson AJ. The structure of CodY, a GTP- and isoleucine-responsive regulator of stationary phase and virulence in gram-positive bacteria. J Biol Chem. 2006;281(16):11366–11373. doi:10.1074/jbc.M513015200.
- Shivers RP, Sonenshein AL. Activation of the Bacillus subtilis global regulator CodY by direct interaction with branched-chain amino acids. Mol Microbiol. 2004;53(2):599–611. doi:10.1111/j.1365-2958.2004.04135.x.
- Lobel L, Sigal N, Borovok I, Belitsky BR, Sonenshein AL, Herskovits AA. The metabolic regulator CodY links Listeria monocytogenes metabolism to virulence by directly activating the virulence regulatory gene prfA. Mol Microbiol. 2015;95(4):624–644. doi:10.1111/mmi.12890.
- Sternkopf Lillebaek EM, Lambert Nielsen S, Scheel Thomasen R, Færgeman NJ, Kallipolitis BH. Antimicrobial medium- and long-chain free fatty acids prevent PrfA-dependent activation of virulence genes in Listeria monocytogenes. Res Microbiol. 2017;168(6):547–557. doi:10.1016/j.resmic.2017.03.002.
- Dos Santos PT, Thomasen RSS, Green MS, Færgeman NJ, Kallipolitis BH. Free fatty acids interfere with the DNA binding activity of the virulence regulator PrfA of Listeria monocytogenes. J Bacteriol. 2020;202(15). doi:10.1128/JB.00156-20.
- Kutzner E, Kern T, Felsl A, Eisenreich W, Fuchs TM. Isotopologue profiling of the listerial N-metabolism. Mol Microbiol. 2016;100(2):315–327. doi:10.1111/mmi.13318.
- Haber A, Friedman S, Lobel L, Burg-Golani T, Sigal N, Rose J, Livnat-Levanon N, Lewinson O, Herskovits AA. L-glutamine induces expression of listeria monocytogenes virulence genes. PLOS Pathog. 2017;13(1):e1006161. doi:10.1371/journal.ppat.1006161.
- Ripio MT, Brehm K, Lara M, Suarez M, Vázquez-Boland JA. Glucose-1-phosphate utilization by Listeria monocytogenes is PrfA dependent and coordinately expressed with virulence factors. J Bacteriol. 1997;179(22):7174–7180. doi:10.1128/jb.179.22.7174-7180.1997.
- Chico-Calero IS, González-Zorn M, Scortti B, Slaghuis M, Goebel W, Vázquez-Boland JA. European Listeria genome consortium, Hpt, a bacterial homolog of the microsomal glucose- 6-phosphate translocase, mediates rapid intracellular proliferation in Listeria. Proc Natl Acad Sci USA. 2002;99(1):431–436. doi:10.1073/pnas.012363899.
- Haschka D, Nairz M, Demetz E, Wienerroither S, Decker T, Weiss G. Contrasting regulation of macrophage iron homeostasis in response to infection with Listeria monocytogenes depending on localization of bacteria. Metallomics. 2015;7(6):1036–1045. doi:10.1039/C4MT00328D.
- Lam GY, Fattouh R, Muise A, Grinstein S, Higgins D, Brumell J. Listeriolysin O suppresses phospholipase C-mediated activation of the microbicidal NADPH oxidase to promote Listeria monocytogenes infection. Cell Host & Microbe. 2011;10(6):627–634. doi:10.1016/j.chom.2011.11.005.
- Dos Santos PT, Larsen PT, Menendez-Gil P, Lillebæk EMS, Kallipolitis BH. Listeria monocytogenes relies on the heme-regulated transporter hrtAB to resist heme toxicity and uses heme as a signal to induce transcription of lmo1634. Encoding Listeria Adhes Protein Front Microbiol. 2018;9:3090. doi:10.3389/fmicb.2018.03090.
- Böckmann RD, Middendorf C, Goebel B, Sokolovic W, Sokolovic, ZZ. Specific binding of the Listeria monocytogenes transcriptional regulator PrfA to target sequences requires additional factor(s) and is influenced by iron. Mol Microbiol. 1996;22(4):643–653. doi:10.1046/j.1365-2958.1996.d01-1722.x.
- Conte MP, Longhi C, Polidoro M, Petrone G, Buonfiglio V, Di Santo S, Papi E, Seganti L, Visca P, Valenti P. Iron availability affects entry of Listeria monocytogenes into the enterocytelike cell line Caco-2. Infect And Immun. 1996;64(9):3925–3929.
- Pi H, Patel SJ, Argüello JM, Helmann JD. The Listeria monocytogenes fur-regulated virulence protein FrvA is an Fe(II) efflux P1B4-type ATPase. Mol Microbiol. 2016;100(6):1066–1079. doi:10.1111/mmi.13368.
- McLaughlin HP, Bahey-El-Din M, Casey PG, Hill C, Gahan CGM. A mutant in the Listeria monocytogenes fur-regulated virulence locus (frvA) induces cellular immunity and confers protection against listeriosis in mice. J Med Microbiol. 2013;62(2):185–190. doi:10.1099/jmm.0.049114-0.
- Rea R, Hill C, Gahan CG. Listeria monocytogenes PerR mutants display a small-colony phenotype, increased sensitivity to hydrogen peroxide, and significantly reduced murine virulence. Appl Environ Microbiol. 2005;71(12):8314–8322. doi:10.1128/AEM.71.12.8314-8322.2005.
- Makino M, Kawai M, Kawamura I, Fujita M, Gejo F, Mitsuyama M. Involvement of reactive oxygen intermediate in the enhanced expression of virulence-associated genes of Listeria monocytogenes inside activated macrophages. Microbiol Immunol. 2005;49(8):805–811. doi:10.1111/j.1348-0421.2005.tb03661.x.
- Mains DR, Eallonardo SJ, Freitag NE, Torres VJ. Identification of listeria monocytogenes genes contributing to oxidative stress resistance under conditions relevant to host infection. Infect Immun. 2021;89(4). doi:10.1128/IAI.00700-20.
- Waters CM, Bassler BL. Quorum sensing: cell-to-cell communication in bacteria. Annu Rev Cell Dev Biol. 2005;21(1):319–346. doi:10.1146/annurev.cellbio.21.012704.131001.
- Paul D, Gopal J, Kumar M, Manikandan M. Nature to the natural rescue: Silencing microbial chats. Chem Biol Interact. 2018;280:86–98. doi:10.1016/j.cbi.2017.12.018.
- Hawver LA, Jung SA, Ng WL, Shen A. Specificity and complexity in bacterial quorum-sensing systems. FEMS Microbiol Rev. 2016;40(5):738–752. doi:10.1093/femsre/fuw014.
- Novick RP. Autoinduction and signal transduction in the regulation of staphylococcal virulence. Mol Microbiol. 2003;48(6):1429–1449. doi:10.1046/j.1365-2958.2003.03526.x.
- Michiel Kleerebezem LENQ, Kuipers OP, de Vos WM, De Vos WM. Quorum sensing by peptide pheromones and two component signal-transduction systems in Gram-positive bacteria. Mol Microbiol. 1997;25(5):895–904. doi:10.1046/j.1365-2958.1997.4251782.x.
- Lyon GJ, Novick RP. Peptide signaling in Staphylococcus aureus and other Gram-positive bacteria. Peptides. 2004;25(9):1389–1403. doi:10.1016/j.peptides.2003.11.026.
- Stock AM, Goudreau RV, Goudreau, Pn PN. Two-component signal transduction. Annu Rev In Biochem. 2000;69(1):183–215. doi:10.1146/annurev.biochem.69.1.183.
- Rutherford ST, Bassler BL. Bacterial quorum sensing: its role in virulence and possibilities for its control. Cold Spring Harb Perspect Med. 2012;2(11):p.a01242727. doi:10.1101/cshperspect.a012427.
- Slamti L, L D. A cell-cell singaling peptide activates PlcR virulence regulon in bacteria. Embo J. 2002;21(17):4550–4559. doi:10.1093/emboj/cdf450.
- Autret N, Raynaud C, Dubail I, Berche P, Charbit A. Identification of the Agr locus of Listeria monocytogenes: role in bacterial virulence. Infect Immun. 2003;71(8):4463–4471. doi:10.1128/IAI.71.8.4463-4471.2003.
- Podbielski A, Kreikemeyer B. Cell density–dependent regulation: basic principles and effects on the virulence of Gram-positive cocci. Int J Infect Dis. 2004;8(2):81–95. doi:10.1016/j.ijid.2003.04.003.
- Ohtani K, Yuan Y, Hassan S, Wang R, Wang Y, Shimizu T. Virulence gene regulation by the agr system in Clostridium perfringens. J Bacteriol. 2009;191(12):3919–3927. doi:10.1128/JB.01455-08.
- Thoendel M, Kavanaugh JS, Flack CE, Horswill AR. Peptide signaling in the staphylococci. Chem Rev. 2011;111(1):117–151. doi:10.1021/cr100370n.
- Pinheiro J, Lisboa J, Pombinho R, Carvalho F, Carreaux A, Brito C, Pöntinen A, Korkeala H, dos Santos NM, Morais-Cabral JH, et al. MouR controls the expression of the Listeria monocytogenes Agr system and mediates virulence. Nucleic Acids Res. 2018;46(18):9338–9352. doi:10.1093/nar/gky624.
- Paspaliari DK, Mollerup MS, Kallipolitis BH, Ingmer H, Larsen MH. Chitinase expression in Listeria monocytogenes is positively regulated by the Agr system. PLOS One. 2014;9(4):e95385. doi:10.1371/journal.pone.0095385.
- Zeng Z, Boeren S, Bhandula V, Light SH, Smid EJ, Notebaart RA, Abee T. Bacterial Microcompartments coupled with extracellular electron transfer drive the anaerobic utilization of ethanolamine in Listeria monocytogenes. mSystems. 2021;6(2).
- Lebreton A, Cossart P. RNA- and protein-mediated control of Listeria monocytogenes virulence gene expression. RNA Biol. 2017;14(5):460–470. doi:10.1080/15476286.2016.1189069.
- Rieu A, Weidmann S, Garmyn D, Piveteau P, Guzzo J. Agr system of Listeria monocytogenes EGD-e: role in adherence and differential expression pattern. Appl Environ Microbiol. 2007;73(19):6125–6133. doi:10.1128/AEM.00608-07.
- Vivant AL, Garmyn D, Gal L, Piveteau P. The agr communication system provides a benefit to the populations of listeria monocytogenes in soil. Front Cell Infect Microbiol. 2014;4:160. doi:10.3389/fcimb.2014.00160.
- Saenz HL, Augsburger V, Vuong C, Jack RW, Götz F, Otto M. Inducible expression and cellular location of AgrB, a protein involved in the maturation of the staphylococcal quorum-sensing pheromone. Arch Microbiol. 2000;174(6):452–455. doi:10.1007/s002030000223.
- Gless BH, Bejder BS, Monda F, Bojer MS, Ingmer H, Olsen CA. Rearrangement of Thiodepsipeptides by S → N acyl shift delivers homodetic autoinducing peptides. J Am Chem Soc. 2021;143(28):10514–10518. doi:10.1021/jacs.1c02614.
- Hoskisson PA, Rigali S. Chapter 1: Variation in form and function the helix-turn-helix regulators of the GntR superfamily. Adv Appl Microbiol. 2009;69:1–22.
- Suvorova IA, Korostelev YD, Gelfand MS, Rogozin IB. GntR family of bacterial transcription factors and their DNA binding motifs: structure, positioning and co-evolution. PLAS One. 2015;10(7):e0132618. doi:10.1371/journal.pone.0132618.
- Jain D. Allosteric control of transcription in GntR family of transcription regulators: a structural overview. IUBMB Life. 2015;67(7):556–563. doi:10.1002/iub.1401.
- West KHJ, Ma SV, Pensinger DA, Tucholski T, Tiambeng TN, Eisenbraun EL, Yehuda A, Hayouka Z, Ge Y, Sauer J-D, et al. Characterization of an Autoinducing peptide signal reveals highly efficacious synthetic inhibitors and activators of quorum sensing and biofilm formation in Listeria monocytogenes. Biochemistry. 2023;62(19):2878–2892. doi:10.1021/acs.biochem.3c00373.
- Marques PH, Jaiswal AK, de Almeida FA, Pinto UM, Ferreira-Machado AB, Tiwari S, Soares SC, Paiva AD. Lactic acid bacteria secreted proteins as potential Listeria monocytogenes quorum sensing inhibitors. Mol Divers; 2023.
- Guerreiro DN, Wu J, McDermott E, Garmyn D, Dockery P, Boyd A, Piveteau P, O’Byrne CP. In vitro evolution of listeria monocytogenes reveals selective pressure for loss of sigb and AgrA function at different incubation temperatures. Appl Environ Microbiol. 2022;88(11):e0033022. doi:10.1128/aem.00330-22.
- Caldelari I, Chao Y, Romby P, Vogel J. RNA-mediated regulation in pathogenic bacteria. Cold Spring Harb Perspect Med. 2013;3(9):a010298. doi:10.1101/cshperspect.a010298.
- Storz G, Vogel J, Wassarman KM. Regulation by small RNAs in bacteria: expanding frontiers. Mol Cell. 2011;43(6):880–891. doi:10.1016/j.molcel.2011.08.022.
- Papenfort K, Vogel J. Regulatory RNA in bacterial pathogens. Cell Host Microbe. 2010;8(1):116–127. doi:10.1016/j.chom.2010.06.008.
- Waters LS, Storz G. Regulatory RNAs in bacteria. Cell. 2009;136(4):615–628. doi:10.1016/j.cell.2009.01.043.
- Geissmann T, Possedko M, Huntzinger E, Fechter P, Ehresmann C, Romby P. Regulatory RNAs as mediators of virulence gene expression in bacteria. In: Erdmann V, Barciszewski J, Brosius J, editors. RNA towards medicine. Handbook of experimental pharmacology. Vol. 173. Berlin, Heidelberg: Springer; 2006. doi:10.1007/3-540-27262-3_2.
- Becavin C, Koutero M, Tchitchek N, Cerutti F, Lechat P, Maillet N, Hoede C, Chiapello H, Gaspin C, Cossart P, et al. Listeriomics: an interactive web platform for systems biology of Listeria. mSystems. 2017;2(2):2(2. doi:10.1128/mSystems.00186-16.
- Schultze T, Izar B, Qing X, Mannala GK, Hain T. Current status of antisense RNA-mediated gene regulation in Listeria monocytogenes. Vol. 4. Front Cell Infect Microbiol; 2014. p. 135.
- Saberi F, Kamali M, Najafi A, Yazdanparast A, Moghaddam MM. Natural antisense RNAs as mRNA regulatory elements in bacteria: a review on function and applications. Cell Mol Biol Lett. 2016;21(1):6. doi:10.1186/s11658-016-0007-z.
- Kortmann J, Narberhaus F. Bacterial RNA thermometers: molecular zippers and switches. Nat Rev Microbiol. 2012;10(4):255–265. doi:10.1038/nrmicro2730.
- Heroven AK, Nuss AM, Dersch P. RNA-based mechanisms of virulence control in enterobacteriaceae. RNA Biol. 2017;14(5):471–487. doi:10.1080/15476286.2016.1201617.
- de las Heras A, Cain RJ, Bielecka MK, Vázquez-Boland JA. Regulation of Listeria virulence: PrfA master and commander. Curr Opin Microbiol. 2011;14(2):118–127. doi:10.1016/j.mib.2011.01.005.
- Hamon M, Bierne H, Cossart P. Listeria monocytogenes: a multifaceted model. Nat Rev Microbiol. 2006;4(6):423–434. doi:10.1038/nrmicro1413.
- Freitag NE, Port GC, Miner MD. Listeria monocytogenes - from saprophyte to intracellular pathogen. Nat Rev Microbiol. 2009;7(9):623–628. doi:10.1038/nrmicro2171.
- Johansson J, Renzoni MP, Chiaruttini A, Springer C, Cossart M, Cossart P. An RNA thermosensor controls expression of virulence genes in listeria monocytogenes. Cell. 2002;110(5):551–561. doi:10.1016/S0092-8674(02)00905-4.
- Scortti M, Monzó HJ, Lacharme-Lora L, Lewis DA, Vázquez-Boland JA. The PrfA virulence regulon. Microbes Infect. 2007;9(10):1196–1207. doi:10.1016/j.micinf.2007.05.007.
- Traykovska M, Penchovsky R. Targeting SAM-I riboswitch using antisense oligonucleotide technology for inhibiting the growth of staphylococcus aureus and listeria monocytogenes. Antibiot (Basel). 2022;11(11). doi:10.3390/antibiotics11111662.
- Papenfort K, Vogel J. Multiple target regulation by small noncoding RNAs rewires gene expression at the post-transcriptional level. Res Microbiol. 2009;160(4):278–287. doi:10.1016/j.resmic.2009.03.004.
- Cho KH, Kim JH. Cis-encoded non-coding antisense RNAs in streptococci and other low GC Gram (+) bacterial pathogens. Front Genet. 2015;6:110. doi:10.3389/fgene.2015.00110.
- Miller EW, Cao TN, Pflughoeft KJ, Sumby P. RNA-mediated regulation in gram-positive pathogens: an overview punctuated with examples from the group a streptococcus. Mol Microbiol. 2014;94(1):9–20. doi:10.1111/mmi.12742.
- Romby P, Charpentier E. An overview of RNAs with regulatory functions in gram-positive bacteria. Cell Mol Life Sci. 2010;67(2):217–237. doi:10.1007/s00018-009-0162-8.
- Christiansen JK, Nielsen JS, Ebersbach T, Valentin-Hansen P, Søgaard-Andersen L, Kallipolitis BH. Identification of small Hfq-binding RNAs in Listeria monocytogenes. Rna. 2006;12(7):1383–1396. doi:10.1261/rna.49706.
- Nielsen JS, Larsen MH, Lillebæk EMS, Bergholz TM, Christiansen MHG, Boor KJ, Wiedmann M, Kallipolitis BH. A small RNA controls expression of the chitinase ChiA in Listeria monocytogenes. PLOS One. 2011;6(4):e19019. doi:10.1371/journal.pone.0019019.
- Chaudhuri S, Bruno JC, Alonzo F, Xayarath B, Cianciotto NP, Freitag NE. Contribution of chitinases to Listeria monocytogenes pathogenesis. Appl Environ Microbiol. 2010;76(21):7302–7305. doi:10.1128/AEM.01338-10.
- Garcia-Del Portillo F, Calvo E, D’Orazio V, Pucciarelli MG. Association of ActA to peptidoglycan revealed by cell wall proteomics of intracellular Listeria monocytogenes. J Biol Chem. 2011;286(40):34675–34689. doi:10.1074/jbc.M111.230441.
- Quereda JJ, Garcia-Del Portillo F, Pucciarelli MG. Listeria monocytogenes remodels the cell surface in the blood-stage. Environ Microbiol Rep. 2016;8(5):641–648. doi:10.1111/1758-2229.12416.
- Peng YL, Meng Q-L, Qiao J, Xie K, Chen C, Liu T-L, Hu Z-X, Ma Y, Cai X-P, Chen C-F, et al. The Regulatory roles of ncRNA Rli60 in adaptability of Listeria monocytogenes to environmental stress and biofilm formation. Curr Microbiol. 2016;73(1):77–83. doi:10.1007/s00284-016-1028-6.
- Camilli A, Bassler BL. Bacterial small-molecule signaling pathways. Science. 2006;311(5764):1113–1116. doi:10.1126/science.1121357.
- Pesavento C, Hengge R. Bacterial nucleotide-based second messengers. Curr Opin Microbiol. 2009;12(2):170–176. doi:10.1016/j.mib.2009.01.007.
- Elbakush AM, Miller KW, Gomelsky M. CodY-Mediated c-di-GMP-Dependent inhibition of mammalian cell invasion in Listeria monocytogenes. J Bacteriol. 2018;200(5):200(5. doi:10.1128/JB.00457-17.
- Witte CE, Whiteley AT, Burke TP, Sauer J-D, Portnoy DA, Woodward JJ. Cyclic di-AMP is critical for Listeria monocytogenes growth, cell wall homeostasis, and establishment of infection. mBio. 2013;4(3): p. e00282–13. doi:10.1128/mBio.00282-13.
- Fahmi T, Port GC, Cho KH. c-di-AMP: An essential molecule in the signaling pathways that regulate the viability and virulence of gram-positive bacteria. Genes (Basel). 2017;8(8):8(8. doi:10.3390/genes8080197.
- Whiteley AT, Garelis NE, Peterson BN, Choi PH, Tong L, Woodward JJ, Portnoy DA. c-di-AMP modulates Listeria monocytogenes central metabolism to regulate growth, antibiotic resistance and osmoregulation. Mol Microbiol. 2017;104(2):212–233. doi:10.1111/mmi.13622.
- Huynh TN, Choi PH, Sureka K, Ledvina HE, Campillo J, Tong L, Woodward JJ. Cyclic di-AMP targets the cystathionine beta-synthase domain of the osmolyte transporter OpuC. Mol Microbiol. 2016;102(2):233–243. doi:10.1111/mmi.13456.
- Woodward JJ, Iavarone AT, Portnoy DA. c-di-AMP secreted by intracellular Listeria monocytogenes activates a host type I interferon response. Science. 2010;328(5986):1703–1705. doi:10.1126/science.1189801.
- Jenal U, Reinders A, Lori C. Cyclic di-GMP: second messenger extraordinaire. Nat Rev Microbiol. 2017;15(5):271–284. doi:10.1038/nrmicro.2016.190.
- Galperin MY, Natale DA, Aravind L, Koonin EV. A specialized version of the HD hydrolase domain implicated in signal transduction. J Mol Microbiol Biotechnol. 1999;1(2):303–305.
- Schmidt AJ, Ryjenkov DA, Gomelsky M. The ubiquitous protein domain EAL is a cyclic diguanylate-specific phosphodiesterase: enzymatically active and inactive EAL domains. J Bacteriol. 2005;187(14):4774–4781. doi:10.1128/JB.187.14.4774-4781.2005.
- Valentini M, Filloux A. Multiple Roles of c-di-GMP signaling in bacterial pathogenesis. Annu Rev Microbiol. 2019;73(1):387–406. doi:10.1146/annurev-micro-020518-115555.
- Romling U, Gomelsky M, Galperin MY. C-di-GMP: the dawning of a novel bacterial signalling system. Mol Microbiol. 2005;57(3):629–639. doi:10.1111/j.1365-2958.2005.04697.x.
- Waters CM, Lu W, Rabinowitz JD, Bassler BL. Quorum sensing controls biofilm formation in Vibrio cholerae through modulation of cyclic di-GMP levels and repression of vpsT. J Bacteriol. 2008;190(7):2527–2536. doi:10.1128/JB.01756-07.
- Srivastava D, Waters CM. A tangled web: regulatory connections between quorum sensing and cyclic Di-GMP. J Bacteriol. 2012;194(17):4485–4493. doi:10.1128/JB.00379-12.
- Chen LH, Köseoğlu VK, Güvener ZT, Myers-Morales T, Reed JM, D’Orazio SEF, Miller KW, Gomelsky M. Cyclic di-GMP-dependent signaling pathways in the pathogenic firmicute Listeria monocytogenes. PloS Pathog. 2014;10(8):e1004301. doi:10.1371/journal.ppat.1004301.
- Hengge R. Trigger phosphodiesterases as a novel class of c-di-GMP effector proteins. Philos Trans R Soc Lond B Biol Sci. 2016;371(1707):20150498. doi:10.1098/rstb.2015.0498.
- Huynh TN, Woodward JJ. Too much of a good thing: regulated depletion of c-di-AMP in the bacterial cytoplasm. Curr Opin Microbiol. 2016;30:22–29. doi:10.1016/j.mib.2015.12.007.
- Commichau FM, Heidemann JL, Ficner R, Stülke J. Making and breaking of an essential poison: the Cyclases and Phosphodiesterases that produce and degrade the essential second messenger Cyclic di-AMP in Bacteria. J Bacteriol. 2019;201(1):201(1. doi:10.1128/JB.00462-18.
- Gall AR, Hsueh BY, Siletti C, Waters CM, Huynh TN. NrnA is a linear dinucleotide phosphodiesterase with limited function in cyclic dinucleotide metabolism in listeria monocytogenes. J Bacteriol. 2022;204(1):e0020621. doi:10.1128/JB.00206-21.
- Shaw C, Hess M, Weimer BC. Two-component systems regulate bacterial virulence in response to the host gastrointestinal environment and metabolic cues. Virulence. 2022;13(1):1666–1680. doi:10.1080/21505594.2022.2127196.
- A HJ. Two-component and phosphorelay signal transduction. Current opinion in microbiology. Curr Opin In Microbiol. 2000;3(2):165–170. doi:10.1016/S1369-5274(00)00070-9.
- Cotter PD, Emerson N, Gahan CG, Hill C. Identification and disruption of lisRK, a genetic locus encoding a two-component signal transduction system involved in stress tolerance and virulence in listeria monocytogenes. J Bacteriol. 1999;181(21):6840–6843. doi:10.1128/JB.181.21.6840-6843.1999.
- Kallipolitis BHI, H. Listeria monocytogenes response regulators important for stress tolerance and pathogenesis. FEMS microbiology letters. FEMS Microbiol Lett. 2001;204(1):111–115. doi:10.1016/S0378-1097(01)00386-X.
- Alonzo FP, C G, Cao M, Freitag NE. The posttranslocation chaperone PrsA2 contributes to multiple facets of Listeria monocytogenes pathogenesis. Infect Immun. 2009;77(7):2612–2623. doi:10.1128/IAI.00280-09.
- Cahoon LA, Freitag NE, Camilli A. Identification of conserved and species-specific functions of the listeria monocytogenes PrsA2 Secretion Chaperone. Infect Immun. 2015;83(10):4028–4041. doi:10.1128/IAI.00504-15.
- Cahoon LA, Freitag NE, Prehna G. A structural comparison of Listeria monocytogenes protein chaperones PrsA1 and PrsA2 reveals molecular features required for virulence. Mol Microbiol. 2016;101(1):42–61. doi:10.1111/mmi.13367.
- Zemansky J, Kline BC, Woodward JJ, Leber JH, Marquis H, Portnoy DA. Development of a mariner-based transposon and identification of Listeria monocytogenes determinants, including the peptidyl-prolyl isomerase PrsA2, that contribute to its hemolytic phenotype. J Bacteriol. 2009;191(12):3950–3964. doi:10.1128/JB.00016-09.
- Tsai HN, Hodgson DA. Development of a synthetic minimal medium for Listeria monocytogenes. Appl Environ Microbiol. 2003;69(11):6943–6945. doi:10.1128/AEM.69.11.6943-6945.2003.
- Portman JL, Dubensky SB, Peterson BN, Whiteley AT, Portnoy DA. Activation of the Listeria monocytogenes Virulence Program by a Reducing Environment. mBio. 2017;8(5).
- Sauer JD, Herskovits AA, O’Riordan MXD, Fischetti VA, Novick RP, Ferretti JJ, Portnoy DA, Braunstein M, Rood JI. Metabolism of the gram-positive bacterial pathogen listeria monocytogenes. Microbiol Spectr. 2019;7(4):7(4. doi:10.1128/microbiolspec.GPP3-0066-2019.
- Xayarath B, Marquis H, Port GC, Freitag NE. Listeria monocytogenesCtaP is a multifunctional cysteine transport-associated protein required for bacterial pathogenesis. Mol Microbiol. 2009;74(4):956–973. doi:10.1111/j.1365-2958.2009.06910.x.
- Chen M, Zhang J, Xia J, Sun J, Zhang X, Xu J, Deng S, Han Y, Jiang L, Song H, et al. Listeria monocytogenes GshF contributes to oxidative stress tolerance via regulation of the phosphoenolpyruvate-carbohydrate phosphotransferase system. Microbiol Spectr. 2023;11(5):5(e0236523). doi:10.1128/spectrum.02365-23.
- Berude JC, Kennouche P, Reniere ML, Portnoy DA. Listeria monocytogenes utilizes glutathione and limited inorganic sulfur compounds as sources of essential cysteine. Infect Immun; 2024. p. e0042223.
- Anaya-Sanchez A, Feng Y, Berude JC, Portnoy DA. Detoxification of methylglyoxal by the glyoxalase system is required for glutathione availability and virulence activation in Listeria monocytogenes. PLOS Pathog. 2021;17(8):e1009819. doi:10.1371/journal.ppat.1009819.
- Lam O, Wheeler J, Tang CM. Thermal control of virulence factors in bacteria: a hot topic. Virulence. 2014;5(8):852–862. doi:10.4161/21505594.2014.970949.
- Steinmann R, D P. Thermosensing to adjust bacterial virulence in a fluctuating environment. Future Microbiol. 2013;8(1):85–105. doi:10.2217/fmb.12.129.
- Leimeister-Wächter M, Domann E, Chakraborty T. The expression of virulence genes in listeria monocytogenes is thermoregulated. J Bacteriol. 1992;174(3):947–952. doi:10.1128/jb.174.3.947-952.1992.
- Guerreiro DN, Boyd A, O’Byrne CP. The stressosome is required to transduce low pH signals leading to increased transcription of the amino acid-based acid tolerance mechanisms in Listeria monocytogenes. Access Microbiol. 2022;4(9):cmi000455. doi:10.1099/acmi.0.000455.
- Cotter PD, Gahan CG, Hill C. A glutamate decarboxylase system protects Listeria monocytogenes in gastric fluid. Mol Microbiol. 2001;40(2):465–475. doi:10.1046/j.1365-2958.2001.02398.x.
- Cotter PD, Ryan S, Gahan CGM, Hill C. Presence of GadD1 glutamate decarboxylase in selected Listeria monocytogenes strains is associated with an ability to grow at low pH. Appl Environ Microbiol. 2005;71(6):2832–2839. doi:10.1128/AEM.71.6.2832-2839.2005.
- Karatzas KA, Suur L, O’Byrne CP. Characterization of the intracellular glutamate decarboxylase system: analysis of its function, transcription, and role in the acid resistance of various strains of Listeria monocytogenes. Appl Environ Microbiol. 2012;78(10):3571–3579. doi:10.1128/AEM.00227-12.
- O’Byrne CP, Karatzas KA. The role of sigma B (sigma B) in the stress adaptations of Listeria monocytogenes: overlaps between stress adaptation and virulence. Adv Appl Microbiol. 2008;65:115–140.
- Bavdek A, Kostanjšek R, Antonini V, Lakey JH, Dalla Serra M, Gilbert RJC, Anderluh G. pH dependence of listeriolysin O aggregation and pore-forming ability. FEBS J. 2012;279(1):126–141. doi:10.1111/j.1742-4658.2011.08405.x.
- Schuerch DWW-K, M E, Tweten RK. Molecular basis of listeriolysin O pH dependence. Proc Natl Acad Sci USA. 2005;102(35):12537–12542. doi:10.1073/pnas.0500558102.
- Xiong A, Cabrera SV, Jayaswal G, Jayaswal, Rk RK. Molecular characterization of the ferric-uptake regulator, fur, from staphylococcus aureus. Microbiol. 2000;146(3):659–668. doi:10.1099/00221287-146-3-659.
- Litwin CM, C S. Role of iron in regulation of virulence genes. Clin Microbiol Rev. 1993;6(2):137–149. doi:10.1128/CMR.6.2.137.
- Schaible UE, Kaufmann SH. Iron and microbial infection. Nat Rev Microbiol. 2004;2(12):946–953. doi:10.1038/nrmicro1046.
- Lungu B, Ricke SC, Johnson MG. Growth, survival, proliferation and pathogenesis of Listeria monocytogenes under low oxygen or anaerobic conditions: a review. Anaerobe. 2009;15(1–2):7–17. doi:10.1016/j.anaerobe.2008.08.001.
- Jin B, Newton SMC, Shao Y, Jiang X, Charbit A, Klebba PE. Iron acquisition systems for ferric hydroxamates, haemin and haemoglobin in Listeria monocytogenes. Mol Microbiol. 2006;59(4):1185–1198. doi:10.1111/j.1365-2958.2005.05015.x.
- McLaughlin HP, Hill C, Gahan CG. The impact of iron on Listeria monocytogenes; inside and outside the host. Curr Opin Biotechnol. 2011;22(2):194–199. doi:10.1016/j.copbio.2010.10.005.
- Seyoum Y, Baye K, Humblot C. Iron homeostasis in host and gut bacteria - a complex interrelationship. Gut Microbes. 2021;13(1):1–19. doi:10.1080/19490976.2021.1874855.
- Pizarro-Cerda J, Kuhbacher A, Cossart P. Entry of Listeria monocytogenes in mammalian epithelial cells: an updated view. Cold Spring Harb Perspect Med. 2012;2(11):a010009–a010009. doi:10.1101/cshperspect.a010009.
- Conte MPL, Petrone C, Polidoro G, Valenti M, Seganti P, L, Valenti P. Modulation of actA gene expression in Listeria monocytogenes by iron. J Med Microbiol. 2000;49(8):681–683. doi:10.1099/0022-1317-49-8-681.
- Drolia R, Tenguria S, Durkes AC, Turner JR, Bhunia AK. Listeria adhesion protein induces intestinal epithelial barrier dysfunction for bacterial translocation. Cell Host Microbe. 2018;23(4):470–484 e7. doi:10.1016/j.chom.2018.03.004.
- Chand D, Avinash VS, Yadav Y, Pundle AV, Suresh CG, Ramasamy S. Molecular features of bile salt hydrolases and relevance in human health. Biochim Biophys Acta Gen Subj. 2017;1861(1):2981–2991. doi:10.1016/j.bbagen.2016.09.024.
- Sauer JD, Sotelo-Troha K, von Moltke J, Monroe KM, Rae CS, Brubaker SW, Hyodo M, Hayakawa Y, Woodward JJ, Portnoy DA, et al. The N-ethyl-N-nitrosourea-induced Goldenticket mouse mutant reveals an essential function of Sting in the in vivo interferon response to Listeria monocytogenes and cyclic dinucleotides. Infect Immun. 2011;79(2):688–694. doi:10.1128/IAI.00999-10.
- Auerbuch V, Brockstedt DG, Meyer-Morse N, O’Riordan M, Portnoy DA. Mice lacking the type I interferon receptor are resistant to Listeria monocytogenes. J Exp Med. 2004;200(4):527–533. doi:10.1084/jem.20040976.
- Osborne SE, Sit B, Shaker A, Currie E, Tan JM, van Rijn J, Higgins DE, Brumell JH. Type I interferon promotes cell-to-cell spread of Listeria monocytogenes. Cell Microbiol. 2017;19(3).
- O’Connell RM, Saha SK, Vaidya SA, Bruhn KW, Miranda GA, Zarnegar B, Perry AK, Nguyen BO, Lane TF, Taniguchi T, et al. Type I interferon production enhances susceptibility to Listeria monocytogenes infection. J Exp Med. 2004;200(4):437–445. doi:10.1084/jem.20040712.
- Marquis H, Bouwer HG, Hinrichs DJ, Portnoy DA. Intracytoplasmic growth and virulence of Listeria monocytogenes auxotrophic mutants. Infect Immun. 1993;61(9):3756–3760. doi:10.1128/iai.61.9.3756-3760.1993.
- Ripio MT, Domínguez-Bernal G, Suárez M, Brehm K, Berche P, Vázquez-Boland J-A. Transcriptional activation of virulence genes in wild-type strains of Listeria monocytogenes in response to a change in the extracellular medium composition. Res Microbiol. 1996;147(5):371–384. doi:10.1016/0923-2508(96)84712-7.
- Mitchell MK, Ellermann M. Long chain fatty acids and virulence repression in intestinal bacterial pathogens. Front Cell Infect Microbiol. 2022 Jun 17;12:928503. doi:10.3389/fcimb.2022.928503.
- Desbois AP, Smith VJ. Antibacterial free fatty acids: activities, mechanisms of action and biotechnological potential. Appl Microbiol Biotechnol. 2010;85(6):1629–1642. doi:10.1007/s00253-009-2355-3.
- Wang S, Orsi RH, Tang S, Zhang W, Wiedmann M, Boor KJ. Phosphotransferase system-dependent extracellular growth of listeria monocytogenes is regulated by alternative sigma factors σ L and σ H. Appl Environ Microbiol. 2014;80(24):7673–7682. doi:10.1128/AEM.02530-14.
- Eylert E, Schär J, Mertins S, Stoll R, Bacher A, Goebel W, Eisenreich W. Carbon metabolism of Listeria monocytogenes growing inside macrophages. Mol Microbiol. 2008;69(4):1008–1017. doi:10.1111/j.1365-2958.2008.06337.x.
- Massillon D, Bollen M, De Wulf H, Overloop K, Vanstapel F, Van Hecke P, Stalmans W. Demonstration of a glycogen/glucose 1-phosphate cycle in hepatocytes from fasted rats. Selective inactivation of phosphorylase by 2-deoxy-2-fluoro-alpha-D-glucopyranosyl fluoride. J Biol Chem. 1995;270(33):19351–19356. doi:10.1074/jbc.270.33.19351.
- Joseph B, Mertins S, Stoll R, Schär J, Umesha KR, Luo Q, Müller-Altrock S, Goebel W. Glycerol metabolism and PrfA activity in Listeria monocytogenes. J Bacteriol. 2008;190(15):5412–5430. doi:10.1128/JB.00259-08.
- Monniot C, Zébré AC, Aké FMD, Deutscher J, Milohanic E. Novel listerial glycerol dehydrogenase- and phosphoenolpyruvate-dependent dihydroxyacetone kinase system connected to the pentose phosphate pathway. J Bacteriol. 2012;194(18):4972–4982. doi:10.1128/JB.00801-12.
- Milenbachs AA, Brown DP, Moors M, Youngman P. Carbon-source regulation of virulence gene expression in Listeria monocytogenes. Mol Microbiol. 1997;23(5):1075–1085. doi:10.1046/j.1365-2958.1997.2711634.x.
- Ake FM, Joyet P, Deutscher J, Milohanic E. Mutational analysis of glucose transport regulation and glucose-mediated virulence gene repression in Listeria monocytogenes. Mol Microbiol. 2011;81(1):274–293. doi:10.1111/j.1365-2958.2011.07692.x.
- Klarsfeld ADG, L P, Cossart P. Five Listeria monocytogenes genes preferentially expressed in infected mammalian cells: plcA, purH, purD, pyrE and an arginine ABC transporter gene, arpJ. Mol Microbiol. 1994;13(4):585–597.