ABSTRACT
Commensal microorganisms in the human gut produce numerous metabolites by using small molecules derived from the host or diet as precursors. Host or dietary lipid molecules are involved in energy metabolism and maintaining the structural integrity of cell membranes. Notably, gut microbes can convert these lipids into bioactive signaling molecules through their biotransformation and synthesis pathways. These microbiota-derived lipid metabolites can affect host physiology by influencing the body’s immune and metabolic processes. This review aims to summarize recent advances in the microbial transformation and host immunomodulatory functions of these lipid metabolites, with a special focus on fatty acids and steroids produced by our gut microbiota.
1. Introduction
Lipid molecules are an important source of energy for the human body and are also essential for maintaining the structural stability of cell membranes. At the same time, the structural diversity of lipid molecules determines their function as signaling molecules involved in many physiological and pathological processes in the body. Lipid molecules are not only derived from the diet or the host’s metabolic pathways but can also be synthesized and converted by the commensal microbial communities that colonize the intestinal mucosal surface. In recent years, the role of lipid molecules derived from symbiotic microorganisms in maintaining human health or in the progression of disease has attracted widespread research interest.
The intestinal mucosal immune system consists of a single layer of intestinal epithelial cells, innate and adaptive immune cells residing in the intraepithelial or lamina propria region, and immune cells within the gut-associated lymphoid tissues (GALTs) such as Peyer’s patches and mesenteric lymph nodes.Citation1,Citation2 Because the intestine is in close contact with external stimuli, the intestinal immune system must defend against foreign pathogens and develop tolerance to commensal microorganisms and food antigens. Thus, intestinal mucosal immune cells are distributed along the intestinal tract with strong regional heterogeneity, and their development and maturation are accompanied by the establishment of a symbiotic relationship between the human body and external microorganisms.Citation1,Citation2
Human symbiotic microorganisms include bacteria, fungi, viruses, and a small number of protists that live with us, and the collection of all these microbes and their genes is called the microbiome.Citation3,Citation4 A recent study estimated that the gut’s symbiotic microorganisms are dominated by bacteria, with thousands of species, and their number reaches 38 trillion, slightly more than the total number of cells in our body, which is 30 trillion.Citation5 Intestinal commensal bacterial species are dominated by 7 phyla: Bacteroidetes, Firmicutes, Proteobacteria, Verrucomicrobia, Actinobacteria, Fusobacteria, and Cyanobacteria.Citation3 As the “second genome” of the human body, the biosynthetic/transformational network encoded by the microbiome can convert natural precursors, including lipid molecules, etc., derived from the body or food into a variety of small molecules with host-modulating activities.Citation6,Citation7 Bioactive lipid molecules derived from symbiotic bacteria include structural lipid molecules and biotransformed lipid metabolites. The former mainly includes microbial membrane lipid components such as phospholipids, glycerolipids, glycolipids, sphingolipids, and sulfonolipids.Citation8,Citation9 The latter consists mainly of microbial lipid metabolites such as bile acids (BAs), cholesterol/hormone derivatives, and various short-chain or long-chain fatty acid (SCFA or LCFA) metabolites.Citation8,Citation9
2. The lipid diversity of gut microbiota
The structural lipids of symbiotic bacteria are critical for the integrity of their cell membranes, the stability of membrane proteins, the energy production of the electron transport chain, and the resistance to external environmental stresses. Currently, our understanding of microbial membrane lipid molecules comes mainly from intestinal Gram-negative bacterial species such as Escherichia coli, Bacteroides, Alistipes, and Prevotella, etc. Similar to the membrane lipid components of eukaryotes, the membrane lipids of symbiotic bacteria also include different types of phospholipid molecules, such as phosphoethanolamine (PE), phosphoglycerol (PG), phosphocholine (PC), phosphoserine (PS), and phosphoinositol (PI), or different types of glycerolipid molecules, such as diacylglycerol (DAG) and triacylglycerol (TAG).Citation10,Citation11 Other classes of lipids are restricted to specific bacterial taxa, such as the sphingolipid molecules found in Bacteroides strains, including ceramide-phosphoethanolamine (CerPE), ceramide-phosphoinositol (CerPI), dihydroceramide (DHCer), α-galactosylceramide (α-GC), sphinganine, and deoxy sphingolipids.Citation12 Gram-negative bacteria also synthesize saccharolipids not found in eukaryotes, such as lipopolysaccharides (LPS) or lipooligosaccharides (LOS), which are composed of acylated lipid groups (Lipid A) and variously sized head groups of sugar structures.Citation13,Citation14 Compared to host cell membrane lipids, microbial membrane lipids have unique structural features. For example, the length of their lipid acyl chains can be odd or even, and their ends can form a branched chain structure. The microbial lipid structures differ from those of eukaryotes, making these molecules likely to be recognized by the host’s innate immune system and to activate innate immune sensors such as Toll-like or C-type lectin receptors.Citation8,Citation9,Citation15–18 In addition, variations in the structure of microbial lipids, such as LPS or LOS, also determined the immunogenicity of these molecules.Citation18,Citation19 However, microbial structural lipids can also exert modulatory functions on the host immune system. For example, the sphingolipids from Bacteroides fragilis limited the number of gut-invariant natural killer T cells (iNKT) to prevent the inflammatory effects caused by their hyperactivation.Citation20,Citation21
Gut symbiotic microorganisms also convert natural precursors from host or dietary sources into various lipid metabolites, including fatty acids and steroid molecules. Gut microbes can produce a range of SCFAs (the FA chain contains 2–6 carbon atoms). Among them, SCFAs with linear chain structures such as acetic acid, propionic acid, and butyric acid are mainly derived from microbial fermentation of dietary fiber, while SCFAs with branched-chain structures such as 2-methyl-butyric acid, isobutyric acid, and isovaleric acid are mainly derived from the branched-chain amino acid (BCAA) metabolism pathway of microorganisms.Citation22 Microbial LCFAs (the FA chain contains more than 12 carbon atoms) have unique structural characteristics and originate from different types of biosynthetic or conversion pathways. For example, several iso- or anteiso-FAs have been synthesized from BCAA-derived α-keto acids, such as iso-15:0 FA or anteiso-15:0 FA.Citation23,Citation24 Microbes can also convert monounsaturated FAs to cyclopropane FAs, such as 9,10-methylene hexadecanoic acid, which activates the brain angiogenesis factor 1 (BAI1) receptor in host cells.Citation23,Citation25 In addition, intestinal bacteria can convert unsaturated fatty acids, such as linoleic acid (LA) or linolenic acid (LNA), to various FA isomers and their hydroxylated or oxidized intermediates via the LCFA isomerization pathway.Citation26 Microbes can also synthesize other long-chain lipid metabolites, such as fatty amide commendamide or serine dipeptide lipids, by forming an amide bond between amines and fatty acids,Citation27–30 or convert arachidonic acid into eicosanoids such as prostaglandins.Citation31 The microbial sterol lipids are mainly composed of various BA derivatives, including deconjugated BAs, secondary BAs, and newly discovered microbially conjugated BAs,Citation32–34 and various types of cholesterol/hormone derivatives, such as sulfonated cholesterol or estrogen, etc.Citation35–38
Microbial lipid molecules are associated with the diversity of the microbiome and host health outcomes. For instance, consuming dietary fiber increased gut microbial diversity and SCFA production.Citation39 Conversely, dietary fiber deprivation led to a loss of microbiota diversity and impaired host gut barrier functions.Citation40,Citation41 As fermentation end products, SCFAs have been shown to have various host modulatory functions. These include enhancing gut barrier function,Citation42,Citation43 maintaining gut motility,Citation44,Citation45 and controlling gut hormone release.Citation46–48 BAs have been reported to be the primary gut steroid molecules responsible for the maturation of the gut microbiota in newborns,Citation49 and microbial metabolism of BAs can alter host metabolic phenotypes.Citation50–52 In addition to modulating host metabolism, microbial lipids may also serve as immune signaling molecules in the gut mucosa, regulating the fate programming and functionality of different types of intestinal immune cells, as well as host mucosal homeostasis and disease susceptibility. Given the biomass and diversity of human symbiotic microorganisms, research on the biosynthetic pathways and structural identification of microbial lipid molecules is emerging. Here, we summarize recent advances in this field, focusing on the bacterial transformation pathways of various FAs and BAs and the immunomodulatory functions of the resulting metabolites. However, we should appreciate that bacterial structural lipids also interact with host immunological or metabolic pathways, which has been reviewed in detail elsewhere.Citation10,Citation12,Citation53
3. Microbial transformations of gut fatty acids
3.1. Short-chain fatty acids
Gut microbes ferment dietary fibers to produce SCFAs, especially acetic, propionic, and butyric acids.Citation39,Citation54 The levels of fermentable fibers in human diets may influence the SCFA pool in the gut.Citation39,Citation54 Gut microbes can break down the host’s indigestible fibers into monosaccharides such as hexoses, pentoses, fucose, and rhamnose, and then convert them to SCFAs through various enzymatic cascades.Citation54 For example, hexoses and pentoses can be metabolized into pyruvate, and one of the representative SCFAs, acetic acid, can be synthesized from pyruvate via either the acetyl-CoA or Wood-Ljungdahl pathway in bacteria. Pyruvate can also be metabolized into lactate or succinate, which are further converted to propionyl-CoA for propionic acid biosynthesis. Gut microbes also ferment deoxyhexose sugars such as fucose or rhamnose into propionic acid via the propanediol pathway. In addition, two molecules of acetyl-CoA can be subsequently reduced into butyryl-CoA for butyric acid biosynthesis. The microbial fermentation of proteins also contributes to the gut SCFA pool via the production of branched-SCFAs such as 2-methyl-butyric acid, isovaleric acid, and isobutyric acid, which are derived from BCAAs isoleucine, leucine, and valineCitation54 ().
Figure 1. Biotransformation and immunomodulation of microbial FAs in the intestine.
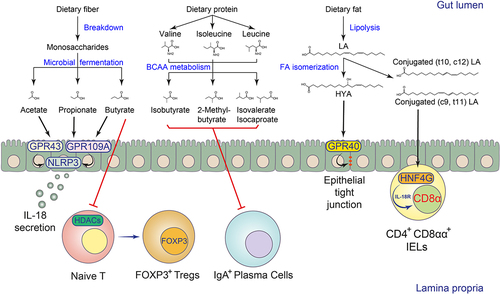
SCFAs can be produced by many types of gut microbes, including members of the genera Clostridium, Eubacterium, and Butyrivibrio.Citation55 However, due to the complexity of the microbiome, it remains difficult to elucidate the impact of different microbial SCFA pathways on specific end products. The application of microbial genetics technology can help us understand the impact of fermentation pathways in different microorganisms on SCFA biosynthesis. To this end, Guo et al. predicted the potential metabolic gene clusters for small molecule synthesis in Clostridium sporogenes and developed a CRISPR-Cas9-based genetic manipulation system for this species to validate key genes responsible for the production of SCFAs as well as other metabolites.Citation22 In this study, they confirmed that the production of propionate and butyrate in Clostridium sporogenes requires the catabolic threonine dehydratase TdcB and an enoyl-CoA hydratase homolog encoded by the talA and croA genes, respectively.Citation22 Intestinal Bacteroides species can also produce SCFAs. For example, in Bacteroides thetaiotaomicron, propionate production was mediated by the enzyme methylmalonyl-CoA mutase, which was also common in other enteric Bacteroides species.Citation56
In the bacterium Streptomyces avermitilis, branched SCFAs were converted from BCAAs by the branched-chain alpha-keto acid dehydrogenase (BCKDH) complex (EC 1.2.4.4).Citation57 However, the deletion of the BCKDH gene did not affect the production of branched SCFAs in Clostridium sporogenes.Citation22 On the other hand, the porA gene, which is closely related to α-ketoisovalerate:ferredoxin oxidoreductase (VOR) (EC 1.2.7.7),Citation58 was found to be essential. A mutation in the porA gene resulted in the cessation of 2-methylbutyrate, isobutyrate, and isovalerate production in Clostridium sporogenes. Additionally, a mutation in the hadB gene, which is involved in leucine fermentation, led to the loss of isocaproate production in this bacterium.Citation22 Mutations in both genes in Clostridium sporogenes were shown to alter the host intestinal IgA response in a monocolonized gnotobiotic mouse model, suggesting a modulatory function of these branched SCFAs on host immunity.Citation22 In a recent study, it was also found that the commensal Parabacteroides merdae produces branched SCFAs through the porA gene homolog.Citation59 Additionally, the degradation of BCAA by this bacterium was shown to reduce atherosclerotic lesions in animal modelsCitation59 ().
Table 1. The gut microbial biotransformation of lipid metabolites.
3.2. Long-chain fatty acids
The human body’s essential fatty acids include linoleic acid (LA, C18:2 c9, c12) and linolenic acid (LNA, C18:3 c9, c12, c15), which are the precursors of omega-6 and omega-3 polyunsaturated fatty acids, respectively. After dietary intake, they can form dihydroxy gamma-linolenic acid (DGLA) and arachidonic acid (AA), or eicosapentaenoic acid (EPA) and docosahexaenoic acid (DHA) through FA synthesis pathways in the host.Citation76 The host and microbes can further convert arachidonic acid to eicosanoid molecules such as prostaglandin derivatives.Citation31,Citation77 Symbiotic microorganisms also biotransform LA and LNA released from lipolysis, changing the position of their C=C unsaturated bonds in the fatty acid chain to form a series of microbially derived FA isomers.Citation26 The LA isomers, also known as conjugated LAs (CLAs), include c9, t11 CLA, t10, c12 CLA, and t8, t10 CLA, while the LNA isomers, also known as conjugated LNAs (CLNAs), include c9, t11, c15 CLNA and c9, t13, c15 CLNACitation26 ().
Microbial fatty acid isomerization is mainly mediated by linoleate isomerase (LAI) encoded by symbiotic microorganisms, which can convert LA and LNA to CLAs and CLNAs, respectively.Citation26 Sequence alignment shows that LAI is highly homologous to myosin cross-reactive antigen (MCRA). Both have hydratase activity and are involved in the synthesis of hydroxylated fatty acids.Citation26 In addition to LAI, research has shown that other proteins may also be involved in the fatty acid isomerization process, initiating a multi-step enzymatic reaction that ultimately changes the position of the C=C unsaturated bond to form fatty acid isomers.Citation60 Symbiotic microorganisms with LAI include Lactobacillus, Bifidobacterium, Enterococcus, Propionibacterium, and a small number of Clostridium species such as Clostridium sporogenes, etc.Citation26,Citation78
The microbial conversion of LA to CLAs also produces a variety of hydroxylated or oxidized fatty acid intermediates. For instance, Lactobacillus plantarum has been shown to produce fatty acid intermediates, such as 10-hydroxy-cis-12-octadecenoic acid, 10-hydroxyoctadecanoic acid, and 10-oxooctadecanoic acid, during the conversion of LA to CLAs. This biotransformation was mediated by four enzymes, namely CLA-HY (LAI), CLA-DH, CLA-DC, and CLA-ER, in Lactobacillus plantarum. Studies have also shown that germ-free (GF) mice have lower levels of these hydroxy fatty acids in their guts.Citation60,Citation61 Research on the biological activities of these microbially derived long-chain fatty acid intermediates is emerging. Recent studies have shown that microbial LA metabolism can reduce the obesity-related inflammatory response caused by excessive LA intake.Citation79 Mechanistically, 10-hydroxy-cis-12-octadecenoic acid (also known as HYA), a hydroxylated derivative of LA, activated the free fatty acid-sensing receptors GPR40 and GPR120 in the gut. The HYA-GPR40/GPR120 signaling then promoted the secretion of intestinal glucagon-like peptide-1 (GLP-1) to regulate host metabolic homeostasis.Citation79 Similarly, another oxidized LA metabolite, 10-oxo-cis-12-octadecenoic acid (KetoA), was shown to increase energy expenditure in mice via transient receptor potential vanilloid 1 (TRPV1).Citation80 A recent study also found that in addition to LA and LNA metabolites, other microbial-derived LCFA metabolites also have peroxisome proliferator-activated receptor (PPAR) agonist activity, suggesting that gut microbes, particularly lactic acid bacteria, may influence host physiology through a variety of LCFA metabolites.Citation81
In addition to modifying the fatty acyl chain, bacteria can conjugate various amine groups to the carboxyl group of LCFAs to generate fatty amides via N-acyl synthase (NAS).Citation28 Similar to host-derived fatty amines, these bacteria-derived fatty amines can also serve as GPCR agonists. For example, both host endogenous N-hexadecanoyl glycine and commensal bacteria-derived N-3-hydroxypalmitoyl glycine (as known as Commendamide) or N-myristoyl alanine activated GPR132/G2A, a GPCR with immunomodulatory functions.Citation28,Citation29,Citation82,Citation83 Bacterial N-palmitoyl serinol could activate GPR119, a GPCR involved in intestinal GLP-1 production, similar to host N-9-octadecenoyl ethanolamine.Citation28,Citation84 GF mice colonized with N-palmitoyl serinol-producing E. coli have been reported to have decreased levels of blood glucose due to increased GLP-1 secretion.Citation28 Bacterial fatty amines also exhibit distinct agonist selectivity or even receptor antagonist properties compared to host ligands for the same type of GPCRs. For example, bacterial N-3-hydroxypalmitoyl ornithine specifically activated sphingosine-1-phosphate receptor (S1PR) 4, while the host ligand, S1P, activated all five members of the S1PR family.Citation28 In addition, microbial N-acyloxyacyl glutamine has been shown to inhibit both prostaglandin I2 receptor (PTGIR) and prostaglandin E receptor 4 (PTGER4)Citation28 ().
4. Immunomodulation of microbial SCFAs
4.1. Innate immunity
SCFAs have been shown to affect different cell types involved in innate immunity via several metabolite-sensing receptors such as GPR41, GPR43, and GPR109A or histone deacetylases (HDACs) inhibition.Citation54 Intestinal epithelial cells (IECs) form a physical and biochemical defense barrier that separates host tissues from commensal microorganisms.Citation85 SCFAs have been shown to promote intestinal epithelial integrity via the NLRP3 inflammasome activation and IL-18 release.Citation86 The barrier-promoting effects of SCFAs require epithelial GPR43 and GPR109A signaling and downstream potassium ion efflux and ERK phosphorylationCitation86,Citation87 (). Secretory IECs such as goblet cells and Paneth cells support barrier function by producing mucins and antimicrobial peptides. One recent study showed that the SCFA propionate promotes intestinal goblet cell differentiation and mucus-relevant gene expression via epithelial GPR41 signaling.Citation56
Macrophages are classic innate immune cells that can engulf invading pathogens and senescent or damaged host cells, present antigens to T cells, and mount proper immune responses to maintain tissue homeostasis. Macrophages express a myriad of pattern recognition receptors (PRRs) to recognize pathogen or damage-associated molecular patterns (PAMPs or DAMPs). In response to different stimuli present in the microenvironment, macrophages can be polarized into inflammatory macrophages (M1) or alternatively activated macrophages (M2). Several reports support the anti-inflammatory role of SCFAs in modulating macrophage activity in both in vitro and in vivo models. A study by Pamela et al. showed that butyrate suppresses LPS-induced IL-6, iNOS, and IL-12, but not TNF-α or MCP-1 production by colonic CX3CR1hiCD103− macrophages.Citation88 This effect was independent of either TLR4 or GPR109A signaling but was due to the inhibition of HDACs by butyrate.Citation88 However, another study found that butyrate-induced expression of IL-10 and ALDH1A1 requires GPR109A signaling in intestinal macrophages and dendritic cells (DCs).Citation89 These observations suggest that the anti-inflammatory function of butyrate in macrophages may be gene and cell-type context-dependent. In addition to resolving inflammation, SCFAs have been reported to play beneficial roles in antibacterial and antiviral immunity. Schulthess et al. found that macrophages differentiated in vitro in the presence of butyrate exhibit enhanced antimicrobial activity without increased inflammatory responses.Citation90 Butyrate imprinted the antimicrobial state through HDAC3 inhibition, driving metabolic switch and LC3-associated phagocytosis in macrophages for enhanced microbicidal function against Salmonella infection in vivo.Citation90 Butyrate-induced HDAC3 inhibition in macrophages has also been implicated in an antimicrobial program to control Staphylococcus aureus-induced mastitis.Citation91 Microbiota-derived acetate has been reported to induce antiviral responses via GPR43-mediated type I interferon production.Citation92,Citation93
Innate lymphoid cells, including ILC1, ILC2, and ILC3, are characterized by the expression of signature transcriptional factors and cytokines similar to their T helper cell counterparts. ILCs are primarily found in mucosal tissues such as the gut, lung, and skin and are therefore putative responders to commensal-derived metabolites. Two groups reported that ILCs, particularly ILC2 and ILC3, express the SCFA receptor GPR43 at high levels.Citation94,Citation95 Activation of GPR43 by SCFAs or the chemical agonist selectively promoted ILC3 expansion and IL-22 expression. Mice deficient in Gpr43 had less IL-22+ ILC3 and were consequently more susceptible to dextran sulfate sodium (DSS)-induced colitis and Citrobacter rodentium infection.Citation94 Mechanistically, SCFAs triggered the expression of IL-22 in ILC3 via AKT and STAT3 signaling.Citation94 Similarly, acetate was reported to coordinate ILC3 responses against Clostridioides difficile infection by promoting the expression of IL-1 R in ILC3, which further facilitated IL-22 production in response to IL-1β stimulation.Citation96 However, in the context of the ILC2 scenario, butyrate was reported to inhibit HDCA activity, thus downregulating the expression of type 2 cytokines IL-5 and IL-13 in ILC2 to alleviate allergic inflammation.Citation97
4.2. Adaptive immunity
The immunomodulatory role of SCFAs in adaptive immunity has also been demonstrated (). Effector CD4+ T cells are critical for host defense and the development of autoimmune diseases, while FOXP3+ regulatory T cells (Tregs) are immunosuppressive T cells that maintain immune homeostasis. GF mice, which lack all commensal microorganisms, were reported to be defective in colonic Treg development, whereas microbiota colonization restored Treg levels.Citation98,Citation99 Supplementation of SCFAs in drinking water normalized Treg numbers in GF mice and increased IL-10 expression in Tregs. This effect was GPR43 dependent, as SCFAs failed to restore Treg numbers in Gpr43-deficient mice.Citation100 Yukihiro et al. also found that butyrate induces the differentiation of colonic Tregs by upregulating histone H3 acetylation levels at both the promoter and conserved non-coding sequence regions (CNS1 and CNS3) of the Foxp3 gene locus.Citation101 In addition, a third study showed that butyrate and propionate could increase extrathymic CNS1-dependent differentiation of Tregs via their HDAC inhibitory activity.Citation102 Recently, pentanoate, also known as valerate, a less characterized SCFA, was also shown to promote c-Maf-expressing colonic Treg differentiation via increasing iron uptake.Citation103 Besides Tregs, SCFAs were also found to regulate the functions of effector CD4+ T cells. In a naïve T cell differentiation system, SCFAs could promote Th1 and Th17 differentiation. Interestingly, these SCFA-differentiated effector T cells were also mixed with IL-10-producing CD4+ T cells. Similarly, SCFAs inhibited HDAC activity to enhance mTOR-S6K signaling to increase the expression of the effector T cell cytokines.Citation104 A higher concentration of butyrate induced Th1-like IFN-γ production in either Treg or conventional T effector cells. This effect was mediated by HDAC inhibition of butyrate and H3 acetylation at the Tbx21 and Ifng locus and was independent of the SCFA receptors GPR41 and GPR43.Citation105 Later, two studies found that butyrate signals through GPR43 to induce IL-10 production in Th1 cells or through GPR41 and HDAC inhibition to promote IL-22 production in CD4+ T cells, requiring downstream activation of STAT3 and mTOR in these cells.Citation106,Citation107
CD8+ T cells are important for the control of intracellular pathogens and tumor growth. Serum acetate levels were found to increase rapidly after bacterial infection. Upon uptake by memory CD8+ T cells, acetate increased the acetyl-coenzyme A (acetyl-CoA) pool and promoted the acetylation of GAPDH and glycolysis rate, which further facilitated the rapid memory CD8+ T response and IFN-γ production to control Listeria monocytogenes infection.Citation108 The transition of activated CD8+ T cells into memory cells has also been reported to be microbiota-dependent. High-fiber diet (HFD)-induced butyrate could promote the memory potential of activated CD8+ T cells via GPR41 and GPR43. Mechanistically, butyrate mediated metabolic rewiring of activated CD8+ T cells and facilitated sustained glutamine utilization and fatty acid oxidation.Citation109 HFD-derived butyrate has been shown to enhance the CD8+ T cell response to influenza infection via the GPR41 receptor and altered cellular metabolism.Citation110 SCFAs could also directly induce IFN-γ expression in CD8+ cytotoxic T cells (CTLs) and CD8+IL-17+ T cells (Tc17) by inhibiting HDAC activity, similar to their effect on CD4+ T cells.Citation111 Recent studies suggest a strong link between the microbiome and antitumor therapy. It was shown that antibiotic-treated mice were less responsive to anticancer chemotherapy, and butyrate supplementation restored their response to chemotherapy.Citation112 Butyrate upregulated the ID2-IL-12R cascade through the epigenetic remodeling of CD8+ T cells, which further promoted the functionality of CD8+ T cells to control tumor growth.Citation112 In addition, a similar role of pentanoate and butyrate in enhancing the anti-tumor activity of CTLs and chimeric antigen receptor (CAR) T cells has been reported.Citation113 By activating mTOR kinase and inhibiting HDAC activity, pentanoate and butyrate induced metabolic and epigenetic reprogramming of CTLs and CAR T cells to enhance TNF-α and IFN-γ production.Citation113 However, there are conflicting observations of SCFAs in anti-tumor immunity. Systemic administration of SCFAs was reported to limit the anti-tumor effect of CTLA-4 blockade, which was associated with increased Treg frequency in the periphery. In addition, butyrate also inhibited CD80/CD86 expression on DCs, which impeded T cell priming and activation.Citation114 The discrepancy in the conflicting results may be due to differences in experimental settings, such as anti-tumor therapy approaches, mouse tumor models, or SCFA doses/types used in each study. These parameters not only directly affect CD8+ T cell responses, but also likely affect other cell types that inhibit tumor killing, and thus in combination may create different in vivo biological contexts during antitumor therapy, leading to discrepant cancer therapy outcomes.
B cells can produce immunoglobulins (Ig) against pathogens and commensals to maintain intestinal homeostasis. SCFAs could directly increase acetyl-CoA levels and promote overall metabolic fitness including glycolysis, oxidative phosphorylation, and fatty acid synthesis to fuel plasma cell differentiation and IgA and IgG production. In addition to metabolic regulation, SCFAs also inhibited HDACs to upregulate the expression of Prdm1, Aicda, and Xbp1, which were positively associated with B cell differentiation.Citation115 SCFAs may also indirectly promote antibody production by regulating DC function. Acetate was shown to increase IgA-producing B cells in the intestine of wild-type but not Gpr43-knockout mice. They further suggested that acetate increases the expression of Aldh1a2 in DCs, a key enzyme that converts vitamin A to retinoid acid, and promotes IgA production by B cells.Citation116 However, a recent study showed that the SCFAs butyrate and propionate differentially regulate the antibody response in a dose-dependent manner. While low doses of SCFAs enhanced class-switch DNA recombination (CSR), high doses of SCFAs impaired Aicda and Prdm1 expression and CSR, leading to reduced antibody production. High-dose SCFAs acted through epigenetic upregulation of miRNAs that target Aicda and Prdm1 mRNA for degradation, thereby dampening B cell functionsCitation117 ().
Table 2. The immunomodulatory functions of microbial lipid metabolites.
5. Immunomodulation of microbial LCFAs
5.1. Innate immunity
LCFAs, such as LA and LNA, are essential polyunsaturated fatty acids (PUFAs) that people get from their diets. They may serve as precursors of omega-6 and omega-3 PUFAs, respectively. Both types of lipid mediators could modulate inflammation in a context-dependent manner. CLAs or CLNAs are microbial isomers of LA or LNA with anti-inflammatory properties that may benefit health.Citation26,Citation136 In vitro studies suggested that CLAs could modulate inflammatory cytokine production in myeloid cells. The cis-9, trans-11 isomer of CLAs (c9, t11-CLA) could inhibit IL-12 production in LPS-treated bone marrow-derived DCs. The c9, t11-CLA was shown to suppress NF-κB activation and promote IL-10 production through the ERK pathway.Citation118 HYA, a hydroxylated metabolite of LA, was found to improve epithelial barrier function via the GPR40-ERK pathway,Citation119,Citation120 while LNA derivatives such as 13-hydroxy-9(Z),15(Z)-octadecadienoic acid and 13-oxo-9(Z),15(Z)-octadecadienoic acid were also shown to induce anti-inflammatory M2 macrophage differentiation via the same signaling pathway.Citation121 Both CLAs and CLNAs, as well as these intermediate derivatives, have been reported to be potential agonists for PPARs,Citation81 likely protecting mice from DSS-induced colitis via activation of the PPAR-mediated pathways in macrophages.Citation137
In addition to their GPCR modulatory activities, microbial fatty amides can also activate host innate receptors. Porphyromonas gingivalis (P. gingivalis) is a periodontopathic pathogen involved in chronic periodontal disease.Citation138 P. gingivalis-derived fatty amides such as N-3-hydroxy-15-methylhexadecanoyl glycylserine (lipid 430) and N-15-methyl-3-((13-methyltetradecanoyl)oxy)hexadecanoyl glycylserine (lipid 654) contributed to periodontal inflammatory responses via activating host TLR2.Citation27 Another fatty amide lipid produced by P. gingivalis, phosphoglycerol serine-glycine lipodipeptide (lipid 1256), was a 50-fold more potent agonist in engaging TLR2 than lipid 654.Citation62
5.2. Adaptive immunity
The role of microbial LCFAs in modulating host adaptive immunity remains largely unexplored. We recently found that the gut microbial fatty acid isomerization pathway and its end products, CLAs, modulate the development of a subset of intraepithelial lymphocytes (IELs), termed CD4+CD8αα+ IELs, in the small intestineCitation78 (). Previous studies and our data suggest that the development of CD4+CD8αα+ IELs is dependent on microbial and dietary cues,Citation139–141 supported by the fact that GF or SPF mice fed a chemically simplified diet had impaired CD4+CD8αα+ IEL development. In the monocolonized gnotobiotic mouse model, only symbionts with intact LCFA isomerization pathways could restore CD4+CD8αα+ IEL numbers, suggesting that isomerization of LCFAs by microbes is required for the induction of CD4+CD8αα+ IELs. In addition, supplementation of CLAs in drinking water also normalized CD4+CD8αα+ IELs in mice fed a chemically simplified diet.Citation78 Mechanistically, we found that intestinal CD4+CD8αα+ IELs selectively express the transcription factor HNF4G, which can bind to CLAs and promote intrinsic Il18r1 transcription. While IL-18 signaling downregulated the CD4 lineage committed transcription factor ThPOK, thereby supporting CD4+CD8αα+ IEL development. Consequently, mice deficient in Hnf4g or Il18r1 had impaired CD4+CD8αα+ IEL development and were susceptible to Salmonella typhimurium infection.Citation78 The transcription factor HNF4A, analog to HNF4G, on the other hand, has been reported to promote the differentiation of natural IELs, namely TCRβ+CD8αα+ and TCRγδ+ cells, by regulating the expression of butyrophilin-like molecules in intestinal epithelial cells.Citation142 In addition to CLAs, the immunological functions of other microbial LCFAs, especially various intermediate metabolites, and their host sensing mechanisms in modulating host adaptive immunity need further investigation ().
6. Microbial transformations of gut steroids
6.1. Bile acids
Bile acids are sterol molecules formed from cholesterol through a cascade of enzymatic reactions in the liver. The two major primary bile acids synthesized by the human liver are cholic acid (CA) and chenodeoxycholic acid (CDCA). At the same time, these primary bile acids undergo a further conjugation reaction with glycine or taurine to form conjugated primary bile acids such as TCA, TCDCA, GCA, and GCDCA, etc., which are secreted from the liver into the gallbladder for storage.Citation34,Citation143 The gallbladder contracts in response to food intake, releasing bile acids into the small intestine to help the host absorb dietary fatty acids and fat-soluble vitamins. 95% of bile acids in the small intestine are reabsorbed by the liver via the enterohepatic circulation, while approximately 5% of bile acids enter the colon and are biotransformed by commensal microorganisms to microbial bile acid metabolites.Citation34,Citation143
The bile acid biotransformation pathways of symbiotic microorganisms include three main categories: 1) The bile acid deconjugation reaction mediated by bile salt hydrolase (BSH) converts the conjugated primary bile acids synthesized in the liver to deconjugated primary bile acids. Microbial BSHs belong to the choloylglycine hydrolase family (EC3.5.1.24) and can hydrolyze the C-24 N-acyl amide bond that links primary bile acids to their taurine and/or glycine conjugates. 2) Oxidation and epimerization of the 3-, 7-, or 12-hydroxyl group of bile acid molecules mediated by 3α/β-, 7α/β-, or 12α/β-hydroxysteroid dehydrogenases (HSDHs) to form various dehydrogenated or hydroxyl isomerized bile acid molecules. The conversion of bile acid hydroxy groups from α to β configuration involves a stable oxidized bile acid intermediate. These stereochemistry changes, mediated by microbial HSDHs, are also reversible from β to α configuration. The epimerization reaction can occur within a single bacterium that possesses both α and β-HSDHs or can be achieved through the coexistence of different bacteria, where one harbors α-HSDHs while the other has β-HSDHs. 3) The 7α-dehydroxylation pathway removes the 7α-hydroxy or 7β-hydroxy group of primary bile acids to form secondary bile acids, including deoxycholic acid (DCA) and lithocholic acid (LCA). The process requires a bile acid-inducible operon called Bai, which consists of eight genes.Citation34,Citation143–145 Recently, researchers have discovered that intestinal microorganisms can also mediate novel bile acid conjugation reactions that are independent of glycine or taurine, forming phenylalanocholic acid, tyrosocholic acid, or leucocholic acid, and other novel conjugated BAs.Citation33,Citation133,Citation146,Citation147 Subsequent studies have shown that microbial BSHs not only have hydrolase activity, but can also serve as N-acyltransferases that conjugate amines to BAs, forming microbial conjugated BAs.Citation67,Citation68 In addition to amine-conjugated BAs, microbial acylated BAs such as 3-acetylated CA and 3-succinylated CA were also discovered by a click chemistry-based mass spectrometry approach.Citation50 The biosynthesis of 3-succinylated CA was mediated by the BA acyl synthetase for succinyl (BAS-suc) in Bacteroides uniformis.Citation50
The enzymatic diversity and specificity of microbial bile acid metabolism determine the relative abundance of different bile acid metabolites and shape the intestinal bile acid landscape.Citation52,Citation144,Citation148 Compared to the microbial 7α-dehydroxylation pathway for secondary bile acid production, the microbial pathways for bile acid deconjugation, oxidation, and epimerization are widely distributed in various intestinal commensal bacteria, including Bacteroides, Clostridium, Lactobacillus, Bifidobacterium, and others.Citation34,Citation144 The microbial secondary bile acid production pathway exists mainly in a small number of intestinal commensal Clostridium species such as Clostridium scindens and Clostridium leptum.Citation34 The biotransformation cascade is mediated by the 7α-dehydroxylation pathway, encoded by the Bai operon in Clostridium, which converts CA and CDCA to DCA and LCA, respectively. The enzymatic cascade and its bile acid intermediates of the 7α-dehydroxylation pathway have recently been elucidated.Citation32 The secondary bile acids DCA and LCA, in turn, undergo 3α/β-HSDH-mediated oxidation and epimerization to yield various bile acid derivatives, such as isoDCA, isoLCA, 3-oxoLCA, and isoalloLCA, which have recently been shown to have immunomodulatory functions in the gutCitation63,Citation65,Citation66 ( and ).
Figure 2. Biotransformation and immunomodulation of microbial BAs in the intestine.
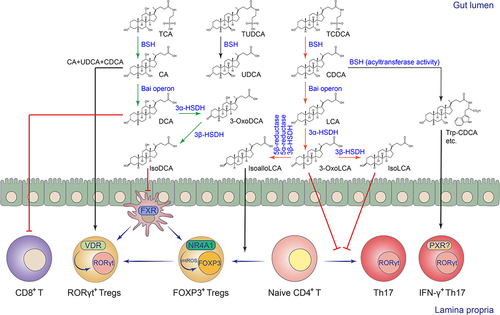
6.2. Cholesterol and hormones
Cholesterol is the chemical precursor for the synthesis of bile acids and other steroid molecules. Circulatory cholesterol levels are an important indicator of human health. Intestinal microorganisms have been shown to regulate serum cholesterol levels through microbial cholesterol metabolism. For example, recent metabolomics and bioinformatics studies found that certain uncultured members of Cluster IV Clostridium within our gut microbiota carry genes for cholesterol dehydrogenases, namely intestinal sterol metabolism A (ismA), which can convert cholesterol to coprostanol.Citation69,Citation70 Individuals harboring ismA-encoding bacteria had significantly lower fecal and serum cholesterol levels.Citation69,Citation70 In addition to cholesterol dehydrogenation, other gut bacteria such as Bacteroides have been shown to convert cholesterol to cholesterol-3-sulfate via their sulfotransferases (SULTs).Citation36,Citation38 The cholesterol sulfonation gene cluster in Bacteroides also sulfonated other steroid molecules such as isoalloLCA, etc.Citation38 Since LCA derivatives have been reported to be associated with healthy aging in centenarians,Citation149 and, as discussed below, with immunomodulatory activity, it is intriguing to investigate whether bacterial sulfonation alters their bioactivity and impacts our health.
Estrogens and androgens are important for host homeostasis and development. Gut microbes can metabolize them to various derivatives and thus affect systemic levels of sex steroid hormones. Under normal circumstances, estrogens are rapidly deactivated in the liver and eliminated from the body through urine and feces. Estrogens are inactivated by glycosidation, sulfation, or methylation of their sterol core structure.Citation150 However, gut microbes can remove the glucuronides or sulfates from steroids to reactivate estrogens via their deconjugation enzymes. The removal of glucuronides from deactivated estrogens was mediated by microbial β-glucuronidase (GUS) enzymes, which are abundant in human gut microbiota members such as Clostridium and Bacteroides.Citation72,Citation151 One study has reported heterologous expression of 35 gut microbiota-derived GUS enzymes in E. coli, nearly half of which could reactivate estrone or estradiol by removal of glucuronides.Citation71 Gut microbes have also been shown to deconjugate sulfated estrogens or sulfonate estrogen-related molecules via opposite enzymatic reactions. For example, Bacteroides fragilis or Peptococcus niger encoded sulfatases (SULF) that convert estrone sulfate to estrone,Citation73,Citation74 while Bacteroides thetaiotaomicron sulfonated estrogen precursor dehydroepiandrosterone (DHEA) via its sulfotransferase.Citation38 Similar to estrogens, androgens could be inactivated by conjugation, while gut bacteria could degrade them to pyruvate and propionyl-CoA for energy production via the super pathway of testosterone and androsterone degradation.Citation152,Citation153 In addition, Mycobacterium neoaurum-derived 3β-HSDH has been reported to convert testosterone to androstenedione, causing a depression-like phenotype in rats.Citation75 Endogenous hormone levels are closely associated with certain types of metabolic syndromes and cancers, while the impact of microbe-mediated hormone metabolism on the host immune system requires further investigation ().
7. Immunomodulation of microbial BAs
7.1. Innate immunity
The gut microbiota-derived BA metabolites have been reported to regulate several types of innate immune cells through either nuclear or membrane receptors. In general, intestinal BAs at physiological concentrations can promote IEC proliferation and epithelial integrity.Citation154 Although exceeding levels of microbial-deconjugated BAs may increase epithelial permeability, host-conjugated BAs sequester these microbial BAs to form micelles, and thus maintain gut integrity.Citation122 However, consumption of a Western diet (WD) led to overproduction of the secondary bile acid DCA, resulting in host intestinal Paneth cell dysfunction. Mechanistically, WD consumption increased the abundance of the BaiCD operon in the gut microbiome, and the resulting higher level of DCA promoted intestinal type I IFN response and Paneth cell defects in an FXR-dependent manner.Citation123 Microbial bile acid-mediated signaling were also reported to negatively regulate the innate immune response in macrophages or DCs. For example, the BA receptor FXR has been shown to be directly involved in the negative regulation of NF-κB and AP-1 target genes.Citation124 Activated FXR entered the nucleus and controlled the LPS-induced inflammatory response by stabilizing the inhibitory effect of the NCoR complex on the promoter region of inflammation-related genes.Citation124 In addition to FXR, microbial BAs have been reported to inhibit LPS-induced inflammatory factor expression through cAMP signaling mediated by the membrane receptor TGR5.Citation125 Mechanistically, a recent study found that the secondary BA LCA activates TGR5-cAMP-PKA signaling to phosphorylate serine at position 291 of the NLRP3 protein, leading to ubiquitination of NLRP3 and inhibition of inflammasome activation in bone marrow-derived macrophages.Citation126 Another study reported that the secondary BA DCA activates the same pathway, leading to the inhibition of NF-κB activation in human monocyte-derived DCs.Citation127 However, DCA has also been reported to activate the NLRP3 inflammasome by increasing calcium influx in THP-1-derived macrophages.Citation128 The contrary observations suggest the context-dependent biological function of secondary bile acids. Nevertheless, the BA receptor FXR was shown to inhibit the NLRP3 inflammasome via physical interaction with NLRP3 and CASP1 in the study.Citation128 Although dietary fiber may have beneficial anti-inflammatory effects via microbially fermented SCFAs, a recent study showed that inulin fiber alters the structure of the gut microbiota in mice, leading to an increase of a deconjugated primary BA, CA, which induces FXR-dependent IL-33 production, ILC2 activation and type 2 inflammationCitation129 ().
7.2. Adaptive immunity
Emerging evidence also suggests that microbial bile acid metabolites are important regulators of the differentiation and function of adaptive immune cells, particularly CD4+ T cells, via receptor-dependent or independent mechanisms (). The intestinal CD4+ T cell subsets, such as Th17 and Treg cells, are critical for intestinal anti-infection immunity and maintenance of intestinal immune homeostasis.Citation2 Two BA derivatives of LCA, 3-oxoLCA, and isoalloLCA, have been reported to regulate the differentiation and development of intestinal Th17 and Treg cells, respectively.Citation130 3-OxoLCA can inhibit the differentiation and development of Th17 cells by directly binding to RORγt, a master transcription factor that controls Th17 cell fate.Citation130 In addition, some human symbiotic microorganisms from the Actinobacteria or Clostridia phyla, such as Eggerthella lenta and Ruminococcus gnavus, have been shown to convert LCA to 3-oxoLCA and isoLCA through their 3α/β-HSDHs, and isoLCA had similar regulatory effects in inhibiting Th17 cell differentiation.Citation65 IsoalloLCA, on the other hand, was shown to promote mitochondrial ROS (mtROS) generation in a BA receptor-independent manner, thereby enhancing the expression of the Treg cell signature transcription factor FOXP3 to promote Treg cell differentiation and development.Citation130 Mechanistically, the conserved non-coding sequence 3 (CNS3) in the enhancer region of the Foxp3 gene and the nuclear receptor NR4A1 have been reported to be involved in the isoalloLCA-induced Treg differentiation process.Citation66,Citation130 The study also showed that intestinal Bacteroides species can convert 3-oxoLCA to isoalloLCA via a multistep enzymatic reaction involving 5β-reductase, 5α-reductase, and 3β-HSDH.Citation66 Deconjugation of host-derived bile acids by microbial BSH is the gateway reaction for microbial BA metabolism. The metabolic activities of different BSHs ultimately determine the size and composition of the intestinal bile acid pool.Citation34 Our study revealed that the BSH pathway of Bacteroides is critical for maintaining the levels of colonic RORγt+ Treg cells, which have been shown to be mainly regulated by microbial colonization and play an important immunoregulatory function in the gut.Citation131,Citation155,Citation156 We found that microbial-derived bile acid metabolites maintain the levels of RORγt+ Treg cells in the gut mainly through the BA receptor VDR.Citation131 Intrinsic Vdr-deficiency dampened the RORγt-mediated transcriptional program in Treg cells, and predisposed mice to show exacerbated disease phenotypes in the DSS-induced colitis model.Citation131 Microbial BAs may not only directly control the fate of Treg cells in the gut, but also indirectly modulate their differentiation, development, or recruitment. For example, the 3α/β-HSDHs of Eggerthella lenta and Ruminococcus gnavus were reported to convert DCA to isoDCA.Citation64 IsoDCA, in turn, promoted the differentiation and development of peripheral Treg cells by inhibiting FXR-mediated gene expression in intestinal DCs.Citation63 More recently, several newly identified amine-conjugated BAs have been shown to activate the nuclear receptor PXR, and among them, Trp-CDCA in particular, has been shown to induce IFN-γ in RORγt+CD4+ effector T cells and is likely to contribute to the pathogenesis of Inflammatory bowel disease (IBD).Citation133 However, to minimize the cytotoxicity of high concentrations of bile acids in the intestine, the nuclear receptor CAR was activated in intestinal CD4+ effector T cells. This activation induced bile acid tolerance via the xenobiotic transporter MDR1 and controlled excessive intestinal inflammation via the immunosuppressive cytokine IL-10.Citation132 During the progression of colorectal cancer (CRC), the presence of Bacteroides-derived BSH elevated unconjugated DCA and LCA levels. This, in turn, resulted in TGR5-dependent CCL28 production in colon tumors. The chemokine CCL28 recruited intra-tumoral Tregs to dampen CD8+ T cell-mediated anti-tumor immunity.Citation134 Microbial bile acids can also directly modulate the activity of CD8+ T cells. A recent study demonstrated that DCA inhibits calcium-nuclear factor of activated T cells (NFAT)2 signaling by targeting plasma membrane Ca2+ ATPase (PMCA) in CD8+ T cells. As a result, microbial DCA suppressed CD8+ T cell effector function and promoted CRC development in mouse modelsCitation135 ().
These studies revealed that several microbial BA biotransformation pathways, including the BA deconjugation pathway, the 7α-dehydroxylation pathway that produces secondary BAs, and the hydroxyl oxidation and epimerization pathways for these secondary BAs, are all involved in the production of BA metabolites with immunomodulatory activity. Whether strategies targeting relevant bile acid metabolic enzymes or altering bile acid flux can be used to effectively intervene in intestinal inflammatory diseases remains to be investigated.
8. Concluding remarks
In this review, we discuss recent research progress on immune modulation of intestinal barrier function by fatty acid and bile acid metabolites derived from gut microorganisms. Both types of microbial metabolites have diverse structures and are involved in the regulation of host innate and adaptive immunity. The diversity and complexity of lipid molecules produced by the symbiotic microbes of the human gut have presented both great opportunities and challenges to our research community. In recent years, the development of high-throughput microbial sequencing technology, such as 16S ribosomal RNA and metagenomic sequencing, and metabolomics technology have allowed us to identify the structural composition of a given microbial community and its associated lipid metabolites. Furthermore, we can correlate such microbial signatures with host mucosal immune phenotypes using bioinformatics technology. However, it is still difficult to efficiently explore the causal relationship between the diverse microbial lipid metabolites and their corresponding immunomodulatory functions/mechanisms using omics technologies alone. Currently, we face several technical obstacles in studying the host modulatory functions of these microbial lipid molecules. For example, the host’s intestinal commensal microorganisms are very diverse, and some of the species still cannot be isolated and cultured in vitro, making it difficult to evaluate their lipid metabolism capabilities. Second, although microbial genetic manipulation technologies for commensal bacteria are developing, the genomes of a large number of symbiotic microorganisms cannot be precisely edited, limiting our understanding of how commensal bacteria metabolize different lipid molecules and the host modulatory functions of such microbial transformation pathways. Finally, due to the diversity of microbial lipid metabolites, it is still challenging to annotate the chemical structures of a large number of de novo microbial lipid metabolites using untargeted mass spectrometry (MS) technology, making it difficult to establish a relationship between an uncharacterized microbial lipid molecule and its host receptor or target molecule.
Although these research challenges limit our comprehensive understanding of how commensal microbial-derived lipid molecules interact with the host immune system at the cellular and molecular levels, we should also see ample opportunities for exploring new methods to isolate unculturable symbiotic microorganisms, developing microbial genetic tools to edit symbiotic microorganisms, and identifying novel bioactive microbial lipids. Despite the knowledge gained from microbial sequencing data, further experimental validation and functional studies require new isolation and cultivation methods for previously uncultured microorganisms.Citation157 Emerging microbial culturomics technologies, which integrate machine learning with automated platforms, have begun to fascinate the high-throughput cultivation of uncultured gut microbes.Citation158 Using this culturomics framework, the researchers obtained 26,997 microbial isolates, representing over 80% of all abundant taxa, from the fecal samples of 20 human participants.Citation158 This high-throughput microbial isolation platform, combined with high-resolution genomics data, will enable large-scale microbiome research. For a long time, gut Bacteroides have been used as representative commensal microorganisms to study host-microbiota interactions because they are easily cultured and genetically tractable.Citation159–161 With the success of the CRISPR/Cas system in genome editing of eukaryotic cells, new CRISPR/Cas-based microbial gene-editing tools have emerged in recent years, targeting various members of the human gut bacterial communities.Citation22–Citation162–164 Of note, gut bacteria belonging to the Firmicutes/Clostridia are the most abundant species in the human gut, yet the development of genetic manipulation tools for these species has lagged behind. In recent work, an integrated pipeline with CRISPRi and Group II intron technologies was developed that enables gene editing in gut Firmicutes/Clostridia commensals, providing an avenue for genetic manipulation and functional studies for a complex microbiota.Citation165 While bacterial genetics provides insight into microbial metabolic pathways, metabolomics directly measures the metabolic capacity of a given microorganism or community. MS-based metabolomic analysis can detect and quantify a large number of small molecules, but data analysis including feature detection and structure annotation remains challenging, especially for untargeted metabolomics.Citation166 A recently developed open-access MS analysis platform, Global Natural Products Social Molecular Networking (GNPS), allows researchers to share and explore spectrometry data with enhanced annotations.Citation167 Molecular networking connects mass spectra of molecules with similar fragment ion spectra, enabling meta-mass shift chemical profiling without knowledge of the chemical structures of related molecules.Citation168 Using GNPS-based MS informatics, the researchers identified uncharacterized amino acid-conjugated bile acids, such as tyrosocholic acid, phenylalanocholic acid, and leucocholic acid, produced by the mammalian gut microbiota with agonistic activity on host FXR signaling.Citation33 Once the chemical structure is revealed, researchers could apply reverse metabolomics to evaluate whether a given molecule is present in public untargeted metabolomics datasets and whether the molecule is associated with different biological parameters in each public dataset.Citation133
In conclusion, this review summarizes recent advances in microbial transformation pathways and the immunomodulatory roles of gut lipid metabolites, such as short-chain fatty acids, long-chain fatty acids, and bile acids. Both host and diet-derived lipid precursors can be metabolized into different microbial lipid derivatives via distinct biotransformation pathways within the human gut microbiome. The resulting products modulate both the innate and adaptive branches of the host’s immune system by targeting different types of immune cells through receptor-dependent or -independent mechanisms. As new tools for studying the microbiome continue to emerge, we will gain a better understanding of the lipid chemistry and biotransformations of individual gut microbes at a functional level. This will allow us to further dissect the underlying mechanisms of microbial-immune interactions that control our health and disease.
Disclosure statement
No potential conflict of interest was reported by the author(s).
Additional information
Funding
References
- Mowat AM, Agace WW. Regional specialization within the intestinal immune system. Nat Rev Immunol. 2014;14(10):667–24. doi:10.1038/nri3738.
- Agace WW, McCoy KD. Regionalized development and maintenance of the intestinal adaptive immune landscape. Immunity. 2017;46(4):532–548. doi:10.1016/j.immuni.2017.04.004.
- Human Microbiome Project C. Structure, function and diversity of the healthy human microbiome. Nature. 2012;486(7402):207–214.
- Sekirov I, Russell SL, Antunes LC, Finlay BB. Gut microbiota in health and disease. Physiol Rev. 2010;90(3):859–904. doi:10.1152/physrev.00045.2009.
- Sender R, Fuchs S, Milo R. Revised estimates for the number of human and bacteria cells in the body. PLOS Biol. 2016;14(8):e1002533. doi:10.1371/journal.pbio.1002533.
- Donia MS, Fischbach MA. HUMAN MICROBIOTA. Small molecules from the human microbiota. Science. 2015;349(6246):1254766. doi:10.1126/science.1254766.
- Krautkramer KA, Fan J, Backhed F. Gut microbial metabolites as multi-kingdom intermediates. Nat Rev Microbiol. 2021;19(2):77–94. doi:10.1038/s41579-020-0438-4.
- Brown EM, Clardy J, Xavier RJ. Gut microbiome lipid metabolism and its impact on host physiology. Cell Host & Microbe. 2023;31(2):173–186. doi:10.1016/j.chom.2023.01.009.
- Morozumi S, Ueda M, Okahashi N, Arita M. Structures and functions of the gut microbial lipidome. Biochim Biophys Acta Mol Cell Biol Lipids. 2022;1867(3):159110. doi:10.1016/j.bbalip.2021.159110.
- Ryan E, Joyce SA, Clarke DJ. Membrane lipids from gut microbiome-associated bacteria as structural and signalling molecules. Microbiol (Read). 2023;169(3). doi:10.1099/mic.0.001315.
- Sohlenkamp C, Geiger O. Bacterial membrane lipids: diversity in structures and pathways. FEMS Microbiol Rev. 2016;40(1):133–159. doi:10.1093/femsre/fuv008.
- Heaver SL, Johnson EL, Ley RE. Sphingolipids in host-microbial interactions. Curr Opin Microbiol. 2018;43:92–99. doi:10.1016/j.mib.2017.12.011.
- Di Lorenzo F, De Castro C, Silipo A, Molinaro A. Lipopolysaccharide structures of gram-negative populations in the gut microbiota and effects on host interactions. FEMS Microbiol Rev. 2019;43(3):257–272. doi:10.1093/femsre/fuz002.
- Jacobson AN, Choudhury BP, Fischbach MA, Relman DA. The biosynthesis of lipooligosaccharide from bacteroides thetaiotaomicron. mBio. 2018;9(2). doi:10.1128/mBio.02289-17.
- Bae M, Cassilly CD, Liu X, Park SM, Tusi BK, Chen X, Kwon J, Filipcik P, Bolze AS, Liu Z, et al. Akkermansia muciniphila phospholipid induces homeostatic immune responses. Nature. 2022;608(7921):168–173. doi:10.1038/s41586-022-04985-7.
- Imai T, Matsumura T, Mayer-Lambertz S, Wells CA, Ishikawa E, Butcher SK, Barnett TC, Walker MJ, Imamura A, Ishida H, et al. Lipoteichoic acid anchor triggers Mincle to drive protective immunity against invasive group a streptococcus infection. Proc Natl Acad Sci USA. 2018;115(45):E10662–E10671. doi:10.1073/pnas.1809100115.
- Nichols FC, Housley WJ, O’Conor CA, Manning T, Wu S, Clark RB. Unique lipids from a common human bacterium represent a new class of toll-like receptor 2 ligands capable of enhancing autoimmunity. Am J Pathol. 2009;175(6):2430–2438. doi:10.2353/ajpath.2009.090544.
- Vatanen T, Kostic AD, d’Hennezel E, Siljander H, Franzosa EA, Yassour M, Kolde R, Vlamakis H, Arthur TD, Hamalainen AM, et al. Variation in microbiome LPS immunogenicity contributes to autoimmunity in humans. Cell. 2016;165(4):842–853. doi:10.1016/j.cell.2016.04.007.
- Gauthier AE, Chandler CE, Poli V, Gardner FM, Tekiau A, Smith R, Bonham KS, Cordes EE, Shank TM, Zanoni I, et al. Deep-sea microbes as tools to refine the rules of innate immune pattern recognition. Sci Immunol. 2021;6(57). doi:10.1126/sciimmunol.abe0531.
- An D, Oh SF, Olszak T, Neves JF, Avci FY, Erturk-Hasdemir D, Lu X, Zeissig S, Blumberg RS, Kasper DL. Sphingolipids from a symbiotic microbe regulate homeostasis of host intestinal natural killer T cells. Cell. 2014;156(1–2):123–133. doi:10.1016/j.cell.2013.11.042.
- Oh SF, Praveena T, Song H, Yoo JS, Jung DJ, Erturk-Hasdemir D, Hwang YS, Lee CC, Le Nours J, Kim H, et al. Host immunomodulatory lipids created by symbionts from dietary amino acids. Nature. 2021;600(7888):302–307. doi:10.1038/s41586-021-04083-0.
- Guo CJ, Allen BM, Hiam KJ, Dodd D, Van Treuren W, Higginbottom S, Nagashima K, Fischer CR, Sonnenburg JL, Spitzer MH, et al. Depletion of microbiome-derived molecules in the host using clostridium genetics. Science. 2019;366(6471). doi:10.1126/science.aav1282.
- Colosimo DA, Kohn JA, Luo PM, Piscotta FJ, Han SM, Pickard AJ, Rao A, Cross JR, Cohen LJ, Brady SF. Mapping interactions of microbial metabolites with human G-Protein-coupled receptors. Cell Host Microbe. 2019;26(2):273–282 e277. doi:10.1016/j.chom.2019.07.002.
- Kaneda T. Iso- and anteiso-fatty acids in bacteria: biosynthesis, function, and taxonomic significance. Microbiol Rev. 1991;55(2):288–302. doi:10.1128/mr.55.2.288-302.1991.
- Hari SB, Grant RA, Sauer RT. Structural and functional analysis of E. coli cyclopropane fatty acid synthase. Structure. 2018;26(9):1251–1258 e1253. doi:10.1016/j.str.2018.06.008.
- Salsinha AS, Pimentel LL, Fontes AL, Gomes AM, Rodriguez-Alcala LM. Microbial production of conjugated linoleic acid and conjugated linolenic acid relies on a multienzymatic system. Microbiol Mol Biol Rev : MMBR. 2018;82(4). doi:10.1128/MMBR.00019-18.
- Clark RB, Cervantes JL, Maciejewski MW, Farrokhi V, Nemati R, Yao X, Anstadt E, Fujiwara M, Wright KT, Riddle C, et al. Serine lipids of porphyromonas gingivalis are human and mouse toll-like receptor 2 ligands. Infect Immun. 2013;81(9):3479–3489. doi:10.1128/IAI.00803-13.
- Cohen LJ, Esterhazy D, Kim SH, Lemetre C, Aguilar RR, Gordon EA, Pickard AJ, Cross JR, Emiliano AB, Han SM, et al. Commensal bacteria make GPCR ligands that mimic human signalling molecules. Nature. 2017;549(7670):48–53. doi:10.1038/nature23874.
- Cohen LJ, Kang HS, Chu J, Huang YH, Gordon EA, Reddy BV, Ternei MA, Craig JW, Brady SF. Functional metagenomic discovery of bacterial effectors in the human microbiome and isolation of commendamide, a GPCR G2A/132 agonist. Proc Natl Acad Sci USA. 2015;112(35):E4825–4834. doi:10.1073/pnas.1508737112.
- Dietz C, Clark RB, Nichols FC, Smith MB. Convergent synthesis of a deuterium-labeled serine dipeptide lipid for analysis of biological samples. J Labelled Comp Radiopharm. 2017;60(6):274–285. doi:10.1002/jlcr.3498.
- Lamacka M, Sajbidor J. The occurrence of prostaglandins and related compounds in lower organisms. Prostaglandins Leukot Essent Fat Acids. 1995;52(6):357–364. doi:10.1016/0952-3278(95)90062-4.
- Funabashi M, Grove TL, Wang M, Varma Y, McFadden ME, Brown LC, Guo C, Higginbottom S, Almo SC, Fischbach MA. A metabolic pathway for bile acid dehydroxylation by the gut microbiome. Nature. 2020;582(7813):566–570. doi:10.1038/s41586-020-2396-4.
- Quinn RA, Melnik AV, Vrbanac A, Fu T, Patras KA, Christy MP, Bodai Z, Belda-Ferre P, Tripathi A, Chung LK, et al. Global chemical effects of the microbiome include new bile-acid conjugations. Nature. 2020;579(7797):123–129. doi:10.1038/s41586-020-2047-9.
- Ridlon JM, Kang DJ, Hylemon PB. Bile salt biotransformations by human intestinal bacteria. J Lipid Res. 2006;47(2):241–259. doi:10.1194/jlr.R500013-JLR200.
- Jan HM, Chen YC, Shih YY, Huang YC, Tu Z, Ingle AB, Liu SW, Wu MS, Gervay-Hague J, Mong KT, et al. Metabolic labelling of cholesteryl glucosides in Helicobacter pylori reveals how the uptake of human lipids enhances bacterial virulence. Chem Sci. 2016;7(9):6208–6216. doi:10.1039/C6SC00889E.
- Le HH, Lee MT, Besler KR, Comrie JMC, Johnson EL. Characterization of interactions of dietary cholesterol with the murine and human gut microbiome. Nat Microbiol. 2022;7(9):1390–1403. doi:10.1038/s41564-022-01195-9.
- Pellock SJ, Redinbo MR. Glucuronides in the gut: sugar-driven symbioses between microbe and host. J Biol Chem. 2017;292(21):8569–8576. doi:10.1074/jbc.R116.767434.
- Yao L, D’Agostino GD, Park J, Hang S, Adhikari AA, Zhang Y, Li W, Avila-Pacheco J, Bae S, Clish CB, et al. A biosynthetic pathway for the selective sulfonation of steroidal metabolites by human gut bacteria. Nat Microbiol. 2022;7(9):1404–1418. doi:10.1038/s41564-022-01176-y.
- Makki K, Deehan EC, Walter J, Backhed F. The impact of dietary fiber on gut microbiota in host health and disease. Cell Host & Microbe. 2018;23(6):705–715. doi:10.1016/j.chom.2018.05.012.
- Desai MS, Seekatz AM, Koropatkin NM, Kamada N, Hickey CA, Wolter M, Pudlo NA, Kitamoto S, Terrapon N, Muller A, et al. A dietary fiber-deprived gut microbiota degrades the colonic mucus barrier and enhances pathogen susceptibility. Cell. 2016;167(5):1339–1353 e1321. doi:10.1016/j.cell.2016.10.043.
- Sonnenburg ED, Smits SA, Tikhonov M, Higginbottom SK, Wingreen NS, Sonnenburg JL. Diet-induced extinctions in the gut microbiota compound over generations. Nature. 2016;529(7585):212–215. doi:10.1038/nature16504.
- Lewis K, Lutgendorff F, Phan V, Soderholm JD, Sherman PM, McKay DM. Enhanced translocation of bacteria across metabolically stressed epithelia is reduced by butyrate. Inflamm Bowel Dis. 2010;16(7):1138–1148. doi:10.1002/ibd.21177.
- Peng L, Li ZR, Green RS, Holzman IR, Lin J. Butyrate enhances the intestinal barrier by facilitating tight junction assembly via activation of AMP-activated protein kinase in caco-2 cell monolayers. J Nutr. 2009;139(9):1619–1625. doi:10.3945/jn.109.104638.
- Cherbut C, Ferrier L, Roze C, Anini Y, Blottiere H, Lecannu G, Galmiche JP. Short-chain fatty acids modify colonic motility through nerves and polypeptide YY release in the rat. Am J Physiol. 1998;275(6):G1415–1422. doi:10.1152/ajpgi.1998.275.6.G1415.
- Soret R, Chevalier J, De Coppet P, Poupeau G, Derkinderen P, Segain JP, Neunlist M. Short-chain fatty acids regulate the enteric neurons and control gastrointestinal motility in rats. Gastroenterology. 2010;138(5):1772–1782. doi:10.1053/j.gastro.2010.01.053.
- Fukumoto S, Tatewaki M, Yamada T, Fujimiya M, Mantyh C, Voss M, Eubanks S, Harris M, Pappas TN, Takahashi T. Short-chain fatty acids stimulate colonic transit via intraluminal 5-HT release in rats. Am J Physiol Regul Integr Comp Physiol. 2003;284(5):R1269–1276. doi:10.1152/ajpregu.00442.2002.
- Martin AM, Sun EW, Rogers GB, Keating DJ. The influence of the gut microbiome on host metabolism through the regulation of gut hormone release. Front Physiol. 2019;10:428. doi:10.3389/fphys.2019.00428.
- Yano JM, Yu K, Donaldson GP, Shastri GG, Ann P, Ma L, Nagler CR, Ismagilov RF, Mazmanian SK, Hsiao EY. Indigenous bacteria from the gut microbiota regulate host serotonin biosynthesis. Cell. 2015;161(2):264–276. doi:10.1016/j.cell.2015.02.047.
- van Best N, Rolle-Kampczyk U, Schaap FG, Basic M, Olde Damink SWM, Bleich A, Savelkoul PHM, von Bergen M, Penders J, Hornef MW. Bile acids drive the newborn’s gut microbiota maturation. Nat Commun. 2020;11(1):3692. doi:10.1038/s41467-020-17183-8.
- Nie Q, Luo X, Wang K, Ding Y, Jia S, Zhao Q, Li M, Zhang J, Zhuo Y, Lin J, et al. Gut symbionts alleviate MASH through a secondary bile acid biosynthetic pathway. Cell. 2024;187(11):2717–2734.e33. doi:10.1016/j.cell.2024.03.034.
- Sayin SI, Wahlstrom A, Felin J, Jantti S, Marschall HU, Bamberg K, Angelin B, Hyotylainen T, Oresic M, Backhed F. Gut microbiota regulates bile acid metabolism by reducing the levels of tauro-beta-muricholic acid, a naturally occurring FXR antagonist. Cell Metab. 2013;17(2):225–235. doi:10.1016/j.cmet.2013.01.003.
- Yao L, Seaton SC, Ndousse-Fetter S, Adhikari AA, DiBenedetto N, Mina AI, Banks AS, Bry L, Devlin AS. A selective gut bacterial bile salt hydrolase alters host metabolism. Elife. 2018;7. doi:10.7554/eLife.37182.
- Di Lorenzo F, Duda KA, Lanzetta R, Silipo A, De Castro C, Molinaro A. A journey from structure to function of bacterial lipopolysaccharides. Chem Rev. 2022;122(20):15767–15821. doi:10.1021/acs.chemrev.0c01321.
- Koh A, De Vadder F, Kovatcheva-Datchary P, Backhed F. From dietary fiber to host physiology: short-chain fatty acids as key bacterial metabolites. Cell. 2016;165(6):1332–1345. doi:10.1016/j.cell.2016.05.041.
- Mann ER, Lam YK, Uhlig HH. Short-chain fatty acids: linking diet, the microbiome and immunity. Nat Rev Immunol. 2024; doi:10.1038/s41577-024-01014-8.
- Wang X, Cai Z, Wang Q, Wu C, Sun Y, Wang Z, Xu X, Xue W, Cao Z, Zhang M, et al. Bacteroides methylmalonyl-CoA mutase produces propionate that promotes intestinal goblet cell differentiation and homeostasis. Cell Host Microbe. 2024;32(1):63–78 e67. doi:10.1016/j.chom.2023.11.005.
- Denoya CD, Fedechko RW, Hafner EW, McArthur HA, Morgenstern MR, Skinner DD, Stutzman-Engwall K, Wax RG, Wernau WC. A second branched-chain alpha-keto acid dehydrogenase gene cluster (bkdFGH) from Streptomyces avermitilis: its relationship to avermectin biosynthesis and the construction of a bkdF mutant suitable for the production of novel antiparasitic avermectins. J Bacteriol. 1995;177(12):3504–3511. doi:10.1128/jb.177.12.3504-3511.1995.
- Zhu Y, Dwidar M, Nemet I, Buffa JA, Sangwan N, Li XS, Anderson JT, Romano KA, Fu X, Funabashi M, et al. Two distinct gut microbial pathways contribute to meta-organismal production of phenylacetylglutamine with links to cardiovascular disease. Cell Host Microbe. 2023;31(1):18–32 e19. doi:10.1016/j.chom.2022.11.015.
- Qiao S, Liu C, Sun L, Wang T, Dai H, Wang K, Bao L, Li H, Wang W, Liu SJ, et al. Gut parabacteroides merdae protects against cardiovascular damage by enhancing branched-chain amino acid catabolism. Nat Metab. 2022;4(10):1271–1286. doi:10.1038/s42255-022-00649-y.
- Kishino S, Park SB, Takeuchi M, Yokozeki K, Shimizu S, Ogawa J. Novel multi-component enzyme machinery in lactic acid bacteria catalyzing C=C double bond migration useful for conjugated fatty acid synthesis. Biochem Biophys Res Commun. 2011;416(1–2):188–193. doi:10.1016/j.bbrc.2011.11.022.
- Kishino S, Takeuchi M, Park SB, Hirata A, Kitamura N, Kunisawa J, Kiyono H, Iwamoto R, Isobe Y, Arita M, et al. Polyunsaturated fatty acid saturation by gut lactic acid bacteria affecting host lipid composition. Proc Natl Acad Sci USA. 2013;110(44):17808–17813. doi:10.1073/pnas.1312937110.
- Nichols FC, Clark RB, Maciejewski MW, Provatas AA, Balsbaugh JL, Dewhirst FE, Smith MB, Rahmlow A. A novel phosphoglycerol serine-glycine lipodipeptide of porphyromonas gingivalis is a TLR2 ligand. J Lipid Res. 2020;61(12):1645–1657. doi:10.1194/jlr.RA120000951.
- Campbell C, McKenney PT, Konstantinovsky D, Isaeva OI, Schizas M, Verter J, Mai C, Jin WB, Guo CJ, Violante S, et al. Bacterial metabolism of bile acids promotes generation of peripheral regulatory T cells. Nature. 2020;581(7809):475–479. doi:10.1038/s41586-020-2193-0.
- Devlin AS, Fischbach MA. A biosynthetic pathway for a prominent class of microbiota-derived bile acids. Nat Chem Biol. 2015;11(9):685–690. doi:10.1038/nchembio.1864.
- Paik D, Yao L, Zhang Y, Bae S, D’Agostino GD, Zhang M, Kim E, Franzosa EA, Avila-Pacheco J, Bisanz JE, et al. Human gut bacteria produce Tau(Eta)17-modulating bile acid metabolites. Nature. 2022;603(7903):907–912. doi:10.1038/s41586-022-04480-z.
- Li W, Hang S, Fang Y, Bae S, Zhang Y, Zhang M, Wang G, McCurry MD, Bae M, Paik D, et al. A bacterial bile acid metabolite modulates T(reg) activity through the nuclear hormone receptor NR4A1. Cell Host Microbe. 2021;29(9):1366–1377 e1369. doi:10.1016/j.chom.2021.07.013.
- Guzior DV, Okros M, Shivel M, Armwald B, Bridges C, Fu Y, Martin C, Schilmiller AL, Miller WM, Ziegler KM, et al. Bile salt hydrolase acyltransferase activity expands bile acid diversity. Nature. 2024;626(8000):852–858. doi:10.1038/s41586-024-07017-8.
- Rimal B, Collins SL, Tanes CE, Rocha ER, Granda MA, Solanki S, Hoque NJ, Gentry EC, Koo I, Reilly ER, et al. Bile salt hydrolase catalyses formation of amine-conjugated bile acids. Nature. 2024;626(8000):859–863. doi:10.1038/s41586-023-06990-w.
- Kenny DJ, Plichta DR, Shungin D, Koppel N, Hall AB, Fu B, Vasan RS, Shaw SY, Vlamakis H, Balskus EP, et al. Cholesterol metabolism by uncultured human gut bacteria influences host cholesterol level. Cell Host Microbe. 2020;28(2):245–257 e246. doi:10.1016/j.chom.2020.05.013.
- Li C, Strazar M, Mohamed AMT, Pacheco JA, Walker RL, Lebar T, Zhao S, Lockart J, Dame A, Thurimella K, et al. Gut microbiome and metabolome profiling in Framingham heart study reveals cholesterol-metabolizing bacteria. Cell. 2024;187(8):1834–1852.e19. doi:10.1016/j.cell.2024.03.014.
- Ervin SM, Li H, Lim L, Roberts LR, Liang X, Mani S, Redinbo MR. Gut microbial beta-glucuronidases reactivate estrogens as components of the estrobolome that reactivate estrogens. J Biol Chem. 2019;294(49):18586–18599. doi:10.1074/jbc.RA119.010950.
- Sui Y, Wu J, Chen J. The role of gut microbial beta-glucuronidase in estrogen reactivation and breast cancer. Front Cell Dev Biol. 2021;9:631552. doi:10.3389/fcell.2021.631552.
- Ervin SM, Simpson JB, Gibbs ME, Creekmore BC, Lim L, Walton WG, Gharaibeh RZ, Redinbo MR. Structural insights into endobiotic reactivation by human gut microbiome-encoded sulfatases. Biochemistry. 2020;59(40):3939–3950. doi:10.1021/acs.biochem.0c00711.
- Van Eldere JR, De Pauw G, Eyssen HJ. Steroid sulfatase activity in a Peptococcus niger strain from the human intestinal microflora. Appl Environ Microbiol. 1987;53(7):1655–1660. doi:10.1128/aem.53.7.1655-1660.1987.
- Li D, Liu R, Wang M, Peng R, Fu S, Fu A, Le J, Yao Q, Yuan T, Chi H, et al. 3beta-hydroxysteroid dehydrogenase expressed by gut microbes degrades testosterone and is linked to depression in males. Cell Host Microbe. 2022;30(3):329–339 e325. doi:10.1016/j.chom.2022.01.001.
- Das UN. Essential fatty acids: biochemistry, physiology and pathology. Biotechnol J. 2006;1(4):420–439. doi:10.1002/biot.200600012.
- Huang N, Wang M, Peng J, Wei H. Role of arachidonic acid-derived eicosanoids in intestinal innate immunity. Crit Rev Food Sci Nutr. 2021;61(14):2399–2410. doi:10.1080/10408398.2020.1777932.
- Song X, Zhang H, Zhang Y, Goh B, Bao B, Mello SS, Sun X, Zheng W, Gazzaniga FS, Wu M, et al. Gut microbial fatty acid isomerization modulates intraepithelial T cells. Nature. 2023;619(7971):837–843. doi:10.1038/s41586-023-06265-4.
- Miyamoto J, Igarashi M, Watanabe K, Karaki SI, Mukouyama H, Kishino S, Li X, Ichimura A, Irie J, Sugimoto Y, et al. Gut microbiota confers host resistance to obesity by metabolizing dietary polyunsaturated fatty acids. Nat Commun. 2019;10(1):4007. doi:10.1038/s41467-019-11978-0.
- Kim M, Furuzono T, Yamakuni K, Li Y, Kim YI, Takahashi H, Ohue-Kitano R, Jheng HF, Takahashi N, Kano Y, et al. 10-oxo-12(Z)-octadecenoic acid, a linoleic acid metabolite produced by gut lactic acid bacteria, enhances energy metabolism by activation of TRPV1. FASEB J. 2017;31(11):5036–5048. doi:10.1096/fj.201700151R.
- Noguchi M, Shimizu M, Lu P, Takahashi Y, Yamauchi Y, Sato S, Kiyono H, Kishino S, Ogawa J, Nagata K, et al. Lactic acid bacteria-derived gamma-linolenic acid metabolites are PPARdelta ligands that reduce lipid accumulation in human intestinal organoids. J Biol Chem. 2022;298(11):102534. doi:10.1016/j.jbc.2022.102534.
- Foster JR, Ueno S, Chen MX, Harvey J, Dowell SJ, Irving AJ, Brown AJ. N-Palmitoylglycine and other N-acylamides activate the lipid receptor G2A/GPR132. Pharmacol Res Perspect. 2019;7(6):e00542. doi:10.1002/prp2.542.
- Kabarowski JH. G2A and LPC: regulatory functions in immunity. Prostaglandins Other Lipid Mediat. 2009;89(3–4):73–81. doi:10.1016/j.prostaglandins.2009.04.007.
- Lauffer LM, Iakoubov R, Brubaker PL. GPR119 is essential for oleoylethanolamide-induced glucagon-like peptide-1 secretion from the intestinal enteroendocrine L-cell. Diabetes. 2009;58(5):1058–1066. doi:10.2337/db08-1237.
- Peterson LW, Artis D. Intestinal epithelial cells: regulators of barrier function and immune homeostasis. Nat Rev Immunol. 2014;14(3):141–153. doi:10.1038/nri3608.
- Macia L, Tan J, Vieira AT, Leach K, Stanley D, Luong S, Maruya M, Ian McKenzie C, Hijikata A, Wong C, et al. Metabolite-sensing receptors GPR43 and GPR109A facilitate dietary fibre-induced gut homeostasis through regulation of the inflammasome. Nat Commun. 2015;6(1):6734. doi:10.1038/ncomms7734.
- Fujiwara H, Docampo MD, Riwes M, Peltier D, Toubai T, Henig I, Wu SJ, Kim S, Taylor A, Brabbs S, et al. Microbial metabolite sensor GPR43 controls severity of experimental GVHD. Nat Commun. 2018;9(1):3674. doi:10.1038/s41467-018-06048-w.
- Chang PV, Hao L, Offermanns S, Medzhitov R. The microbial metabolite butyrate regulates intestinal macrophage function via histone deacetylase inhibition. Proc Natl Acad Sci USA. 2014;111(6):2247–2252. doi:10.1073/pnas.1322269111.
- Singh N, Gurav A, Sivaprakasam S, Brady E, Padia R, Shi H, Thangaraju M, Prasad PD, Manicassamy S, Munn DH, et al. Activation of Gpr109a, receptor for niacin and the commensal metabolite butyrate, suppresses colonic inflammation and carcinogenesis. Immunity. 2014;40(1):128–139. doi:10.1016/j.immuni.2013.12.007.
- Schulthess J, Pandey S, Capitani M, Rue-Albrecht KC, Arnold I, Franchini F, Chomka A, Ilott NE, Johnston DGW, Pires E, et al. The short chain fatty acid butyrate imprints an antimicrobial program in macrophages. Immunity. 2019;50(2):432–445 e437. doi:10.1016/j.immuni.2018.12.018.
- Zhao C, Bao L, Zhao Y, Wu K, Qiu M, Feng L, Zhang N, Hu X, Fu Y. A fiber-enriched diet alleviates Staphylococcus aureus-induced mastitis by activating the HDAC3-mediated antimicrobial program in macrophages via butyrate production in mice. PLOS Pathog. 2023;19(1):e1011108.
- Antunes KH, Fachi JL, de Paula R, da Silva EF, Pral LP, Dos Santos AA, Dias GBM, Vargas JE, Puga R, Mayer FQ, et al. Microbiota-derived acetate protects against respiratory syncytial virus infection through a GPR43-type 1 interferon response. Nat Commun. 2019;10(1):3273. doi:10.1038/s41467-019-11152-6.
- Niu J, Cui M, Yang X, Li J, Yao Y, Guo Q, Lu A, Qi X, Zhou D, Zhang C, et al. Microbiota-derived acetate enhances host antiviral response via NLRP3. Nat Commun. 2023;14(1):642. doi:10.1038/s41467-023-36323-4.
- Chun E, Lavoie S, Fonseca-Pereira D, Bae S, Michaud M, Hoveyda HR, Fraser GL, Gallini Comeau CA, Glickman JN, Fuller MH, et al. Metabolite-sensing receptor Ffar2 regulates colonic group 3 innate lymphoid cells and gut immunity. Immunity. 2019;51(5):871–884 e876. doi:10.1016/j.immuni.2019.09.014.
- Sepahi A, Liu Q, Friesen L, Kim CH. Dietary fiber metabolites regulate innate lymphoid cell responses. Mucosal Immunol. 2021;14(2):317–330. doi:10.1038/s41385-020-0312-8.
- Fachi JL, Secca C, Rodrigues PB, Mato FCP, Di Luccia B, Felipe JS, Pral LP, Rungue M, Rocha VM, Sato FT, et al. Acetate coordinates neutrophil and ILC3 responses against C. difficile through FFAR2. J Exp Med. 2020;217(3). doi:10.1084/jem.20190489.
- Thio CL, Chi PY, Lai AC, Chang YJ. Regulation of type 2 innate lymphoid cell-dependent airway hyperreactivity by butyrate. J Allergy Clin Immunol. 2018;142(6):1867–1883 e1812. doi:10.1016/j.jaci.2018.02.032.
- Atarashi K, Tanoue T, Oshima K, Suda W, Nagano Y, Nishikawa H, Fukuda S, Saito T, Narushima S, Hase K, et al. Treg induction by a rationally selected mixture of clostridia strains from the human microbiota. Nature. 2013;500(7461):232–236. doi:10.1038/nature12331.
- Atarashi K, Tanoue T, Shima T, Imaoka A, Kuwahara T, Momose Y, Cheng G, Yamasaki S, Saito T, Ohba Y, et al. Induction of colonic regulatory T cells by indigenous clostridium species. Science. 2011;331(6015):337–341. doi:10.1126/science.1198469.
- Smith PM, Howitt MR, Panikov N, Michaud M, Gallini CA, Bohlooly YM, Glickman JN, Garrett WS. The microbial metabolites, short-chain fatty acids, regulate colonic Treg cell homeostasis. Science. 2013;341(6145):569–573. doi:10.1126/science.1241165.
- Furusawa Y, Obata Y, Fukuda S, Endo TA, Nakato G, Takahashi D, Nakanishi Y, Uetake C, Kato K, Kato T, et al. Commensal microbe-derived butyrate induces the differentiation of colonic regulatory T cells. Nature. 2013;504(7480):446–450. doi:10.1038/nature12721.
- Arpaia N, Campbell C, Fan X, Dikiy S, van der Veeken J, deRoos P, Liu H, Cross JR, Pfeffer K, Coffer PJ, et al. Metabolites produced by commensal bacteria promote peripheral regulatory T-cell generation. Nature. 2013;504(7480):451–455. doi:10.1038/nature12726.
- Zhu L, Li G, Liang Z, Qi T, Deng K, Yu J, Peng Y, Zheng J, Song Y, Chang X. Microbiota-assisted iron uptake promotes immune tolerance in the intestine. Nat Commun. 2023;14(1):2790. doi:10.1038/s41467-023-38444-2.
- Park J, Kim M, Kang SG, Jannasch AH, Cooper B, Patterson J, Kim CH. Short-chain fatty acids induce both effector and regulatory T cells by suppression of histone deacetylases and regulation of the mTOR-S6K pathway. Mucosal Immunol. 2015;8(1):80–93. doi:10.1038/mi.2014.44.
- Kespohl M, Vachharajani N, Luu M, Harb H, Pautz S, Wolff S, Sillner N, Walker A, Schmitt-Kopplin P, Boettger T, et al. The microbial metabolite butyrate induces expression of Th1-associated factors in CD4(+) T cells. Front Immunol. 2017;8:1036. doi:10.3389/fimmu.2017.01036.
- Sun M, Wu W, Chen L, Yang W, Huang X, Ma C, Chen F, Xiao Y, Zhao Y, Ma C, et al. Microbiota-derived short-chain fatty acids promote Th1 cell IL-10 production to maintain intestinal homeostasis. Nat Commun. 2018;9(1):3555. doi:10.1038/s41467-018-05901-2.
- Yang W, Yu T, Huang X, Bilotta AJ, Xu L, Lu Y, Sun J, Pan F, Zhou J, Zhang W, et al. Intestinal microbiota-derived short-chain fatty acids regulation of immune cell IL-22 production and gut immunity. Nat Commun. 2020;11(1):4457. doi:10.1038/s41467-020-18262-6.
- Balmer ML, Ma EH, Bantug GR, Grahlert J, Pfister S, Glatter T, Jauch A, Dimeloe S, Slack E, Dehio P, et al. Memory CD8(+) T cells require increased concentrations of acetate induced by stress for optimal function. Immunity. 2016;44(6):1312–1324. doi:10.1016/j.immuni.2016.03.016.
- Bachem A, Makhlouf C, Binger KJ, de Souza DP, Tull D, Hochheiser K, Whitney PG, Fernandez-Ruiz D, Dahling S, Kastenmuller W, et al. Microbiota-derived short-chain fatty acids promote the memory potential of antigen-activated CD8(+) T cells. Immunity. 2019;51(2):285–297 e285. doi:10.1016/j.immuni.2019.06.002.
- Trompette A, Gollwitzer ES, Pattaroni C, Lopez-Mejia IC, Riva E, Pernot J, Ubags N, Fajas L, Nicod LP, Marsland BJ. Dietary fiber confers protection against flu by shaping Ly6c(-) patrolling monocyte hematopoiesis and CD8(+) T cell metabolism. Immunity. 2018;48(5):992–1005 e1008. doi:10.1016/j.immuni.2018.04.022.
- Luu M, Weigand K, Wedi F, Breidenbend C, Leister H, Pautz S, Adhikary T, Visekruna A. Regulation of the effector function of CD8(+) T cells by gut microbiota-derived metabolite butyrate. Sci Rep. 2018;8(1):14430. doi:10.1038/s41598-018-32860-x.
- He Y, Fu L, Li Y, Wang W, Gong M, Zhang J, Dong X, Huang J, Wang Q, Mackay CR, et al. Gut microbial metabolites facilitate anticancer therapy efficacy by modulating cytotoxic CD8(+) T cell immunity. Cell Metab. 2021;33(5):988–1000 e1007. doi:10.1016/j.cmet.2021.03.002.
- Luu M, Riester Z, Baldrich A, Reichardt N, Yuille S, Busetti A, Klein M, Wempe A, Leister H, Raifer H, et al. Microbial short-chain fatty acids modulate CD8(+) T cell responses and improve adoptive immunotherapy for cancer. Nat Commun. 2021;12(1):4077. doi:10.1038/s41467-021-24331-1.
- Coutzac C, Jouniaux JM, Paci A, Schmidt J, Mallardo D, Seck A, Asvatourian V, Cassard L, Saulnier P, Lacroix L, et al. Systemic short chain fatty acids limit antitumor effect of CTLA-4 blockade in hosts with cancer. Nat Commun. 2020;11(1):2168. doi:10.1038/s41467-020-16079-x.
- Kim M, Qie Y, Park J, Kim CH. Gut microbial metabolites fuel host antibody responses. Cell Host & Microbe. 2016;20(2):202–214. doi:10.1016/j.chom.2016.07.001.
- Wu W, Sun M, Chen F, Cao AT, Liu H, Zhao Y, Huang X, Xiao Y, Yao S, Zhao Q, et al. Microbiota metabolite short-chain fatty acid acetate promotes intestinal IgA response to microbiota which is mediated by GPR43. Mucosal Immunol. 2017;10(4):946–956. doi:10.1038/mi.2016.114.
- Sanchez HN, Moroney JB, Gan H, Shen T, Im JL, Li T, Taylor JR, Zan H, Casali P. B cell-intrinsic epigenetic modulation of antibody responses by dietary fiber-derived short-chain fatty acids. Nat Commun. 2020;11(1):60. doi:10.1038/s41467-019-13603-6.
- Loscher CE, Draper E, Leavy O, Kelleher D, Mills KH, Roche HM. Conjugated linoleic acid suppresses NF-kappa B activation and IL-12 production in dendritic cells through ERK-mediated IL-10 induction. J Immunol. 2005;175(8):4990–4998. doi:10.4049/jimmunol.175.8.4990.
- Miyamoto J, Mizukure T, Park SB, Kishino S, Kimura I, Hirano K, Bergamo P, Rossi M, Suzuki T, Arita M, et al. A gut microbial metabolite of linoleic acid, 10-hydroxy-cis-12-octadecenoic acid, ameliorates intestinal epithelial barrier impairment partially via GPR40-MEK-ERK pathway. J Biol Chem. 2015;290(5):2902–2918. doi:10.1074/jbc.M114.610733.
- Yamada M, Takahashi N, Matsuda Y, Sato K, Yokoji M, Sulijaya B, Maekawa T, Ushiki T, Mikami Y, Hayatsu M, et al. A bacterial metabolite ameliorates periodontal pathogen-induced gingival epithelial barrier disruption via GPR40 signaling. Sci Rep. 2018;8(1):9008. doi:10.1038/s41598-018-27408-y.
- Ohue-Kitano R, Yasuoka Y, Goto T, Kitamura N, Park SB, Kishino S, Kimura I, Kasubuchi M, Takahashi H, Li Y, et al. alpha-linolenic acid-derived metabolites from gut lactic acid bacteria induce differentiation of anti-inflammatory M2 macrophages through G protein-coupled receptor 40. FASEB J. 2018;32(1):304–318. doi:10.1096/fj.201700273R.
- Li DK, Chaudhari SN, Lee Y, Sojoodi M, Adhikari AA, Zukerberg L, Shroff S, Barrett SC, Tanabe K, Chung RT, et al. Inhibition of microbial deconjugation of micellar bile acids protects against intestinal permeability and liver injury. Sci Adv. 2022;8(34):eabo2794. doi:10.1126/sciadv.abo2794.
- Liu TC, Kern JT, Jain U, Sonnek NM, Xiong S, Simpson KF, VanDussen KL, Winkler ES, Haritunians T, Malique A, et al. Western diet induces Paneth cell defects through microbiome alterations and farnesoid X receptor and type I interferon activation. Cell Host Microbe. 2021;29(6):988–1001 e1006. doi:10.1016/j.chom.2021.04.004.
- Vavassori P, Mencarelli A, Renga B, Distrutti E, Fiorucci S. The bile acid receptor FXR is a modulator of intestinal innate immunity. J Immunol. 2009;183(10):6251–6261. doi:10.4049/jimmunol.0803978.
- Kawamata Y, Fujii R, Hosoya M, Harada M, Yoshida H, Miwa M, Fukusumi S, Habata Y, Itoh T, Shintani Y, et al. A G protein-coupled receptor responsive to bile acids. J Biol Chem. 2003;278(11):9435–9440. doi:10.1074/jbc.M209706200.
- Guo C, Xie S, Chi Z, Zhang J, Liu Y, Zhang L, Zheng M, Zhang X, Xia D, Ke Y, et al. Bile acids control inflammation and metabolic disorder through inhibition of NLRP3 inflammasome. Immunity. 2016;45(4):802–816. doi:10.1016/j.immuni.2016.09.008.
- Hu J, Wang C, Huang X, Yi S, Pan S, Zhang Y, Yuan G, Cao Q, Ye X, Li H. Gut microbiota-mediated secondary bile acids regulate dendritic cells to attenuate autoimmune uveitis through TGR5 signaling. Cell Rep. 2021;36(12):109726. doi:10.1016/j.celrep.2021.109726.
- Hao H, Cao L, Jiang C, Che Y, Zhang S, Takahashi S, Wang G, Gonzalez FJ. Farnesoid X receptor regulation of the NLRP3 inflammasome underlies cholestasis-associated sepsis. Cell Metab. 2017;25(4):856–867 e855. doi:10.1016/j.cmet.2017.03.007.
- Arifuzzaman M, Won TH, Li TT, Yano H, Digumarthi S, Heras AF, Zhang W, Parkhurst CN, Kashyap S, Jin WB, et al. Inulin fibre promotes microbiota-derived bile acids and type 2 inflammation. Nature. 2022;611(7936):578–584. doi:10.1038/s41586-022-05380-y.
- Hang S, Paik D, Yao L, Kim E, Trinath J, Lu J, Ha S, Nelson BN, Kelly SP, Wu L, et al. Bile acid metabolites control T(H)17 and T(reg) cell differentiation. Nature. 2019;576(7785):143–148. doi:10.1038/s41586-019-1785-z.
- Song X, Sun X, Oh SF, Wu M, Zhang Y, Zheng W, Geva-Zatorsky N, Jupp R, Mathis D, Benoist C, et al. Microbial bile acid metabolites modulate gut RORgamma(+) regulatory T cell homeostasis. Nature. 2020;577(7790):410–415. doi:10.1038/s41586-019-1865-0.
- Chen ML, Huang X, Wang H, Hegner C, Liu Y, Shang J, Eliason A, Diao H, Park H, Frey B, et al. CAR directs T cell adaptation to bile acids in the small intestine. Nature. 2021;593(7857):147–151. doi:10.1038/s41586-021-03421-6.
- Gentry EC, Collins SL, Panitchpakdi M, Belda-Ferre P, Stewart AK, Carrillo Terrazas M, Lu HH, Zuffa S, Yan T, Avila-Pacheco J, et al. Reverse metabolomics for the discovery of chemical structures from humans. Nature. 2023; doi:10.1038/s41586-023-06906-8.
- Sun L, Zhang Y, Cai J, Rimal B, Rocha ER, Coleman JP, Zhang C, Nichols RG, Luo Y, Kim B, et al. Bile salt hydrolase in non-enterotoxigenic bacteroides potentiates colorectal cancer. Nat Commun. 2023;14(1):755. doi:10.1038/s41467-023-36089-9.
- Cong J, Liu P, Han Z, Ying W, Li C, Yang Y, Wang S, Yang J, Cao F, Shen J, et al. Bile acids modified by the intestinal microbiota promote colorectal cancer growth by suppressing CD8+ T cell effector functions. Immunity. 2024;57(4):876–889.e11. doi:10.1016/j.immuni.2024.02.014.
- Putera HD, Doewes RI, Shalaby MN, Ramirez-Coronel AA, Clayton ZS, Abdelbasset WK, Murtazaev SS, Jalil AT, Rahimi P, Nattagh-Eshtivani E, et al. The effect of conjugated linoleic acids on inflammation, oxidative stress, body composition and physical performance: a comprehensive review of putative molecular mechanisms. Nutr Metab (Lond). 2023;20(1):35. doi:10.1186/s12986-023-00758-9.
- Bassaganya-Riera J, Viladomiu M, Pedragosa M, De Simone C, Carbo A, Shaykhutdinov R, Jobin C, Arthur JC, Corl BA, Vogel H, et al. Probiotic bacteria produce conjugated linoleic acid locally in the gut that targets macrophage PPAR gamma to suppress colitis. PLOS ONE. 2012;7(2):e31238. doi:10.1371/journal.pone.0031238.
- How KY, Song KP, Chan KG. Porphyromonas gingivalis: an overview of Periodontopathic Pathogen below the gum line. Front Microbiol. 2016;7:53. doi:10.3389/fmicb.2016.00053.
- Bilate AM, London M, Castro TBR, Mesin L, Bortolatto J, Kongthong S, Harnagel A, Victora GD, Mucida D. T cell receptor is required for differentiation, but not maintenance, of intestinal CD4(+) intraepithelial lymphocytes. Immunity. 2020;53(5):1001–1014 e1020. doi:10.1016/j.immuni.2020.09.003.
- Bousbaine D, Fisch LI, London M, Bhagchandani P, Rezende de Castro TB, Mimee M, Olesen S, Reis BS, VanInsberghe D, Bortolatto J, et al. A conserved bacteroidetes antigen induces anti-inflammatory intestinal T lymphocytes. Science. 2022;377(6606):660–666. doi:10.1126/science.abg5645.
- Cervantes-Barragan L, Chai JN, Tianero MD, Di Luccia B, Ahern PP, Merriman J, Cortez VS, Caparon MG, Donia MS, Gilfillan S, et al. Lactobacillus reuteri induces gut intraepithelial CD4(+)CD8alphaalpha(+) T cells. Science. 2017;357(6353):806–810. doi:10.1126/science.aah5825.
- Lei X, Ketelut-Carneiro N, Shmuel-Galia L, Xu W, Wilson R, Vierbuchen T, Chen Y, Reboldi A, Kang J, Edelblum KL, et al. Epithelial HNF4A shapes the intraepithelial lymphocyte compartment via direct regulation of immune signaling molecules. J Exp Med. 2022;219(8). doi:10.1084/jem.20212563.
- Wahlstrom A, Sayin SI, Marschall HU, Backhed F. Intestinal crosstalk between bile acids and microbiota and its impact on Host metabolism. Cell Metab. 2016;24(1):41–50. doi:10.1016/j.cmet.2016.05.005.
- Dong Z, Lee BH. Bile salt hydrolases: structure and function, substrate preference, and inhibitor development. Protein Sci. 2018;27(10):1742–1754. doi:10.1002/pro.3484.
- Ridlon JM, Harris SC, Bhowmik S, Kang DJ, Hylemon PB. Consequences of bile salt biotransformations by intestinal bacteria. Gut Microbes. 2016;7(1):22–39. doi:10.1080/19490976.2015.1127483.
- Lucas LN, Barrett K, Kerby RL, Zhang Q, Cattaneo LE, Stevenson D, Rey FE, Amador-Noguez D, Manichanh C. Dominant bacterial phyla from the human gut show widespread ability to transform and conjugate bile acids. mSystems. 2021;6(4):mSystems.e0080521. doi:10.1128/mSystems.00805-21.
- Mohanty I, Mannochio-Russo H, Schweer JV, El Abiead Y, Bittremieux W, Xing S, Schmid R, Zuffa S, Vasquez F, Muti VB, et al. The underappreciated diversity of bile acid modifications. Cell. 2024;187(7):1801–1818 e1820. doi:10.1016/j.cell.2024.02.019.
- Mohanty I, Allaband C, Mannochio-Russo H, El Abiead Y, Hagey LR, Knight R, Dorrestein PC. The changing metabolic landscape of bile acids – keys to metabolism and immune regulation. Nat Rev Gastroenterol & Hepatol. 2024;21(7):493–516. doi:10.1038/s41575-024-00914-3.
- Sato Y, Atarashi K, Plichta DR, Arai Y, Sasajima S, Kearney SM, Suda W, Takeshita K, Sasaki T, Okamoto S, et al. Novel bile acid biosynthetic pathways are enriched in the microbiome of centenarians. Nature. 2021;599(7885):458–464. doi:10.1038/s41586-021-03832-5.
- Raftogianis R, Creveling C, Weinshilboum R, Weisz J. Estrogen metabolism by conjugation. J Natl Cancer Inst Monogr. 2000;2000(27):113–124. doi:10.1093/oxfordjournals.jncimonographs.a024234.
- Dabek M, McCrae SI, Stevens VJ, Duncan SH, Louis P. Distribution of beta-glucosidase and beta-glucuronidase activity and of beta-glucuronidase gene gus in human colonic bacteria. FEMS Microbiol Ecol. 2008;66(3):487–495. doi:10.1111/j.1574-6941.2008.00520.x.
- Horinouchi M, Hayashi T, Koshino H, Kurita T, Kudo T. Identification of 9,17-dioxo-1,2,3,4,10,19-hexanorandrostan-5-oic acid, 4-hydroxy-2-oxohexanoic acid, and 2-hydroxyhexa-2,4-dienoic acid and related enzymes involved in testosterone degradation in Comamonas testosteroni TA441. Appl Environ Microbiol. 2005;71(9):5275–5281. doi:10.1128/AEM.71.9.5275-5281.2005.
- Horinouchi M, Koshino H, Malon M, Hirota H, Hayashi T. Steroid degradation in Comamonas testosteroni TA441: identification of metabolites and the genes involved in the reactions necessary before D-Ring cleavage. Appl Environ Microbiol. 2018;84(22). doi:10.1128/AEM.01324-18.
- Hegyi P, Maleth J, Walters JR, Hofmann AF, Keely SJ. Guts and Gall: bile acids in regulation of intestinal epithelial function in health and disease. Physiol Rev. 2018;98(4):1983–2023. doi:10.1152/physrev.00054.2017.
- Ohnmacht C, Park JH, Cording S, Wing JB, Atarashi K, Obata Y, Gaboriau-Routhiau V, Marques R, Dulauroy S, Fedoseeva M, et al. MUCOSAL IMMUNOLOGY. The microbiota regulates type 2 immunity through RORgammat(+) T cells. Science. 2015;349(6251):989–993. doi:10.1126/science.aac4263.
- Sefik E, Geva-Zatorsky N, Oh S, Konnikova L, Zemmour D, McGuire AM, Burzyn D, Ortiz-Lopez A, Lobera M, Yang J, et al. Mucosal immunology. Individual intestinal symbionts induce a distinct population of RORgamma(+) regulatory T cells. Science. 2015;349(6251):993–997. doi:10.1126/science.aaa9420.
- Lewis WH, Tahon G, Geesink P, Sousa DZ, Ettema TJG. Innovations to culturing the uncultured microbial majority. Nat Rev Microbiol. 2021;19(4):225–240. doi:10.1038/s41579-020-00458-8.
- Huang Y, Sheth RU, Zhao S, Cohen LA, Dabaghi K, Moody T, Sun Y, Ricaurte D, Richardson M, Velez-Cortes F, et al. High-throughput microbial culturomics using automation and machine learning. Nat Biotechnol. 2023;41(10):1424–1433. doi:10.1038/s41587-023-01674-2.
- Garcia-Bayona L, Comstock LE, Lemon KP. Streamlined genetic manipulation of diverse bacteroides and parabacteroides isolates from the human gut microbiota. mBio. 2019;10(4). doi:10.1128/mBio.01762-19.
- Lim B, Zimmermann M, Barry NA, Goodman AL. Engineered regulatory systems modulate gene expression of human commensals in the gut. Cell. 2017;169(3):547–558 e515. doi:10.1016/j.cell.2017.03.045.
- Wexler AG, Goodman AL. An insider’s perspective: bacteroides as a window into the microbiome. Nat Microbiol. 2017;2:17026. doi:10.1038/nmicrobiol.2017.26.
- Mimee M, Tucker AC, Voigt CA, Lu TK. Programming a human commensal bacterium, bacteroides thetaiotaomicron, to sense and respond to stimuli in the murine gut microbiota. Cell Syst. 2015;1(1):62–71. doi:10.1016/j.cels.2015.06.001.
- Rubin BE, Diamond S, Cress BF, Crits-Christoph A, Lou YC, Borges AL, Shivram H, He C, Xu M, Zhou Z, et al. Species- and site-specific genome editing in complex bacterial communities. Nat Microbiol. 2022;7(1):34–47. doi:10.1038/s41564-021-01014-7.
- Zheng L, Tan Y, Hu Y, Shen J, Qu Z, Chen X, Ho CL, Leung EL, Zhao W, Dai L. CRISPR/Cas-based genome editing for human gut commensal bacteroides species. ACS Synth Biol. 2022;11(1):464–472. doi:10.1021/acssynbio.1c00543.
- Jin WB, Li TT, Huo D, Qu S, Li XV, Arifuzzaman M, Lima SF, Shi HQ, Wang A, Putzel GG, et al. Genetic manipulation of gut microbes enables single-gene interrogation in a complex microbiome. Cell. 2022;185(3):547–562 e522. doi:10.1016/j.cell.2021.12.035.
- Bauermeister A, Mannochio-Russo H, Costa-Lotufo LV, Jarmusch AK, Dorrestein PC. Mass spectrometry-based metabolomics in microbiome investigations. Nat Rev Microbiol. 2022;20(3):143–160. doi:10.1038/s41579-021-00621-9.
- Wang M, Carver JJ, Phelan VV, Sanchez LM, Garg N, Peng Y, Nguyen DD, Watrous J, Kapono CA, Luzzatto-Knaan T, et al. Sharing and community curation of mass spectrometry data with global natural products social molecular networking. Nat Biotechnol. 2016;34(8):828–837. doi:10.1038/nbt.3597.
- Quinn RA, Nothias LF, Vining O, Meehan M, Esquenazi E, Dorrestein PC. Molecular networking as a drug discovery, drug metabolism, and precision medicine strategy. Trends Pharmacol Sci. 2017;38(2):143–154. doi:10.1016/j.tips.2016.10.011.