ABSTRACT
Most research in nuclear mechanotransduction has focused on the nuclear lamina and lamin binding proteins. These structures provide mechanical stability to the nucleus, establish a link between the cytoskeleton and chromatin, and can transmit mechanical signals. At the same time, mechanical perturbations to the nucleus also affect its phospholipid membranes. In this commentary, we discuss how changes in nuclear membrane tension can mediate mechanotransduction.
Introduction
Mechanical forces affect virtually every tissue and organ in our body. Cells have developed numerous ways to sense and convert mechanical stimuli into biochemical signals in a process known as mechanotransduction. Physical forces applied to the cell surface distort the plasma membrane and are quickly transmitted to the cytosol. Membrane mechanotransduction has been primarily attributed to proteins embedded in, or attached to the plasma membrane, such as integrins and mechanosensitive ion channels.Citation1 The physiological consequences of mechanical stretch of intracellular membranes and organelles are less well understood. Mechanical forces applied to the cell are transmitted to the nucleus and modulate nuclear structure and gene transcription.Citation2,3 Most studied cases of nuclear mechanotransduction involve structural changes of nuclear envelope proteins or chromatin.Citation3 By contrast, whether and how nuclear membranes transduce mechanical signals is little studied and understood.
From nuclear structure to nuclear membrane tension
The nucleus can be divided into 2 parts, the nuclear envelope (NE) and the nuclear interior. The nuclear envelope is composed of an inner and outer nuclear membrane, which are continuous with each other and the endoplasmic reticulum. The two membranes join at the nuclear pore complexes (NPCs), which allow transport between the nucleus and the cytoplasm. Besides separating the genomic space from the cytoplasm, the NE provides mechanical support to the nucleus through its lamina,Citation4 a network of lamin and lamin-binding proteins underlying the inner nuclear membrane. The lamina anchors both to the inner nuclear membrane and to peripheral DNA and chromatin. The major building blocks of the nuclear lamina are A- and B-type lamins. The LINC complex (Linker of Nucleoskeleton to the Cytoskeleton) anchors elements of the cytoskeleton, the actin microfilaments, microtubules and intermediate filaments to lamins, chromatin, NPCs and other nuclear membrane proteins.Citation5,6 This linkage establishes a physical connection between the nucleoskeleton and the cell cortex through which mechanical signals can be transduced from the cell surface into the nucleus - to chromatin, and nuclear membranes ().
Figure 1. The membranes of the nuclear envelope (NE) are supported by the protein meshwork of the nuclear lamina and the cytoskeleton, which are linked via the LINC complex. Stretching forces resulting from mechanical perturbations such as nuclear swelling, are transmitted to the protein networks of the lamina and cytoskeleton, and to the nuclear membrane. Stretching the nuclear membrane increases in-plane membrane tension and loosens lipid packing, which promotes novel hydrophobic protein-lipid interactions between the inner nuclear membrane and proteins such as cPLA2 and LOX-5.
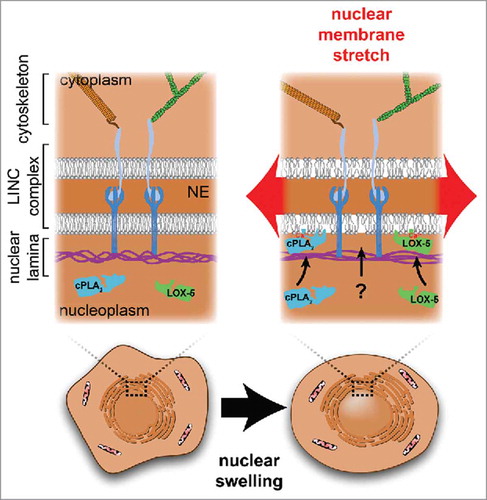
When nuclei are deformed, for example by squeezing or stretching, most of the applied force is redistributed through deformation of the nuclear lamina meshwork, which acts as a ‘molecular shock absorber’, and protects the NE from rupture.Citation7 Likely, only a small fraction of the total force is ultimately transmitted to the nuclear membranes to generate bilayer tension ().Citation8 Of note, we here define nuclear membrane tension as the in-plane tension of the lipid bilayers. This is not to be confused with the apparent NE tension. Akin to the apparent tension of the plasma membrane, the apparent NE tension comprises both the in-plane tension within the lipid bilayer and the cortical tension in the underlying structural protein network: the lamina in case of the nucleus or the actin cortex in case of the plasma membrane.Citation9 Direct physical measurements of nuclear membrane tension are not available. However, emerging literature suggests that the nuclear membranes are stretched during various pathophysiological conditions. For example, when the lamina is impaired in cancer cells or as a consequence of lamin A/C mutations,Citation10 membrane stretch can lead to transient NE rupture. Likewise, cell migration through confined channels can result in increased nuclear pressure and the formation of nuclear membrane blebs that eventually rupture,Citation11,12 implying damaging levels of tension. In addition, membrane stretch is induced by nuclear swelling.Citation13
Nuclear swelling is a direct consequence of cell swelling or lysis, which are universal hallmarks of severe homeostatic tissue perturbation and damage. When cells swell, their cytoplasmic macromolecules become diluted through extracellular water influx. When cells lyse, their cytoplasmic macromolecules leak out. In both cases, the resulting drop of extranuclear oncotic pressure forces water into the nucleus, which induces swelling and nuclear membrane stretch, if the NE remains intact. Pathological cell swelling, also termed ‘cytotoxic edema’ can have various causes. For example, following a shortage of oxygen during ischemic and hypoxic tissue injury, oxidative phosphorylation and ATP generation in mitochondria is halted. ATP is required to operate the Na+/K+ ATPase. ATP depletion leads to intracellular Na+ overload, water influx and cell swelling.Citation14 Necrotic pore formation within the plasma membrane during programmed necrosis (e.g., pyroptosis, necroptosis, etc.) results in ‘oncosis’—a lethal form of cell swelling that eventually leads to plasma membrane rupture.Citation15 More directly, cell swelling is induced by hypotonic exposure of cells.Citation16 Hypotonic cell swelling can be rapidly reversed by a physiological adaption mechanism termed ‘regulatory volume decrease’ (RVD). During RVD, cells expel cytoplasmic osmolytes to dissipate the osmotic pressure gradient over the plasma membrane.Citation17 In animals, such reversible cell swelling occurs, for instance, when the epidermis of freshwater organisms is breached, and low osmolarity fluid enters the tissue. Reminiscent of our descent from freshwater vertebrates, the mucosal linings of mouth and esophagus of land-dwelling mammals are still covered by a hypotonic liquid, known as saliva. Wounding of these mammalian epithelia results in swelling of the underlying tissue just like wounding of freshwater fish.
The thin and translucent tail fin epithelium of the freshwater fish danio rerio (zebrafish) is an excellent model to study the physiological consequences of cell swelling within an intact organism. Healing and inflammatory responses of wounded zebrafish larvae closely resemble those of higher vertebrates.Citation18 In zebrafish, cell swelling acts as a central trigger for wound closure and inflammation by recruiting epithelial cells and leukocytes to the site of injury.Citation19,20 Early leukocyte recruitment is triggered by nuclear swelling. The resulting nuclear membrane stretch recruits and activates 2 key members of the ‘eicosanoid’ cascade, cytosolic phospholipase A2 (cPLA2),Citation13,20 and 5-lipoxygenase (5-LOX),Citation13 which together produce powerful leukocyte chemoattractants.
Unlike the nucleoskeleton and its associated proteins, nuclear membranes have been little considered as mechanotransducing structures although they are, in principle, well suited for this purpose. First, nuclear membranes do not participate in constitutive membrane trafficking. Intrinsic membrane tension fluctuations caused by membrane vesicle fusion or fission are low. Therefore, nuclear membranes are likely better suited for sensing and transducing extrinsic mechanical perturbations than, for instance, the plasma membrane, or membranes of the Golgi apparatus, which are heavily engaged in vesicle trafficking. Second, nuclear membranes are buffered against excessive extrinsic force through the lamina. Owing to this structure, nuclear membranes can endure strong mechanical perturbations without rupture. Nuclei can even stay intact and retain much of their nucleoplasmic protein content long after their host cells have died and released their cytoplasm. We recently showed that these remnant nuclei, when containing cPLA2, can act as sterile inflammatory signaling beacons that attract leukocytes to cell corpses.Citation13
Biological lipid bilayers expand elastically by ∼3% before they rupture.Citation21 However, nuclear surface increases of ∼60% have been reported for isolated nuclei of Xenopus oocytes and Hela cells.Citation7,13 Apparently, swelling taps into additional surface reservoirs to expand nuclear surface without rupture. The underlying mechanisms are very little studied and understood. Nuclear and ER-lumina (cisternae) are separated, but the outer nuclear membrane and rough ER-membranes are continuous,Citation21 which enables lipid diffusion and likely also exchange of bulk membrane between compartments. ER membranes are stabilized by scaffolding proteins and are under tension.Citation21,22 This could provide resistance to membrane flows between compartments. During cell swelling, oncotic pressure gradients cause water influx both into the nucleus and the ER leading to swelling of these organelles. We envision a tug of war between nuclear and ER swelling, with each organelle pulling on the shared membrane estate thereby causing stretch (). Net-flux of membrane into the NE is likely mediated by a membrane tension gradient,Citation23 for example, caused by different osmotic pressures acting on the ER- versus nuclear membranes. Per LaPlace's law, organelle size and topology may also contribute to tension gradients: in response to the same osmotic pressure, a large compartment will develop more membrane tension than a small one, and the nucleus is the largest organelle of the cell. In addition, nuclear NPC dilatation may play a role in nuclear surface expansion: NPCs cover over 11% of the nuclear surface area in HeLa cells.Citation24 Assuming that NPCs can dilate by 30 nm Citation25 upon membrane stretching, NPC-expansion could account for ∼10% of the total ∼60% surface area expansion of swelling HeLa cell nuclei. In summary, we hypothesize that swelling-induced membrane tension, or a gradient thereof, drives net-expansion of nuclear surface through NPC dilatation or ER membrane incorporation, in parallel to activating inflammatory cPLA2 signaling.Citation13
Figure 2. Hypothetical scheme of nuclear surface reservoirs during swelling. The outer nuclear membrane is continuous with the membrane of the rough ER. The lumina of both compartments are separated, and have different size and shapes. Upon cell swelling, water influx (blue arrows) into ER cisternae and nucleoplasm may give rise to compartmental differences in osmotic pressure and membrane tension (red arrows), respectively. Tension gradients may drive bulk flow of membrane from the ER (yellow) to the nucleus (black) to expand nuclear surface. At the same time, nuclear pores may expand and thereby add to increase of nuclear surface.
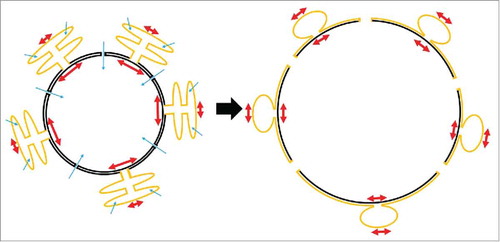
Sensing changes in nuclear membrane tension
What are the structural alterations induced by stretching of lipid bilayers, and how are these exploited for nuclear mechanosensing? Stretching loosens lipid packing of phospholipid bilayers and exposes the hydrophobic membrane core to the solvent.Citation26 This makes bilayers more susceptible to insertion of hydrophobic protein residues.Citation27 Nuclear membranes may be particularly suited for transducing tension-signals: due to their low cholesterol- and unsaturated acyl chain-content, their lipid bilayers are less densely packed, and better tuned for stretch-induced insertion of peripheral membrane proteins than the relatively rigid, tightly packed plasma membrane, which preferentially engages with proteins via electrostatic interactions.Citation28
In vitro experiments had shown that low lateral lipid pressure favors the activity of various peripheral membrane enzymes, such as isoforms of protein kinase C, phospholipase C and A2.Citation29-31 It remained unclear whether this constituted a physiologically relevant transduction mechanism. We found that nuclear membrane stretch directly stimulates membrane binding of the C2(-like) domains of cPLA2 and 5-LOX,Citation13 2 crucial enzymes of the ‘eicosanoid’ cascade. The eicosanoid cascade –one of the most powerful inflammatory mechanisms in humans– produces lipid-based paracrine mediators such as prostaglandins, leukotrienes, oxo-eicosanoids, and many more. We showed that this important cascade is physiologically activated by nuclear membrane stretch upon cell swelling or lysis, which constitutes a key inflammatory mechanism in zebrafish.Citation13,20 Our findings point to an unexpected explanation for the enigmatic nuclear localization of the eicosanoid cascade,Citation32 and assign a novel, non-genetic function to the cell nucleus: By using the eicosanoid cascade to monitor nuclear deformation, organisms can sense dangerous, homeostatic tissue perturbations (e.g., after epidermal wounding in zebrafish) and coordinate the rapid recruitment of antimicrobial cells accordingly. Interestingly, both cPLA2 and 5-LOX have functionally similar membrane binding domains. Their calcium binding loops harbor hydrophobic residues that allow them to deeply penetrate the membrane.Citation33 Calcium ions (Ca2+) neutralize acidic residues within the calcium binding loops, thus decreasing the desolvation penalty of membrane association and penetration.Citation34 By exposing hydrophobic interaction surface through membrane packing defects, nuclear membrane stretch probably promotes this interaction.
How is nuclear membrane mechanotransduction regulated in cells? Our results suggest that an intact actin cytoskeleton and an intact lamina restrict membrane binding of cPLA2, possibly through regulating nuclear morphology and membrane stretch.Citation13 For example, cytoplasmic F-actin structures such as the ‘perinuclear actin cap’, or the actin cortex may restrict nuclear swelling and stretching (). Alternatively, actin filaments may somehow strengthen the nuclear lamina. As detailed above, mechanical forces affecting the nucleus and NE are mainly transmitted to and redistributed by the nuclear lamina. Nuclei with softer nuclear lamina resist compressive forces less. Accordingly, nuclear membranes on top of a soft lamina likely receive a larger share of input force than membranes that are supported by a rigid lamina. Indeed, we observed that swelling-induced nuclear translocation and activation of cPLA2 is enhanced in lamin A/C-depleted cells Citation13 possibly because their lamina is softened. A diverse group of genetic disorders termed laminopathies are caused by mutations in the LMNA gene, which encodes lamin A and C. The precise disease mechanism for symptoms ranging from neuropathies to muscular dystrophies, lipodystrophies, and premature aging syndromes is still unknown,Citation35,36 but an inflammatory component has been linked to some of these diseases.Citation37,38 Our findings raise the question whether nuclear stretch-sensitive inflammatory pathways contribute to the pathomechanism.
The field of nuclear membrane mechanotransduction is in its beginnings, and full of unanswered questions. Given the number of peripheral membrane proteins that –like cPLA2 and 5-LOX– localize to nuclear membranes through similar domains, nuclear stretch sensing could be a broadly utilized principle of cell and tissue regulation.
Disclosure of potential conflicts of interest
No potential conflicts of interest were disclosed.
Acknowledgments
We apologize to the colleagues whose research we did not cite due to space limitations.
Funding
The work was supported by the NIH grant GM099970, and an American Asthma Foundation Scholar grant to Philipp Niethammer.
References
- Hamill OP, Martinac B. Molecular basis of mechanotransduction in living cells. Physiol Rev 2001; 81:685-740; PMID:11274342
- Maniotis AJ, Chen CS, Ingber DE. Demonstration of mechanical connections between integrins, cytoskeletal filaments, and nucleoplasm that stabilize nuclear structure. Proc Natl Acad Sci U S A 1997; 94:849-54; PMID:9023345; http://dx.doi.org/10.1073/pnas.94.3.849
- Fedorchak GR, Kaminski A, Lammerding J. Cellular mechanosensing: getting to the nucleus of it all. Prog Biophys Mol Biol 2014; 115:76-92; PMID:25008017; http://dx.doi.org/10.1016/j.pbiomolbio.2014.06.009
- Dahl KN, Ribeiro AJS, Lammerding J. Nuclear shape, mechanics, and mechanotransduction. Circ Res 2008; 102:1307-18; PMID:18535268; http://dx.doi.org/10.1161/CIRCRESAHA.108.173989
- Alam S, Lovett DB, Dickinson RB, Roux KJ, Lele TP. Nuclear forces and cell mechanosensing. Prog Mol Biol Transl Sci 2014; 126:205-15; PMID:25081619; http://dx.doi.org/10.1016/B978-0-12-394624-9.00008-7
- Jahed Z, Soheilypour M, Peyro M, Mofrad MRK. The LINC and NPC relationship - it's complicated! J Cell Sci 2016; 129:3219-29; PMID:27530973; http://dx.doi.org/10.1242/jcs.184184
- Dahl KN, Kahn SM, Wilson KL, Discher DE. The nuclear envelope lamina network has elasticity and a compressibility limit suggestive of a molecular shock absorber. J Cell Sci 2004; 117:4779-86; PMID:15331638; http://dx.doi.org/10.1242/jcs.01357
- Enyedi B, Niethammer P. A case for the nuclear membrane as a mechanotransducer. Cell Mol Bioeng 2016; 9:247-51; PMID:27453760; http://dx.doi.org/10.1007/s12195-016-0430-2
- Gauthier NC, Masters TA, Sheetz MP. Mechanical feedback between membrane tension and dynamics. Trends Cell Biol 2012; 22:527-35; PMID:22921414; http://dx.doi.org/10.1016/j.tcb.2012.07.005
- Vargas JD, Hatch EM, Anderson DJ, Hetzer MW. Transient nuclear envelope rupturing during interphase in human cancer cells. Nucleus 2012; 3:88-100; PMID:22567193; http://dx.doi.org/10.4161/nucl.18954
- Denais CM, Gilbert RM, Isermann P, McGregor AL, te Lindert M, Weigelin B, Davidson PM, Friedl P, Wolf K, Lammerding J. Nuclear envelope rupture and repair during cancer cell migration. Science 2016; 352:353-8; PMID:27013428; http://dx.doi.org/10.1126/science.aad7297
- Raab M, Gentili M, de Belly H, Thiam HR, Vargas P, Jimenez AJ, Lautenschlaeger F, Voituriez R, Lennon-Duménil AM, Manel N, et al. ESCRT III repairs nuclear envelope ruptures during cell migration to limit DNA damage and cell death. Science 2016; 352:359-62; PMID:27013426; http://dx.doi.org/10.1126/science.aad7611
- Enyedi B, Jelcic M, Niethammer P. The cell nucleus serves as a mechanotransducer of tissue damage-induced inflammation. Cell 2016; 165:1160-70; PMID:27203112; http://dx.doi.org/10.1016/j.cell.2016.04.016
- Liang D, Bhatta S, Gerzanich V, Simard JM. Cytotoxic edema: mechanisms of pathological cell swelling. Neurosurg Focus 2007; 22:E2; PMID:17613233; http://dx.doi.org/10.3171/foc.2007.22.5.3
- Vanden Berghe T, Linkermann A, Jouan-Lanhouet S, Walczak H, Vandenabeele P. Regulated necrosis: the expanding network of non-apoptotic cell death pathways. Nat Rev Mol Cell Biol 2014; 15:135-47; PMID:24452471; http://dx.doi.org/10.1038/nrm3737
- Finan JD, Chalut KJ, Wax A, Guilak F. Nonlinear osmotic properties of the cell nucleus. Ann Biomed Eng 2009; 37:477-91; PMID:19107599; http://dx.doi.org/10.1007/s10439-008-9618-5
- Hoffmann EK, Lambert IH, Pedersen SF. Physiology of cell volume regulation in vertebrates. Physiol Rev 2009; 89:193-277; PMID:19126758; http://dx.doi.org/10.1152/physrev.00037.2007
- Trede NS, Langenau DM, Traver D, Look AT, Zon LI. The use of zebrafish to understand immunity. Immunity 2004; 20:367-79; PMID:15084267; http://dx.doi.org/10.1016/S1074-7613(04)00084-6
- Gault WJ, Enyedi B, Niethammer P. Osmotic surveillance mediates rapid wound closure through nucleotide release. J Cell Biol 2014; 207:767-82; PMID:25533845; http://dx.doi.org/10.1083/jcb.201408049
- Enyedi B, Kala S, Nikolich-Zugich T, Niethammer P. Tissue damage detection by osmotic surveillance. Nat Cell Biol 2013; 15:1123-30; PMID:23934216; http://dx.doi.org/10.1038/ncb2818
- English AR, Voeltz GK. Endoplasmic reticulum structure and interconnections with other organelles. Cold Spring Harb Perspect Biol 2013; 5:a013227; PMID:23545422; http://dx.doi.org/10.1101/cshperspect.a013227
- Upadhyaya A, Sheetz MP. Tension in tubulovesicular networks of Golgi and endoplasmic reticulum membranes. Biophys J 2004; 86:2923-8; PMID:15111408; http://dx.doi.org/10.1016/S0006-3495(04)74343-X
- Dai J, Sheetz MP. Axon membrane flows from the growth cone to the cell body. Cell 1995; 83:693-701; PMID:8521486; http://dx.doi.org/10.1016/0092-8674(95)90182-5
- Mazzanti M, Bustamante JO, Oberleithner H. Electrical dimension of the nuclear envelope. Physiol Rev 2001; 81:1-19; PMID:11152752
- Solmaz SR, Blobel G, Melcák I. Ring cycle for dilating and constricting the nuclear pore. Proc Natl Acad Sci U S A 2013; 110:5858-63; PMID:23479651; http://dx.doi.org/10.1073/pnas.1302655110
- Zhang Y-L, Frangos JA, Chachisvilis M. Laurdan fluorescence senses mechanical strain in the lipid bilayer membrane. Biochem Biophys Res Commun 2006; 347:838-41; PMID:16857174; http://dx.doi.org/10.1016/j.bbrc.2006.06.152
- Janmey PA, Kinnunen PKJ. Biophysical properties of lipids and dynamic membranes. Trends Cell Biol 2006; 16:538-46; PMID:16962778; http://dx.doi.org/10.1016/j.tcb.2006.08.009
- Bigay J, Antonny B. Curvature, lipid packing, and electrostatics of membrane organelles: defining cellular territories in determining specificity. Dev Cell 2012; 23:886-95; PMID:23153485; http://dx.doi.org/10.1016/j.devcel.2012.10.009
- Boguslavsky V, Rebecchi M, Morris AJ, Jhon DY, Rhee SG, McLaughlin S. Effect of monolayer surface pressure on the activities of phosphoinositide-specific phospholipase C-beta 1, -gamma 1, and -delta 1. Biochemistry 1994; 33:3032-7; PMID:8130216; http://dx.doi.org/10.1021/bi00176a036
- Souvignet C, Pelosin JM, Daniel S, Chambaz EM, Ransac S, Verger R. Activation of protein kinase C in lipid monolayers. J Biol Chem 1991; 266:40-4; PMID:1985909
- Lehtonen JY, Kinnunen PK. Phospholipase A2 as a mechanosensor. Biophys J 1995; 68:1888-94; PMID:7612831; http://dx.doi.org/10.1016/S0006-3495(95)80366-8
- Peters-Golden M, Brock TG. Intracellular compartmentalization of leukotriene synthesis: unexpected nuclear secrets. FEBS Lett 2001; 487:323-6; PMID:11163352; http://dx.doi.org/10.1016/S0014-5793(00)02374-7
- Cho W, Stahelin RV. Membrane-protein interactions in cell signaling and membrane trafficking. Annu Rev Biophys Biomol Struct 2005; 34:119-51; PMID:15869386; http://dx.doi.org/10.1146/annurev.biophys.33.110502.133337
- Murray D, Honig B. Electrostatic control of the membrane targeting of C2 domains. Mol Cell 2002; 9:145-54; PMID:11804593; http://dx.doi.org/10.1016/S1097-2765(01)00426-9
- Schreiber KH, Kennedy BK. When lamins go bad: nuclear structure and disease. Cell 2013; 152:1365-75; PMID:23498943; http://dx.doi.org/10.1016/j.cell.2013.02.015
- Isermann P, Lammerding J. Nuclear mechanics and mechanotransduction in health and disease. Curr Biol 2013; 23:R1113-21; PMID:24355792; http://dx.doi.org/10.1016/j.cub.2013.11.009
- Komaki H, Hayashi YK, Tsuburaya R, Sugie K, Kato M, Nagai T, Imataka G, Suzuki S, Saitoh S, Asahina N, et al. Inflammatory changes in infantile-onset LMNA-associated myopathy. Neuromuscul Disord 2011; 21:563-8; PMID:21632249; http://dx.doi.org/10.1016/j.nmd.2011.04.010
- Rosengardten Y, McKenna T, Grochová D, Eriksson M. Stem cell depletion in Hutchinson-Gilford progeria syndrome. Aging Cell 2011; 10:1011-20; PMID:21902803; http://dx.doi.org/10.1111/j.1474-9726.2011.00743.x