ABSTRACT
Heterochromatin is an organizational property of eukaryotic chromosomes, characterized by extensive DNA and histone modifications, that is associated with the silencing of transposable elements and repetitive sequences. Maintaining heterochromatin is crucial for ensuring genomic integrity and stability during the cell cycle. During meiosis, heterochromatin is important for homologous chromosome synapsis, recombination, and segregation, but our understanding of meiotic heterochromatin formation and condensation is limited. In this review, we focus on the dynamics and features of heterochromatin and how it condenses during meiosis in plants. We also discuss how meiotic heterochromatin influences the interaction and recombination of homologous chromosomes during prophase I.
Introduction
Eukaryotic chromosomes are composed of DNA, RNA, and proteins which are collectively called chromatin. The basic structural component of chromatin is the nucleosome, which typically consists of two copies of each of the core histones H2A, H2B, H3, and H4 wrapped with ~146 bp of DNA [Citation1]. The core nucleosome can also be associated with the linker histone H1 which aids in nucleosome stabilization and compaction [Citation2]. Nucleosomes dynamically regulate the accessibility of the genetic information through varied chromatin states. Chromatin can be subdivided into heterochromatin and euchromatin, which were classically defined by differential staining and are now identified by sequence features, nucleosome density, histone, and DNA modifications, and transcriptional states. Euchromatin is characterized by actively transcribed genes with low density of nucleosomes, whereas heterochromatin comprises repetitive sequences and transposable elements (TEs) with highly condensed nucleosome array. Heterochromatin is transcriptionally silenced and sub-classified into two types: facultative heterochromatin and constitutive heterochromatin. The facultative type is defined as regions that are condensed and transcriptionally silent, but can become ‘open’ and transcriptionally active in specific circumstances, such as mammalian X chromosomes during embryo development [Citation3]. Most heterochromatin is constitutive and remains silenced, including pericentromeric regions and telomeres [Citation4,Citation5]. The establishment of heterochromatin restrains the activity of TEs, isolates damaged repetitive DNA, and ensures proper chromosome segregation, which are essential functions for maintaining genomic stability in both mitosis and meiosis [Citation3,Citation6].
Meiosis is essential for sexual reproduction in eukaryotes. In meiosis, germ cells divide twice after one round of DNA replication, producing haploid gametes. Meiotic chromatin and nuclear organization differ dramatically from somatic or mitotic cells, with specific properties and dynamics [Citation7–9]. During mitosis, chromatin fibers rapidly coil into discrete rod-shaped chromosomes from prophase to metaphase [Citation9]. In contrast, the process of chromosome compaction during meiosis prophase I is a long-duration event, with homologous chromosomes interacting and condensing from thin thread-like structures (leptotene) to short rod-shaped bivalents (metaphase I) [Citation10]. During this period, the core events of meiosis, including pairing, synapsis, and recombination between homologous chromosomes, are essential for the exchange of genetic information and segregation of homologs [Citation11]. The properties and dynamics of highly condensed meiotic heterochromatin are important for meiotic homologous recombination and chromosome segregation, but the specific mechanisms of heterochromatin condensation during meiosis are not well understood. In this review, we will use examples from plants supplemented with relevant studies from other model organisms to discuss the features of meiotic heterochromatin with special attention paid to condensation, and summarize how heterochromatin influences meiotic recombination and genome stability.
The features and epigenetics of pericentromeric heterochromatin
Constitutive heterochromatin is mainly present in pericentromeric regions, centromeres, and telomeres [Citation4,Citation5]. The organization of pericentromeric heterochromatin in multicellular eukaryotes appears to use shared principles [Citation3], but many mechanistic details remain elusive, especially in plants. Heterochromatin and euchromatin are distinguished by epigenetic features, including DNA methylation, histone modifications, and the presence of histone variants (). In most eukaryotes, DNA in pericentromeric heterochromatin is densely methylated (), which contributes to the heterochromatin structure and silencing of transposons and other repeats [Citation3,Citation12]. In plants, the majority of DNA methylation occurs in the repeat-rich pericentromeric regions where cytosines are methylated in three sequence contexts: CG, CHG, and CHH (H=A, T or C) [Citation12,Citation13]. CG methylation is catalyzed by DNA Methyltransferase 1 (MET1), which interacts with PCNA (proliferating cell nuclear antigen) and methylates the hemi-methylated CG dinucleotides during DNA replication in a process known as maintenance DNA methylation [Citation14,Citation15]. CHG methylation is mainly catalyzed by methyltransferase Chromomethylase 3 (CMT3), which positively correlates with H3K9me2 [Citation12,Citation16]. CHH methylation is generated by the Domains Rearranged Methylase 2 (DRM2) methyltransferase and regulated by the RNA-directed DNA methylation (RdDM) pathway [Citation12,Citation17]. CHH methylation is also promoted by Decreased DNA Methylation 1 (DDM1) and CMT2 at histone H1-containing heterochromatin independent of RdDM [Citation18].
Figure 1. Structure and characteristics of chromosomes in arabidopsis. (a) The model of chromosome, which consists of centromere (purple), pericentromeric heterochromatin (red), telomere (orange) and euchromatin (blue). Pericentromeric heterochromatin and euchromatin are classified by sequence features, transcriptional states, epigenetic modifications and nucleosome density. The euchromatin is enriched with actively transcribed genes that possess loosely organized H3.3-nucleosomes marked by H3K4me3. The heterochromatin is composed of repetitive sequences and transposons with highly methylated DNA and condensed H3.1- or H2A.W-nucleosomes modified by H3K9me2 and H3K27me1. (b) Immunostaining of heterochromatic markers (5-mC, H3.1 and H3K27me1) at pachytene of meiosis, mitotic prophase and interphase of somatic cells of arabidopsis. The 5-mC, H3.1, and H3K27me1 signals show co-localization with darkly staining pericentromeric regions. SYN1/REC8 is meiosis-specific cohesion labeling chromatin. Scale bars: 5 μm.
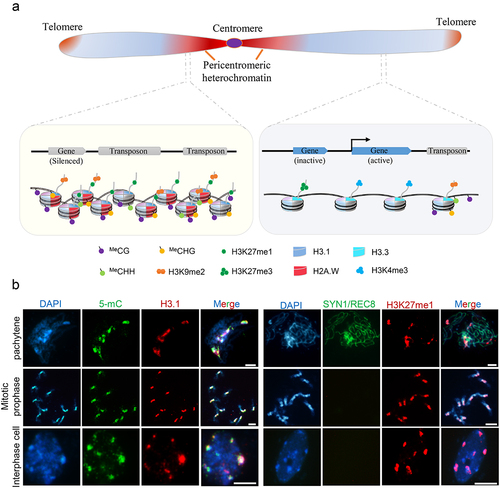
Heterochromatin is characterized by specific histone variants and modifications. In plants, the two main histone H3 variants are H3.1 and H3.3 [Citation19]. Histone variant H3.1 is incorporated into newly synthesized chromatin through the Chromatin Assembly Factor-1 (CAF1) complex in a DNA replication-dependent manner [Citation20,Citation21]. Histone variant H3.3 deposition correlates with active euchromatic genes by replacing H3.1, resulting in the enrichment of H3.1 in heterochromatin [Citation22–24]. Deficiency of CAF1 leads to impaired enrichment of H3.1, derepression of transposable elements (TEs), and pericentromeric heterochromatin deficiency in plants [Citation20,Citation25]. The plant-specific H2A variant H2A.W also promotes heterochromatin formation [Citation26], and linker histone H1 mediates heterochromatin condensation and TEs silencing [Citation27]. Interestingly, H3.1, H2A.W, and H1 are all associated with DNA methylation pathways that maintain the suppression of TEs [Citation27,Citation28].
The histone modifications H3K9me1/2 and H3K27me1 are usually present in the tails of H3.1 and co-occur with H2A.W in heterochromatic regions [Citation21,Citation24,Citation26,Citation29]. In Arabidopsis, the histone methyltransferases SU(VAR)3–9 HOMOLOG 4 (SUVH4), SUVH5, and SUVH6 mediate H3K9me1/2 modification, which primarily occurs in pericentromeric heterochromatin [Citation30,Citation31]. H3K9me2 and CHG DNA methylation form a reinforcing loop [Citation32]. From S. pombe to mammals, heterochromatin protein 1 (HP1) is an evolutionarily conserved protein that serves as a hub for heterochromatin formation [Citation33,Citation34]. HP1 localizes at pericentromeres by recognizing methylated H3 lysine 9 (H3K9me) and recruits histone modifiers, RNAi components, chromatin remodelers, and cohesins to facilitate heterochromatin condensation [Citation35,Citation36]. HP1 can also promote heterochromatin compaction via phase separation [Citation34]. In plants, Like Heterochromatin Protein 1 (LHP1), an ortholog of HP1, recognizes H3K27me3 (H3 lysine 27 trimethylation) on euchromatin and is required for transcriptional regulation [Citation37,Citation38], and a multivalent H3K9me reader ADCP1 has been found to play a role in heterochromatin formation and phase separation by modulating H3K9me and DNA methylation [Citation39,Citation40]. Polycomb Repressive Complex 2 (PRC2) has conserved functions in animals and plants in catalyzing H3K27me [Citation41,Citation42]. In plants, H3K27me1 is preferentially located in constitutive heterochromatin, while H3K27me3 is mainly restricted to gene-rich euchromatin () [Citation43,Citation44]. Intriguingly, plant PRC2 is primarily responsible for H3K27me2/3, while more recently evolved Arabidopsis Trithorax-Related proteins 5 and 6 (ATXR5 and 6) catalyze H3K27me1 of H3.1 preferentially on heterochromatin [Citation29,Citation45]. ATXR5 and 6 are essential for chromatin condensation, genomic stability and transcriptional repression within pericentromeric heterochromatin [Citation46–48].
Other factors also contribute to heterochromatin formation. For example, two members of the conserved Microrchidia (MORC) adenosine triphosphatase (ATPase) protein family, MORC1 and MORC6, form a hetero-dimer that represses TE expression and promotes heterochromatin condensation in Arabidopsis [Citation49]. The dimer is proposed to interact with RdDM components and the SWI/SNF chromatin-remodeling complex to mediate TE silencing and chromatin condensation [Citation50,Citation51]. Additionally, Arabidopsis MORC4 and MORC7 can form homodimers that have redundant roles in repressing gene expression and establishing RdDM [Citation52,Citation53]. In addition to their classical functions in DNA replication, DNA replication factors also contribute to the formation and inheritance of heterochromatin [Citation54]. PCNA not only plays a role in CG DNA methylation maintenance but also is required for H3.1 deposition and H3K27me1 within heterochromatin during DNA replication [Citation12,Citation55,Citation56]. In S. pombe, DNA polymerase epsilon (POL ε) collaborates with RNAi machinery and histone methyltransferase of H3K9me2 to recruit Swi6 (the fission yeast HP1 orthologue), thereby promoting heterochromatin assembly [Citation57,Citation58]. In S. cerevisiae, POL ε is required for the inheritance of silenced telomeres and rDNA after replication [Citation59,Citation60]. Similarly, Arabidopsis POL ε also functions in heterochromatin condensation and TE silencing in somatic cells, and POL ε can prevent CHG DNA hypermethylation of heterochromatin thereby safeguarding DNA replication [Citation61].
Heterochromatin condensation in meiosis
In mitosis, chromatin rapidly condenses into rod-shaped chromosomes from prophase to metaphase, whereas meiotic chromatin undergoes relatively slow condensation from thread-like structures to short rod-shaped bivalents during prophase I, which is divided into 5 stages () [Citation9,Citation62]. In early meiotic prophase, homologs, comprised of two sister chromatids, are organized as co-oriented linear arrays DNA-loops, that are highly packed and joined by a proteinaceous structure known as the chromosome axis [Citation7,Citation63]. The structural organization of the mitotic prophase axis is directly comparable to that observed in meiosis. The axes in both processes consist of a complex structural component, including cohesins, condensins, and topoisomerases [Citation7,Citation63]. Meiotic chromosome axes contain meiotic specific HORMA domain proteins, such as ASY1, ASY3 and ASY3 in Arabidopsis [Citation64–66], which are important for early chromosome architecture and homolog synapsis during prophase I [Citation7,Citation11]. Cohesin and condensin are related complexes that consist of SMC (Structural Maintenance of Chromosomes) proteins and are essential for chromatin axis formation and condensation during cell division [Citation67–70]. Some subunits of cohesin and condensin are used in both meiosis and mitosis, but other subunits are specifically involved in meiotic condensation and chromosome segregation, including the meiosis-specific cohesin subunit SYN1/REC8 [Citation68,Citation71,Citation72]. The condensation of meiotic chromosomes in meiosis I is characterized by two distinct processes. The first process occurs during early prophase I, where chromosomes perform elongated structures mainly organized around the central axis composed of cohesins. The second process resembles mitotic-like longitudinal compaction and takes place after recombination, as meiotic cells prepare for division. This compaction primarily relies on condensins. Moreover, cohesin has different localization patterns and functions in meiosis I and II. In meiosis I, cohesin holds sister chromatids together and localizes along the whole chromosome as part of the chromosome axes and has been proposed to regulate chromosome loop size, which is required for homolog pairing and synapsis [Citation7,Citation72]. Prior to anaphase I cohesin is released from the chromosome arms to facilitate segregation of homologs, but is retained near centromeres to keep the sister chromatids paired until anaphase II [Citation67,Citation73,Citation74].
Figure 2. Chromosome morphology during meiotic prophase I. (a) Diagrams showing the process of chromosome condensation from leptonemato metaphase I. (b) The DAPI (dark) and centromere (red) signals are shown from leptonema to metaphase I of Arabidopsis by chromosome spread. Centromeres are signed via FISH assay using 180-bp repeat DNA probes. Pericentromeric heterochromatin is visualized as dark-staining chromatin adjacent to centromeres. Scale bars: 5 μm.
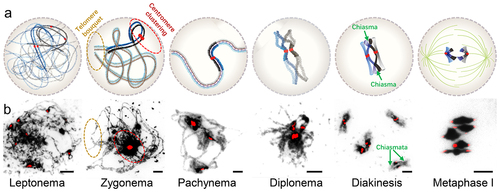
Normal heterochromatin architecture with centromeric cohesion is required for faithful chromosome segregation during both mitosis and meiosis [Citation62,Citation67,Citation75]. In Arabidopsis, SCC2 (Sister Chromatid Cohesion protein 2) is indispensable for loading cohesin during both mitosis and meiosis. Consequently, scc2 null alleles result in embryonic lethality, while weak alleles of scc2 exhibit meiotic defects in meiosis-specific cohesion loading, synapsis, recombination, and chromosome condensation [Citation76,Citation77]. Meiosis-specific cohesin subunit SYN1/REC8 is conserved in a wide range of eukaryotes and is required for chromosome condensation, segregation, and DSB repair [Citation75,Citation78]. The REC8-cohesin complex exhibits significant accumulation in the pericentromeric heterochromatin of meiosis in yeast and plants [Citation72,Citation79,Citation80]. In S. pombe, Swi6 binds H3K9me to recruit meiosis-specific cohesin complexes, further demonstrating the importance of cohesin for meiotic heterochromatin architecture [Citation81]. In addition, Arabidopsis Male Meiocyte Death 1 (MMD1), a PHD-finger protein, specifically regulates meiotic chromatin condensation, including heterochromatin, by interacting with the histone demethylase JMJ16 to promote the expression of condensin CAP-D3 [Citation82–84].
Similar epigenetic features are associated with heterochromatin have been detected using immunofluorescence in both meiosis and mitosis, including DNA methylation, histone modifications, and histone variants () [Citation85,Citation86]. A recent study reveals that the catalytic subunit of POL ε (POL2A) recognizes histone H3.1-H4 and recruits ATPase MORC1-MORC6 to function in meiotic heterochromatin formation and condensation in Arabidopsis [Citation86]. POL2A selectively recognizes histone variant H3.1-H4 via a ZF1 (Zinc finger 1) domain in its C terminus and probably helps mediate H3.1-H4 deposition with CAF1 during replication [Citation86]. Subsequently, POL2A recruits MORC1-MORC6 via its N terminus to function in heterochromatin condensation during meiosis [Citation86]. Although POL2A is also involved in heterochromatin condensation in somatic cells, the mechanism may differ from that in meiotic cells [Citation61]. In somatic cells, POL2A is essential for heterochromatin organization and TE silencing by maintaining proper DNA replication [Citation61]. Heterochromatin condensation and silencing are compromised in three pol2a mutants with mutations in its DNA polymerase domain. These condensation defects are accompanied by compensatory CHG hyper-methylation, while exhibiting minimal effects on H3K27me1, H2A.W, and siRNA [Citation61]. By comparison, mutations in N terminus of POL2A result in decondensed structure, decreased nucleosome density and H3K27me1 modification of meiotic heterochromatin [Citation86]. Interestingly, the N terminus of POL2A interacts not only with MORC1 but also SU(VAR)3–9 homologs SUVH2 and SUVH9 (two RdDM components), and POL2A does not affect TE silencing but is required for CHH methylation in meiosis [Citation87]. These findings suggest that POL2A plays a crucial role in the organization of heterochromatin in both meiotic and somatic cells in plants, but the mechanisms regulated by POL2A may differ from each other [Citation25,Citation49,Citation86]. MORC1 was initially identified in mice and its mutation causes arrest of spermatogenesis in early prophase I [Citation88]. Further study showed that MORC1 is responsible for TEs repression and DNA methylation in the mouse male germline [Citation89]. In the C. elegans germline, CeMORC1 plays an important role in heterochromatin silencing and siRNA-dependent chromatin condensation by preventing the invasion of H3K36me3 into heterochromatin [Citation90]. Deficiency of CeMORC1 and nuclear RNAi leads to decondensation of the germline chromatin and germline mortality [Citation90]. These results indicate the important role of MORC proteins in heterochromatin condensation during germline development. These examples show that meiosis and mitosis share several epigenetic and genetic mechanisms to regulate heterochromatin condensation, albeit with some specific factors in meiosis.
Meiotic recombination rarely occurs within heterochromatin
Recombination, as a key event during meiosis, is initiated by the formation of double-strand breaks (DSBs) generated by an evolutionarily conserved DNA topoisomerase-VI-like complex including SPO11 (SPORULATION 11) [Citation91]. After excising short DNA segments from one end of the break to generate 3’-OH single-stranded DNA, this DNA can invade its allelic partner on one homolog chromatid, forming a D-loop. Subsequently, a few recombination intermediates through DNA synthesis templated from the homologous chromosome and ligation are designated to become crossovers (COs). The remaining majority of intermediates are destined to mature without the exchange of flanking regions, referred to as noncrossovers (NCO) [Citation10,Citation11,Citation92]. Recombination ensures the proper segregation of homologs after metaphase I by facilitating homolog pairing and producing COs to link them during prophase I () [Citation11]. Recombination also generates new allelic combinations and phenotypic diversity among gametes and progeny.
COs are not randomly distributed, but instead occur preferentially in regions known as recombination hotspots [Citation93]. Chromosome organization has a significant influence on the formation and distribution of DSBs and COs, which predominantly occur in euchromatin regions that are enriched with H3K4me3 and H2A.Z, have low nucleosome density (LND) and low DNA methylation [Citation93–103]. H3K4me3 methyltransferases and readers of their activity play a crucial role in determining the localization of DSBs in euchromatin in yeast and animal [Citation104,Citation105]. In mouse and human, a SET domain protein with the zinc finger PRDM9 recognizes the specific DNA motifs and catalyzes H3K4me3 to initiate the DSB formation [Citation106,Citation107]. Although PRDM9 subfamily is lost in plants, in Arabidopsis, DSBs tend to occur within promoter or terminator regions of euchromatin genes that are associated with a series of chromatin marks that promote RNA polymerase II transcription, such as proximity to H3K4me3, with hypomethylated DNA and LND [Citation93,Citation108]. Furthermore, Arabidopsis DSB hotspots exhibit a significant association with certain transposable elements, namely the Helitron, Pogo/Tc1/Mariner and MuDR families that are enriched in proximal regions of the chromosome arms. Conversely, RNA transposons including LTR (long terminal repeat), Copia LTR and LINE-1 are rarely found to overlap with DSBs and tend to be enriched in proximity to the centromeres. These observations indicate that these features may contribute to the chromatin reorganization in euchromatin regions, which is required for the DSB formation. In contrast, due to the repression of DSBs formation in heterochromatic regions with contrasting characteristics, crossover events (COs) are typically suppressed in heterochromatic regions, particularly in the pericentromeric regions [Citation109,Citation110]. This is thought to be due to the inaccessibility of highly condensed heterochromatin and selective pressures against the negative consequences of COs within repetitive sequences that pose a risk to chromosome segregation and genome integrity [Citation111]. The occurrence of COs in pericentromeric or centromeric regions can disrupt cohesin loading and prevent the proper association between kinetochores and spindles during segregation [Citation111,Citation112]. For instance, mis-segregation of chromosomes due to COs near the centromere is correlated with birth defects in human [Citation113]. Pericentromeric COs are associated with premature separation of sister chromatids and lead to sporulation defects in S. cerevisiae [Citation114]. Genome-wide sequencing has enabled high-resolution maps of meiotic recombination in Arabidopsis [Citation95,Citation97,Citation102,Citation109], rice [Citation115,Citation116], tomato [Citation117,Citation118], wheat [Citation119–121], maize [Citation122,Citation123], barley [Citation124] and soybean [Citation125,Citation126], confirming that centromeric and pericentromeric CO suppression is a common feature of plant species.
In addition to the physical exclusion of recombination proteins by highly condensed heterochromatin, epigenetic modifications also play important roles in regulating COs near plant centromeres. DNA methylation commonly functions in gene silencing and correlates with heterochromatin formation [Citation12]. In plants, MET1 is the methyltransferase for CG DNA methylation, which represents the predominant methylation pattern in plants [Citation12]. In met1, the total number of COs is not altered, but they are redistributed along the chromosomes. The number of COs decreases in pericentromeric heterochromatin and increases on euchromatin and centromere-proximal regions [Citation97]. Furthermore, several investigations show that loss of DNA methylation leads to redistribution of meiotic recombination along chromosomes with increased rates in chromosome arms and reinforced or maintained suppression within pericentromeric regions [Citation98,Citation99,Citation127]. These results are surprising given prior hypotheses that DNA methylation is a suppressor of recombination within the heterochromatin near centromeres. Another study provides a possible explanation by showing that while MET1 influences CG DNA methylation, recombination is also suppressed by RdDM (RNA directed DNA methylation) inducing non-CG (CHH and CHG) DNA methylation, H3K9me2, or increased nucleosome occupancy [Citation95]. Consistent with this observation, both CG and non-CG DNA methylation repress meiotic DSBs formation, whereas non-CG methylation and/or H3K9me2 directly inhibit COs in pericentromeric regions [Citation94,Citation108]. Similarly, the RNAi pathway and H3K9 methyltransferase Clr4-Rik1 function in suppressing meiotic recombination within centromeric and pericentromeric heterochromatin in S. pombe [Citation128]. Swi6 can recruit meiosis-specific cohesin by recognizing H3K9me, thereby repressing DSB formation in meiotic pericentromeric heterochromatin [Citation81]. Furthermore, in mice, the dnmt3l (required for de novo DNA methylation) and miwi2 (functions in small RNA-dependent TE silencing) mutants exhibit loss of DNA methylation and H3K9me2, as well as an increase in deleterious DSBs and recombination within retrotransposons, resulting in meiotic arrest [Citation129]. Therefore, repression of DSBs formation likely makes a significant contribution to the inhibition of recombination; however, other factors such as specific histone and DNA modifications play a role in the maturation of COs.
In some species, CO frequency increases from the centromere toward the telomeres, and is high close to the telomeres [Citation121,Citation124,Citation130–134]. In S. cerevisiae, the telomeres and centromeres are both identified as ‘DSB cold’ regions [Citation135], and the recombination frequency in subtelomeric regions is much lower than in the adjacent unique gene-rich regions or the genome-average frequency [Citation136–138]. It has been suggested that a change in the homeostatic balance between CO and NCO with a switch from interhomolog to intersister repair in telomeres is responsible for this pattern [Citation138]. In maize, hexaploid wheat and barley, the distribution of COs exhibits a gradual decline from telomere to centromere, with a notable concentration in the telomeric-near regions [Citation133]. In contrast, CO frequencies show a slight increase from telomere to centromere in Arabidopsis [Citation102,Citation139]. Sex-specific patterns are also observed near telomeres in some species; for example, elevated CO frequencies have been observed near telomeres in human and mouse males [Citation140]. In Arabidopsis, female CO rates are lower in telomeric regions and higher in proximal regions; however, male COs remain high in distal regions including telomeres [Citation132]. The distribution of meiotic recombination is probably correlated with the structural and functional features along the chromosomes, such as GC content, gene density, gene types, transcription, and chromosome modifications, but these data do not reflect a general pattern for all species [Citation108,Citation121,Citation124,Citation134,Citation141].
Centromere coupling during meiosis
Centromeric DNA ranges from discrete motifs of a few hundred base-pairs, as seen in some fungal species such as S. cerevisiae, to megabase-sized arrays of satellite repeats and retrotransposons typical in most multicellular eukaryotes [Citation109,Citation142]. Centromeres function in orchestrating the assembly of the kinetochore complex, facilitating precise attachment of the kinetochore to the spindle and ensuring accurate chromosome segregation [Citation142,Citation143]. Pairing of homologous chromosomes is critical for meiotic recombination. In S. cerevisiae and most plants, centromeres cluster during early prophase I of meiosis [Citation144–150]. Centromeres of non-homologous chromosomes are associated with the leptotene–zygotene transition stage. Subsequently, centromeres pair which aids in the synapsis of homologous chromosomes from zygotene to pachytene [Citation148–151]. During this process, recombination-independent non-homologous centromere clustering draws the meiotic chromosomes into close proximity, promoting recognition and pairing of homologous chromosomes () [Citation148].
Heterochromatin, cohesin, and the synaptonemal complex (SC) help promote centromere associations during meiotic prophase I. In mouse, centromeres do not appear to cluster in early prophase I, but pair in late prophase I [Citation152,Citation153]. However the meiosis-specific cohesin component STAG3 is important for the formation of pericentromeric heterochromatin clustering at leptotene and zygotene [Citation154]. Furthermore, the SC is required for the homologous pericentromeric heterochromatin pairing at pachytene, which further mediates homologous centromere connections from heterologous centromere clusters [Citation144,Citation153]. Interestingly, following the dissolution of SC-induced centromere pairing during diplotene, the pericentromeric heterochromatin with centromeres of the homologs remains persistently associated, thereby maintaining connections between homologous chromosomes even without crossover tethering [Citation144,Citation153]. Therefore, SC plays a role in the formation but not maintenance of homologous heterochromatin/centromere connections. However, it is still unknown what maintains heterochromatin connections with centromeres between homologs without SC. In S. cerevisiae, the central SC element Zip1 and Rec8 play an important role in the interaction between nonhomologous centromeres independently of Spo11 [Citation151,Citation155,Citation156], and the zip1 mutants show increased recombination rate in pericentromeric regions [Citation138].
In plants, ZYP1 (the ortholog of yeast Zip1) does not participate in meiotic centromere coupling, whereas REC8 is still required [Citation157,Citation158]. In maize, centromere clustering is independent of ZYP1, but REC8 and the SC component Structural Maintenance of Chromosome 6 (SMC6) have effects on centromere pairing [Citation158]. Furthermore, the transition from non-homologous centromere clustering to homologous centromere pairing seems to rely on the initiation of meiotic recombination by SPO11 [Citation145,Citation148,Citation155]. In Arabidopsis, homologous centromere pairing partially depends on the meiosis-specific recombinase DMC1 and SPO11, while RAD51 is required for subsequent pairing and synapsis of chromosome arms [Citation145].
Non-homologous centromere clustering leads to homologous pairing and bivalent formation after pachytene [Citation86,Citation148,Citation149]. Intriguingly, deficiency of POL2A or MORC1 not only compromises meiotic heterochromatin condensation, but also leads to defects in disassembly of non-homologous centromere clustering after pachytene [Citation86]. In pol2a-1 and morc1, meiotic chromosomes show centromere coupling at late leptotene, but aberrantly maintain centromere clustering with heterochromatin association from zygotene to diakinesis, which may lead to meiotic genome instability [Citation86]. In polyploid wheat, the Ph1 (pairing homoeologous 1) locus plays an important role in the switch from centromere clustering to homologous pairing upon entry into meiosis [Citation62,Citation159,Citation160]. Ph1 functions in homologous pairing by regulating the remodeling of heterochromatic regions. In absence of Ph1, heterochromatin assumes a more open state, which leads to the abnormal association of repetitive regions on homoeologous chromosomes, which can lead to ectopic recombination [Citation62,Citation161]. The mechanisms that facilitate clustering and separation of meiotic centromeres with pericentromeric heterochromatin in prophase I remain to be fully characterized.
Telomere bouquet in meiosis
Telomeres are composed of repeated sequences and function to protect the ends of chromosomes from degradation and loss due to the end replication problem [Citation162]. Telomeres also participate in chromosome organization, pairing, synapses, and movement during meiotic prophase [Citation163,Citation164]. In many organisms, the telomeres cluster together to form a bouquet that facilitates homologous interactions in early prophase I of meiosis. Telomere bouquets are required for homologous pairing, synapsis, and recombination [Citation165,Citation166]. Surprisingly, telomere bouquets also play a role in centromere assembly and spindle attachment that promotes proper chromosome segregation in S. pombe [Citation167], but similar phenotype is not observed in other organisms.
In many fungi and animals, telomeres cluster on the nuclear envelope (NE) and form a bouquet microtubule organizing center (MTOC) in meiotic prophase I, which is regulated by several telomere-binding proteins, including the Sad1/UNC-84 (SUN) homology and Klarsicht/ANC-1/Syne (KASH) homology (SUN/KASH) complexes [Citation166]. In S. pombe, a SUN domain protein Sad1 and a putative KASH domain protein Kms1 form a transmembrane complex linking telomeres to microtubules and cytoplasmic dynein for telomere clustering [Citation168]. Bouquet1 and − 2 forming dimers function as a bridge to link Sad1 with telomere protein Rap1, thereby tethering telomeres to the spindle pole body (SPB, the MTOC in yeast) [Citation168,Citation169]. Additionally, Bouquet3 and − 4 also target telomeres to the SPB to promote bouquet formation [Citation170]. In mammals, telomere-bound SUN/KASH complexes with dynein and microtubules to link the nucleoskeleton and cytoskeleton thereby facilitating the movement of telomeres on the NE [Citation171–173]. SUN1 and SUN2 are components of the linker of nucleoplasm and cytoplasm (LINC) complexes and function, with partial redundancy, in recruiting telomeres to the NE and promoting bouquet formation in meiosis [Citation167,Citation173–175]. The meiosis-specific KASH protein, KASH5, interacts with SUN1 and regulates localization of telomeres in a SUN1-dependent manner [Citation172,Citation176]. Deficiency of SUN1 or KASH5 prevents telomere association with NE, leading to impaired homolog synapsis and sterility [Citation172,Citation173]. Recent reports found that the transmembrane protein KASH5 functions as a dynein activating adaptor in driving telomere dynamics in meiotic prophase I, which binds and converts dynein into a processive motor [Citation177,Citation178]. In summary, the LINC complexes, consisting of SUN on inner nuclear membrane and KASH on outer nuclear membrane, play a crucial role in connecting the nucleoplasm and cytoplasm across the NE, thus facilitating the transmission of force from the cytoskeleton to the nuclei. The force is finally transduced onto telomeres via telomere-binding proteins and promotes the movement of telomeres along the NE to transiently cluster in a ‘bouquet’ that brings homologs into close proximity for homologous pairing, synapsis and recombination [Citation166,Citation172,Citation175–178].
In plants, telomere-led movements are also conserved and function in facilitating homolog pairing and recombination [Citation179]. In Arabidopsis, the homologous telomeres pair in early prophase and move to the nuclear membrane to promote synapsis (). However, telomere clustering is very loose and forms a transitory bouquet because of the absence of a MTOC [Citation163,Citation180]. In Arabidopsis and rice, SUN1 and SUN2 (SUN domain proteins) function in telomere bouquet formation independent of DSB formation and affect homologous pairing and synapsis [Citation181,Citation182]. In wheat, subtelomeric regions participate in homolog recognition and pairing [Citation183]. In barley, both chromosome axes and synapsis initiate at the telomeric regions, which is required for synapsis and recombination of homologs [Citation184]. In maize, PAM1 (plural abnormalities of meiosis 1) was identified to function in telomere clustering and movement on NE, and deficiency of PAM1 leads to impaired homologous synapsis and delayed DSB repair [Citation185]. However, the biochemical function and mechanism of PAM1 in telomere clustering are still elusive. Further investigation is required to understand the formation of telomere bouquets and related proteins in plants.
Discussion and perspectives
An important challenge in studying meiotic heterochromatin in plants is that epigenomic profiling of meiocytes is relatively difficult compared to somatic or mitotic cells. Plant meiocytes are typically low in abundance, buried within somatic tissue, and are often found as a heterogeneous mixture of stages. In recent years, advances in germ cell isolation by microdissection [Citation87,Citation186–188] or flow cytometry [Citation189,Citation190] and low‐input epigenomic sequencing techniques [Citation191,Citation192] have enabled the analysis of the DNA methylation landscape, small RNA and transcription dynamics in germline cells, including meiocytes of plants [Citation87,Citation190,Citation193–196]. In animals, epigenetic reprograming occurs during meiosis, including DNA methylation and histone modifications in heterochromatin [Citation197,Citation198]. As mentioned above, CG, CHG and CHH methylation are the three main types of DNA methylation in plants, which are enriched in heterochromatin. Interestingly, in Arabidopsis, reprograming of DNA methylation in the male germline is driven by tapetal 24‐nt siRNAs [Citation189]. Furthermore, it is observed that CHH methylation levels are relatively low in heterochromatin regions compared to somatic cells, while CG or CHG methylation largely remains unchanged [Citation87,Citation190]. However, the mechanism for establishing CHH methylation and the effects of the CHH reprograming on meiotic heterochromatin are still elusive. Moreover, the dynamics of histone modifications are also unclear in plant meiocytes. Although significant progress in meiotic epigenetics has been made in the past 10 years, the association between histone modifications and meiotic recombination in plants is predominantly inferred from epigenomic sequencing data obtained from somatic cells [Citation72,Citation93,Citation94,Citation102]. Thus, the landscapes of meiotic histone modifications need to be further defined in order to understand the specific characteristics and regulatory mechanisms that govern meiotic heterochromatin condensation.
The roles of heterochromatin during meiosis are largely unknown in most species. Emerging evidence support the idea that recombination suppression in heterochromatin is a common feature in most eukaryotes [Citation11,Citation93,Citation100,Citation103,Citation137,Citation140], which could be mediated by several potential mechanisms. First, epigenetic factors enriched in heterochromatin involved in DNA methylation and H3K9me2 were demonstrated to function in repressing COs. Second, meiotic recombination initiates from DSBs, whose formation is commonly repressed in heterochromatin [Citation108,Citation141], likely due to the compact chromatin. Third, selection of the meiotic DSB repair pathways may be different in heterochromatin and euchromatin, providing a possible explanation why DSBs are observed in pericentromeric regions, but are rarely repaired as COs [Citation102,Citation108,Citation109]. Alternatively, the underlying mechanisms could also be different across species, due to differences in chromatin architecture. In S. pombe, the histone reader Swi6 specifically binds to H3K9me on pericentromeric heterochromatin, which impedes the recruitment of the machinery for meiotic DSB formation, including Spo11 [Citation81]. In mammalian, PRDM9 (a SET domain protein with the zinc finger) recognizes the specific DNA motifs and catalyzes H3K4me3 to promote DSB formation on euchromatin [Citation106,Citation107]. In the model plant Arabidopsis, DSB or recombination hotspots are also closely associated with H3K4me3 and specific motifs on euchromatin [Citation102,Citation199]. However, plants have neither a functionally conserved Swi6 ortholog nor an ortholog of PRDM9. Taking advantage of newly developed technologies such as ultramicro sample sequencing and super-resolution microscopy, will aid in the investigation of commonalities and differences in the roles of heterochromatin in meiosis across species.
Disclosure statement
No potential conflict of interest was reported by the author(s).
Additional information
Funding
References
- Luger K, Mader AW, Richmond RK, et al. Crystal structure of the nucleosome core particle at 2.8 Å resolution. Nature 1997; 389:251–18. 6648 doi: 10.1038/38444
- Hergeth SP, Schneider R. The H1 linker histones: multifunctional proteins beyond the nucleosomal core particle. EMBO Rep. 2015;16(11):1439–53. doi: 10.15252/embr.201540749
- Allshire RC, Madhani HD. Ten Principles of heterochromatin formation and function. Nat Rev Mol Cell Biol. 2018;19(4):229–44. doi: 10.1038/nrm.2017.119
- Bell O, Burton A, Dean C, et al. Heterochromatin definition and function. Nat Rev Mol Cell Biol. 2023;24(10):691–694. doi: 10.1038/s41580-023-00599-7
- Liu J, Ali M, Zhou Q. Establishment and evolution of heterochromatin. Ann N Y Acad Sci. 2020;1476(1):59–77. doi: 10.1111/nyas.14303
- Feng W, Michaels SD. Accessing the inaccessible: the organization, transcription, replication, and repair of heterochromatin in plants. Ann Rev Genet. 2015;49(1):439–59. doi: 10.1146/annurev-genet-112414-055048
- Zickler D, Kleckner N. Meiosis: dances between homologs. Ann Rev Genet 2023; 57. 1 1–63 doi: 10.1146/annurev-genet-061323-044915
- Ohkura H. Meiosis: an overview of key differences from mitosis. Cold Spring Harb Perspect Biol. 2015;7(5):a015859. doi: 10.1101/cshperspect.a015859
- Hirano T. Chromosome dynamics during mitosis. Cold Spring Harb Perspect Biol. 2015;7(6):a015792. doi: 10.1101/cshperspect.a015792
- Ma H. A molecular portrait of Arabidopsis meiosis. Arabidopsis Book. 2006;4:e0095. doi: 10.1199/tab.0095
- Wang Y, Copenhaver GP. Meiotic recombination: mixing it up in plants. Annu Rev Plant Biol. 2018;69(1):577–609. doi: 10.1146/annurev-arplant-042817-040431
- Zhang H, Lang Z, Zhu JK. Dynamics and function of DNA methylation in plants. Nat Rev Mol Cell Biol. 2018;19(8):489–506. doi: 10.1038/s41580-018-0016-z
- Wendte JM, Schmitz RJ. Specifications of targeting heterochromatin modifications in plants. Mol Plant. 2018;11(3):381–7. doi: 10.1016/j.molp.2017.10.002
- Saze H, Mittelsten Scheid O, Paszkowski J. Maintenance of CpG methylation is essential for epigenetic inheritance during plant gametogenesis. Nat Genet. 2003;34(1):65–9. doi: 10.1038/ng1138
- Kankel MW, Ramsey DE, Stokes TL, et al. Arabidopsis MET1 cytosine methyltransferase mutants. Genetics. 2003;163(3):1109–22. doi: 10.1093/genetics/163.3.1109
- Lindroth AM, Cao X, Jackson JP, et al. Requirement of CHROMOMETHYLASE3 for maintenance of CpXpG methylation. Science. 2001;292(5524):2077–80. doi: 10.1126/science.1059745
- Zhong X, Du J, Hale CJ, et al. Molecular mechanism of action of plant DRM de novo DNA methyltransferases. Cell. 2014;157(5):1050–60. doi: 10.1016/j.cell.2014.03.056
- Zemach A, Kim MY, Hsieh PH, et al. The Arabidopsis nucleosome remodeler DDM1 allows DNA methyltransferases to access H1-containing heterochromatin. Cell. 2013;153(1):193–205. doi: 10.1016/j.cell.2013.02.033
- Borg M, Jiang D, Berger F. Histone variants take center stage in shaping the epigenome. Curr Opin Plant Biol. 2021;61:101991. doi: 10.1016/j.pbi.2020.101991
- Benoit M, Simon L, Desset S, et al. Replication-coupled histone H3.1 deposition determines nucleosome composition and heterochromatin dynamics during Arabidopsis seedling development. New Phytol. 2019;221(1):385–98. doi: 10.1111/nph.15248
- Tagami H, Ray-Gallet D, Almouzni G, et al. Histone H3.1 and H3.3 complexes mediate nucleosome assembly pathways dependent or independent of DNA synthesis. Cell. 2004;116(1):51–61. doi: 10.1016/S0092-8674(03)01064-X
- Wollmann H, Stroud H, Yelagandula R, et al. The histone H3 variant H3.3 regulates gene body DNA methylation in Arabidopsis thaliana. Genome Biol. 2017;18(1):94. doi: 10.1186/s13059-017-1221-3
- Nie X, Wang H, Li J, et al. The HIRA complex that deposits the histone H3.3 is conserved in Arabidopsis and facilitates transcriptional dynamics. Biol Open 2014; 3:794–802. 9 10.1242/bio.20148680
- Stroud H, Otero S, Desvoyes B, et al. Genome-wide analysis of histone H3.1 and H3.3 variants in Arabidopsis thaliana. Proc Natl Acad Sci U S A. 2012;109(14):5370–5. doi: 10.1073/pnas.1203145109
- Ono T, Kaya H, Takeda S, et al. Chromatin assembly factor 1 ensures the stable maintenance of silent chromatin states in Arabidopsis. Genes Cells. 2006;11(2):153–62. doi: 10.1111/j.1365-2443.2006.00928.x
- Yelagandula R, Stroud H, Holec S, et al. The histone variant H2A.W defines heterochromatin and promotes chromatin condensation in Arabidopsis. 158:98–109. 2014 Cell 1 doi: 10.1016/j.cell.2014.06.006
- Choi J, Lyons DB, Kim MY, et al. DNA methylation and histone H1 jointly repress transposable elements and aberrant intragenic transcripts. Mol Cell 2020; 77:310–323.e7. 2 doi: 10.1016/j.molcel.2019.10.011
- Jiang D, Berger F. Histone variants in plant transcriptional regulation. Biochim Biophys Acta, Gene Regul Mech. 2017;1860(1):123–30. doi: 10.1016/j.bbagrm.2016.07.002
- Jacob Y, Bergamin E, Donoghue MT, et al. Selective methylation of histone H3 variant H3.1 regulates heterochromatin replication. Science 2014; 343:1249–1253. 6176 doi: 10.1126/science.1248357
- Ebbs ML, Bartee L, Bender J. H3 lysine 9 methylation is maintained on a transcribed inverted repeat by combined action of SUVH6 and SUVH4 methyltransferases. Mol Cell Biol. 2005;25(23):10507–15. doi: 10.1128/MCB.25.23.10507-10515.2005
- Ebbs ML, Bender J. Locus-specific control of DNA methylation by the Arabidopsis SUVH5 histone methyltransferase. Plant Cell. 2006;18(5):1166–76. doi: 10.1105/tpc.106.041400
- Du J, Zhong X, Bernatavichute YV, et al. Dual binding of chromomethylase domains to H3K9me2-containing nucleosomes directs DNA methylation in plants. Cell. 2012;151(1):167–80. doi: 10.1016/j.cell.2012.07.034
- Nishibuchi G, Nakayama J. Biochemical and structural properties of heterochromatin protein 1: understanding its role in chromatin assembly. J Biochem. 2014;156(1):11–20. doi: 10.1093/jb/mvu032
- Sanulli S, Trnka MJ, Dharmarajan V, et al. HP1 reshapes nucleosome core to promote heterochromatin phase separation. Nature 2019. 575 7782 390–394 doi: 10.1038/s41586-019-1669-2
- Lachner M, O’Carroll D, Rea S, et al. Methylation of histone H3 lysine 9 creates a binding site for HP1 proteins. Nature. 2001;410(6824):116–20. doi: 10.1038/35065132
- Kwon SH, Workman JL. The heterochromatin protein 1 (HP1) family: put away a bias toward HP1. Mol Cells. 2008;26(3):217–27. doi: 10.1016/S1016-8478(23)13988-4
- Wang H, Jiang D, Axelsson E, et al. LHP1 interacts with ATRX through plant-specific domains at specific loci targeted by PRC2. Mol Plant. 2018;11(8):1038–52. doi: 10.1016/j.molp.2018.05.004
- Zhang X, Germann S, Blus BJ, et al. The Arabidopsis LHP1 protein colocalizes with histone H3 Lys27 trimethylation. Nat Struct Mol Biol. 2007;14(9):869–71. doi: 10.1038/nsmb1283
- Zhao S, Cheng L, Gao Y, et al. Plant HP1 protein ADCP1 links multivalent H3K9 methylation readout to heterochromatin formation. Cell Res 2018. 29 1 54–66 doi: 10.1038/s41422-018-0104-9
- Zhang C, Du X, Tang K, et al. Arabidopsis AGDP1 links H3K9me2 to DNA methylation in heterochromatin. Nat Commun. 2018;9(1):4547. doi: 10.1038/s41467-018-06965-w
- Simon JA, Kingston RE. Mechanisms of polycomb gene silencing: knowns and unknowns. Nat Rev Mol Cell Biol. 2009;10(10):697–708. doi: 10.1038/nrm2763
- Xiao J, Wagner D. Polycomb repression in the regulation of growth and development in Arabidopsis. Curr Opin Plant Biol. 2015;23:15–24. doi: 10.1016/j.pbi.2014.10.003
- Zhang X, Clarenz O, Cokus S, et al. Whole-genome analysis of histone H3 lysine 27 trimethylation in Arabidopsis. PLoS Biol. 2007;5(5):e129. doi: 10.1371/journal.pbio.0050129
- Mathieu O, Probst AV, Paszkowski J. Distinct regulation of histone H3 methylation at lysines 27 and 9 by CpG methylation in Arabidopsis. EMBO J. 2005;24(15):2783–91. doi: 10.1038/sj.emboj.7600743
- Jacob Y, Feng S, LeBlanc CA, et al. ATXR5 and ATXR6 are H3K27 monomethyltransferases required for chromatin structure and gene silencing. Nat Struct Mol Biol. 2009;16(7):763–8. doi: 10.1038/nsmb.1611
- Potok ME, Zhong Z, Picard CL, et al. The role of ATXR6 expression in modulating genome stability and transposable element repression in Arabidopsis. Proc Natl Acad Sci U S A. 2022;119(3):119. doi: 10.1073/pnas.2115570119
- Dong J, LeBlanc C, Poulet A, et al. H3.1K27me1 maintains transcriptional silencing and genome stability by preventing GCN5-mediated histone acetylation. Plant Cell. 2021;33(4):961–979. doi:10.1093/plcell/koaa027
- Jacob Y, Stroud H, Leblanc C, et al. Regulation of heterochromatic DNA replication by histone H3 lysine 27 methyltransferases. Nature. 2010;466(7309):987–91. doi: 10.1038/nature09290
- Moissiard G, Cokus SJ, Cary J, et al. MORC family ATPases required for heterochromatin condensation and gene silencing. Science. 2012;336(6087):1448–51. doi: 10.1126/science.1221472
- Liu ZW, Zhou JX, Huang HW, et al. Two components of the RNA-Directed DNA methylation pathway associate with MORC6 and silence loci targeted by MORC6 in Arabidopsis. PloS Genet. 2016;12(5):e1006026. doi: 10.1371/journal.pgen.1006026
- Jing Y, Sun H, Yuan W, et al. SUVH2 and SUVH9 couple two essential steps for transcriptional gene silencing in Arabidopsis. Mol Plant. 2016;9(8):1156–67. doi: 10.1016/j.molp.2016.05.006
- Xue Y, Zhong Z, Harris CJ, et al. Arabidopsis MORC proteins function in the efficient establishment of RNA directed DNA methylation. Nat Commun. 2021;12(1):12. doi: 10.1038/s41467-021-24553-3
- Harris CJ, Husmann D, Liu WL, et al. Arabidopsis AtMORC4 and AtMORC7 form nuclear bodies and repress a large number of protein-coding genes. PloS Genet. 2016;12(5):e1005998. doi: 10.1371/journal.pgen.1005998
- Liu Q, Gong Z. The coupling of epigenome replication with DNA replication. Curr Opin Plant Biol. 2011;14(2):187–94. doi: 10.1016/j.pbi.2010.12.001
- Jiang D, Berger F DNA replication–coupled histone modification maintains Polycomb gene silencing in plants. Science 2017; 357:1146–1149. 6356 doi: 10.1126/science.aan4965
- Davarinejad H, Joshi M, Ait-Hamou N, et al. ATXR5/6 forms alternative protein complexes with PCNA and the nucleosome core particle. J Mol Biol. 2019;431(7):1370–9. doi: 10.1016/j.jmb.2019.02.020
- Li F, Martienssen R, Cande WZ Coordination of DNA replication and histone modification by the Rik1–Dos2 complex. Nature 2011; 475:244–248. 7355 doi: 10.1038/nature10161
- He H, Li Y, Dong Q, et al. Coordinated regulation of heterochromatin inheritance by Dpb3–Dpb4 complex. Proc Natl Acad Sci U S A 2017; 114:12524–12529. 47 doi: 10.1073/pnas.1712961114
- Smith JS, Caputo E, Boeke JD. A genetic screen for ribosomal DNA silencing defects identifies multiple DNA replication and chromatin-modulating factors. Mol Cell Biol. 1999;19(4):3184–97. doi: 10.1128/MCB.19.4.3184
- Iida T, Araki H Noncompetitive Counteractions of DNA polymerase ε and ISW2/yCHRAC for epigenetic inheritance of telomere position effect in Saccharomyces cerevisiae. Mol Cell Biol 2004; 24:217–227. 1 doi: 10.1128/MCB.24.1.217-227.2004
- Bourguet P, Lopez-Gonzalez L, Gomez-Zambrano A, et al. DNA polymerase epsilon is required for heterochromatin maintenance in Arabidopsis. Genome Biol 2020; 21:283. 1 doi: 10.1186/s13059-020-02190-1
- Mainiero S, Pawlowski WP. Meiotic chromosome structure and function in plants. Cytogenet Genome Res. 2014;143(1–3):6–17. doi: 10.1159/000365260
- Kleckner N. Chiasma formation: chromatin/axis interplay and the role(s) of the synaptonemal complex. Chromosoma. 2006;115(3):175–94. doi: 10.1007/s00412-006-0055-7
- Ferdous M, Higgins JD, Osman K, et al. Inter-homolog crossing-over and synapsis in Arabidopsis meiosis are dependent on the chromosome axis protein AtASY3. PloS Genet. 2012;8(2):e1002507. doi: 10.1371/journal.pgen.1002507
- Armstrong SJ, Caryl AP, Jones GH, et al. Asy1, a protein required for meiotic chromosome synapsis, localizes to axis-associated chromatin in Arabidopsis and Brassica. J Cell Sci. 2002;115(18):3645–55. doi: 10.1242/jcs.00048
- Chambon A, West A, Vezon D, et al. Identification of ASYNAPTIC4, a component of the meiotic chromosome axis. Plant Physiol 2018; 178:233–246. 1 doi: 10.1104/pp.17.01725
- Hagstrom KA, Meyer BJ. Condensin and cohesin: more than chromosome compactor and glue. Nat Rev Genet. 2003;4(7):520–34. doi: 10.1038/nrg1110
- Schubert V. SMC proteins and their multiple functions in higher plants. Cytogenet Genome Res. 2009;124(3–4):202–14. doi: 10.1159/000218126
- Heldrich J, Milano CR, Markowitz TE, et al. Two pathways drive meiotic chromosome axis assembly in Saccharomyces cerevisiae. Nucleic Acids Res 2022; 50:4545–4556. 8 doi: 10.1093/nar/gkac227
- Fujiwara Y, Horisawa-Takada Y, Inoue E, et al. Meiotic cohesins mediate initial loading of HORMAD1 to the chromosomes and coordinate SC formation during meiotic prophase. PloS Genet. 2020;16(9):e1009048. doi: 10.1371/journal.pgen.1009048
- Lee J. Roles of cohesin and condensin in chromosome dynamics during Mammalian meiosis. J Reprod Dev. 2013;59(5):431–6. doi: 10.1262/jrd.2013-068
- Lambing C, Tock AJ, Topp SD, et al. Interacting genomic landscapes of REC8-cohesin, chromatin, and meiotic recombination in Arabidopsis. Plant Cell. 2020;32(4):1218–39. doi: 10.1105/tpc.19.00866
- Mercier R, De K, Sterle L, et al. Arabidopsis thaliana WAPL is Essential for the Prophase Removal of Cohesin during Meiosis. PloS Genet 2014; 10. 10 7 doi: 10.1371/journal.pgen.1004497
- Zamariola L, De Storme N, Vannerum K, et al. Shugoshins and PATRONUS protect meiotic centromere cohesion in Arabidopsis thaliana. Plant J. 2014;77(5):782–94. doi: 10.1111/tpj.12432
- Sakuno T, Hiraoka Y. Rec8 Cohesin: a structural platform for shaping the meiotic chromosomes. Genes (Basel). 2022;13(2):200. doi: 10.3390/genes13020200
- Sebastian J, Ravi M, Andreuzza S, et al. The plant adherin AtSCC2 is required for embryogenesis and sister‐chromatid cohesion during meiosis in Arabidopsis. Plant J. 2009;59(1):1–13. doi: 10.1111/j.1365-313X.2009.03845.x
- Wang H, Xu W, Sun Y, et al. The cohesin loader SCC2 contains a PHD finger that is required for meiosis in land plants. PloS Genet. 2020;16(6):e1008849. doi: 10.1371/journal.pgen.1008849
- Cai X, Dong FG, Edelmann RE, et al. The Arabidopsis SYN1 cohesin protein is required for sister chromatid arm cohesion and homologous chromosome pairing. J Cell Sci. 2003;116(14):2999–3007. doi: 10.1242/jcs.00601
- Ito M, Kugou K, Fawcett JA, et al. Meiotic recombination cold spots in chromosomal cohesion sites. Genes Cells. 2014;19(5):359–73. doi: 10.1111/gtc.12138
- Folco HD, Chalamcharla VR, Sugiyama T, et al. Untimely expression of gametogenic genes in vegetative cells causes uniparental disomy. Nature. 2017;543(7643):126–30. doi: 10.1038/nature21372
- Nambiar M, Smith GR Pericentromere-Specific Cohesin Complex Prevents Meiotic Pericentric DNA Double-Strand Breaks and Lethal Crossovers. Mol Cell 2018; 71:540–553.e4. 4 doi: 10.1016/j.molcel.2018.06.035
- Wang J, Yu C, Zhang S, et al. Cell-type-dependent histone demethylase specificity promotes meiotic chromosome condensation in Arabidopsis. Nat Plants. 2020;6(7):823–37. doi: 10.1038/s41477-020-0697-0
- Wang J, Niu B, Huang J, et al. The PHD finger protein MMD1/DUET ensures the progression of male meiotic chromosome condensation and directly regulates the expression of the condensin gene CAP-D3. Plant Cell. 2016;28(8):1894–909. doi: 10.1105/tpc.16.00040
- Li X, Wang H, Wang Y, et al. Comparison of metabolic profiling of arabidopsis inflorescences between landsberg erecta and Columbia, and Meiosis-Defective Mutants by (1)H-NMR Spectroscopy. Phenomics 2021; 1:73–89. 2 doi: 10.1007/s43657-021-00012-3
- Oliver C, Pradillo M, Corredor E, et al. The dynamics of histone H3 modifications is species-specific in plant meiosis. Planta. 2013;238(1):23–33. doi: 10.1007/s00425-013-1885-1
- Wang C, Huang J, Li Y, et al. DNA polymerase epsilon binds histone H3.1-H4 and recruits MORC1 to mediate meiotic heterochromatin condensation. Proc Natl Acad Sci U S A. 2022;119(43):e2213540119. doi: 10.1073/pnas.2213540119
- Wang C, Huang J, Zhang J, et al. DNA polymerase epsilon interacts with SUVH2/9 to repress the expression of genes associated with meiotic DSB hotspot in Arabidopsis. Proc Natl Acad Sci U S A. 2022;119(41):e2208441119. doi: 10.1073/pnas.2208441119
- Watson ML, Zinn AR, Inoue N, et al. Identification of morc (microrchidia), a mutation that results in arrest of spermatogenesis at an early meiotic stage in the mouse. Proc Natl Acad Sci U S A. 1998;95(24):14361–6. doi: 10.1073/pnas.95.24.14361
- Pastor WA, Stroud H, Nee K, et al. MORC1 represses transposable elements in the mouse male germline. Nat Commun. 2014;5(1). doi: 10.1038/ncomms6795
- Weiser NE, Yang DX, Feng S, et al. MORC-1 Integrates Nuclear RNAi and Transgenerational Chromatin Architecture to Promote Germline Immortality. Dev Cell 2017; 41:408–423.e7. 4 doi: 10.1016/j.devcel.2017.04.023
- Keeney S, Giroux CN, Kleckner N. Meiosis-specific DNA double-strand breaks are catalyzed by Spo11, a member of a widely conserved protein family. Cell. 1997;88(3):375–84. doi: 10.1016/S0092-8674(00)81876-0
- Lambing C, Franklin FC, Wang CR Understanding and Manipulating Meiotic Recombination in Plants. Plant Physiol 2017; 173:1530–1542. 3 10.1104/pp.16.01530
- Tock AJ, Henderson IR. Hotspots for initiation of meiotic recombination. Front Genet. 2018;9:521. doi: 10.3389/fgene.2018.00521
- Underwood CJ, Choi K, Lambing C, et al. Epigenetic activation of meiotic recombination near Arabidopsis thaliana centromeres via loss of H3K9me2 and non-CG DNA methylation. Genome Res. 2018;28(4):519–31. doi: 10.1101/gr.227116.117
- Yelina NE, Lambing C, Hardcastle TJ, et al. DNA methylation epigenetically silences crossover hot spots and controls chromosomal domains of meiotic recombination in Arabidopsis. Genes Dev. 2015;29(20):2183–202. doi: 10.1101/gad.270876.115
- Yelina N, Diaz P, Lambing C, et al. Epigenetic control of meiotic recombination in plants. Sci China Life Sci. 2015;58(3):223–31. doi: 10.1007/s11427-015-4811-x
- Yelina NE, Choi K, Chelysheva L, et al. Epigenetic remodeling of meiotic crossover frequency in Arabidopsis thaliana DNA methyltransferase mutants. PloS Genet. 2012;8(8):e1002844. doi: 10.1371/journal.pgen.1002844
- Melamed-Bessudo C, Levy AA. Deficiency in DNA methylation increases meiotic crossover rates in euchromatic but not in heterochromatic regions in Arabidopsis. Proc Natl Acad Sci U S A. 2012;109(16):E981–E8. doi: 10.1073/pnas.1120742109
- Colome-Tatche M, Cortijo S, Wardenaar R, et al. Features of the Arabidopsis recombination landscape resulting from the combined loss of sequence variation and DNA methylation. Proc Natl Acad Sci U S A. 2012;109(40):16240–5. doi: 10.1073/pnas.1212955109
- Fernandes JB, Wlodzimierz P, Henderson IR. Meiotic recombination within plant centromeres. Curr Opin Plant Biol. 2019;48:26–35. doi: 10.1016/j.pbi.2019.02.008
- Lange J, Yamada S, Tischfield SE, et al. The landscape of mouse meiotic double-strand break formation, processing, and repair. Cell 2016; 167:695–708.e16. 3 doi: 10.1016/j.cell.2016.09.035
- Choi K, Zhao X, Kelly KA, et al. Arabidopsis meiotic crossover hot spots overlap with H2A.Z nucleosomes at gene promoters. Nat Genet. 2013;45(11):1327–36. doi: 10.1038/ng.2766
- Baudat F, Imai Y, de Massy B. Meiotic recombination in mammals: localization and regulation. Nat Rev Genet. 2013;14(11):794–806. doi: 10.1038/nrg3573
- Borde V, de Massy B. Programmed induction of DNA double strand breaks during meiosis: setting up communication between DNA and the chromosome structure. Curr Opin Genet Dev. 2013;23(2):147–55. doi: 10.1016/j.gde.2012.12.002
- Xie C, Wang W, Tu C, et al. Meiotic recombination: insights into its mechanisms and its role in human reproduction with a special focus on non-obstructive azoospermia. Hum Reprod Update 2022. 28 6 763–797 doi: 10.1093/humupd/dmac024
- Paiano J, Wu W, Yamada S, et al. ATM and PRDM9 regulate SPO11-bound recombination intermediates during meiosis. Nat Commun. 2020;11(1):857. doi: 10.1038/s41467-020-14654-w
- Baudat F, Buard J, Grey C, et al. PRDM9 is a major determinant of meiotic recombination hotspots in humans and mice. Science. 2010;327(5967):836–40. doi: 10.1126/science.1183439
- Choi K, Zhao X, Tock AJ, et al. Nucleosomes and DNA methylation shape meiotic DSB frequency in Arabidopsis thaliana transposons and gene regulatory regions. Genome Res. 2018;28(4):532–46. doi: 10.1101/gr.225599.117
- Naish M, Alonge M, Wlodzimierz P, et al. The genetic and epigenetic landscape of the Arabidopsis centromeres. Science. 2021;374(6569):eabi7489. doi: 10.1126/science.abi7489
- Petes TD. Meiotic recombination hot spots and cold spots. Nat Rev Genet. 2001;2(5):360–9. doi: 10.1038/35072078
- Nambiar M, Smith GR. Repression of harmful meiotic recombination in centromeric regions. Semin Cell Dev Biol. 2016;54:188–97. doi: 10.1016/j.semcdb.2016.01.042
- Stewart MN, Dawson DS. Changing partners: moving from non-homologous to homologous centromere pairing in meiosis. Trends Genet. 2008;24(11):564–73. doi: 10.1016/j.tig.2008.08.006
- Hassold T, Hunt P. To err (meiotically) is human: the genesis of human aneuploidy. Nat Rev Genet. 2001;2(4):280–91. doi: 10.1038/35066065
- Rockmill B, Voelkel-Meiman K, Roeder GS. Centromere-proximal crossovers are associated with precocious separation of sister chromatids during meiosis in Saccharomyces cerevisiae. Genetics. 2006;174(4):1745–54. doi: 10.1534/genetics.106.058933
- Si W, Yuan Y, Huang J, et al. Widely distributed hot and cold spots in meiotic recombination as shown by the sequencing of rice F2 plants. New Phytol. 2015;206(4):1491–502. doi: 10.1111/nph.13319
- Marand AP, Zhao H, Zhang W, et al. Historical meiotic crossover hotspots fueled patterns of evolutionary divergence in rice. Plant Cell. 2019;31(3):645–62. doi: 10.1105/tpc.18.00750
- Fuentes RR, de Ridder D, van Dijk ADJ, et al. Domestication shapes recombination patterns in tomato. Mol Biol Evol 2022; 39. 39 1 10.1093/molbev/msab287
- Demirci S, van Dijk ADJ, Perez GS, et al. Distribution, position and genomic characteristics of crossovers in tomato recombinant inbred lines derived from an interspecific cross between Solanum lycopersicum and Solanum pimpinellifolium. Plant J 2017; 89:554–564. 3 doi: 10.1111/tpj.13406
- Tock AJ, Holland DM, Jiang W, et al. Crossover-active regions of the wheat genome are distinguished by DMC1, the chromosome axis, H3K27me3, and signatures of adaptation. Genome Res. 2021;31(9):1614–28. doi: 10.1101/gr.273672.120
- Saintenac C, Faure S, Remay A, et al. Variation in crossover rates across a 3-Mb contig of bread wheat (Triticum aestivum) reveals the presence of a meiotic recombination hotspot. Chromosoma. 2011;120(2):185–98. doi: 10.1007/s00412-010-0302-9
- Mayer KFX, Rogers J, Doležel J, et al. A chromosome-based draft sequence of the hexaploid bread wheat (Triticum aestivum) genome. Science 2014; 345:1251788. 6194 doi: 10.1126/science.1251788
- He Y, Wang M, Dukowic-Schulze S, et al. Genomic features shaping the landscape of meiotic double-strand-break hotspots in maize. Proc Natl Acad Sci U S A. 2017;114(46):12231–6. doi: 10.1073/pnas.1713225114
- He Y, Wang M, Sun Q, et al. Mapping recombination initiation sites using chromatin immunoprecipitation. Methods Mol Biol 2016; 1429:177–188.
- Mascher M, Gundlach H, Himmelbach A, et al. A chromosome conformation capture ordered sequence of the barley genome. Nature. 2017;544(7651):427–33. doi: 10.1038/nature22043
- McConaughy S, Amundsen K, Song Q, et al. Recombination hotspots in soybean [Glycine ma (L.) Merr.] [Glycine max (L.) Merr.]. G3: Genes | Genomes | Genetics. 2023;13. doi: 10.1093/g3journal/jkad075
- Ma X, Fan L, Zhang Z, et al. Global dissection of the recombination landscape in soybean using a high-density 600K SoySNP array. Plant Biotechnol J. 2023;21(3):606–20. doi: 10.1111/pbi.13975
- Mirouze M, Lieberman-Lazarovich M, Aversano R, et al. Loss of DNA methylation affects the recombination landscape in Arabidopsis. Proc Natl Acad Sci U S A. 2012;109(15):5880–5. doi: 10.1073/pnas.1120841109
- Ellermeier C, Higuchi EC, Phadnis N, et al. Rnai and heterochromatin repress centromeric meiotic recombination. Proc Natl Acad Sci U S A. 2010;107(19):8701–5. doi: 10.1073/pnas.0914160107
- Zamudio N, Barau J, Teissandier A, et al. DNA methylation restrains transposons from adopting a chromatin signature permissive for meiotic recombination. Genes Dev. 2015;29(12):1256–70. doi: 10.1101/gad.257840.114
- Saintenac C, Falque M, Martin OC, et al. Detailed recombination studies along chromosome 3B provide new insights on crossover distribution in wheat (triticum aestivum L.). Genetics. 2009;181(2):393–403. doi: 10.1534/genetics.108.097469
- Kong A, Gudbjartsson DF, Sainz J, et al. A high-resolution recombination map of the human genome. Nat Genet. 2002;31(3):241–7. doi: 10.1038/ng917
- Giraut L, Falque M, Drouaud J, et al. Genome-wide crossover distribution in Arabidopsis thaliana meiosis reveals sex-specific patterns along chromosomes. PloS Genet. 2011;7(11):e1002354. doi: 10.1371/journal.pgen.1002354
- Liu S, Yeh CT, Ji T, et al. Mu transposon insertion sites and meiotic recombination events co-localize with epigenetic marks for open chromatin across the maize genome. PloS Genet. 2009;5(11):e1000733. doi: 10.1371/journal.pgen.1000733
- Darrier B, Rimbert H, Balfourier F, et al. High-resolution mapping of crossover events in the hexaploid wheat genome suggests a universal recombination mechanism. Genetics. 2017;206(3):1373–88. doi: 10.1534/genetics.116.196014
- Buhler C, Borde V, Lichten M, et al. Mapping meiotic single-strand DNA reveals a new landscape of DNA double-strand breaks in Saccharomyces cerevisiae. PLoS Biol. 2007;5(12):e324. doi: 10.1371/journal.pbio.0050324
- Su Y, Barton AB, Kaback DB. Decreased meiotic reciprocal recombination in subtelomeric regions in Saccharomyces cerevisiae. Chromosoma. 2000;109(7):467–75. doi: 10.1007/s004120000098
- Barton AB, Pekosz MR, Kurvathi RS, et al. Meiotic recombination at the ends of chromosomes in Saccharomyces cerevisiae. Genetics. 2008;179(3):1221–35. doi: 10.1534/genetics.107.083493
- Chen SY, Tsubouchi T, Rockmill B, et al. Global analysis of the meiotic crossover landscape. Dev Cell. 2008;15(3):401–15. doi: 10.1016/j.devcel.2008.07.006
- Salome PA, Bomblies K, Fitz J, et al. The recombination landscape in Arabidopsis thaliana F2 populations. Heredity (Edinb). 2012;108(4):447–55. doi: 10.1038/hdy.2011.95
- Jensen-Seaman MI, Furey TS, Payseur BA, et al. Comparative recombination rates in the rat, mouse, and human genomes. Genome Res. 2004;14(4):528–38. doi: 10.1101/gr.1970304
- Blitzblau HG, Bell GW, Rodriguez J, et al. Mapping of meiotic single-stranded DNA reveals double-strand-break hotspots near centromeres and telomeres. Curr Biol. 2007;17(23):2003–12. doi: 10.1016/j.cub.2007.10.066
- McKinley KL, Cheeseman IM. The molecular basis for centromere identity and function. Nat Rev Mol Cell Biol. 2016;17(1):16–29. doi: 10.1038/nrm.2015.5
- Simon L, Voisin M, Tatout C, et al. Structure and function of centromeric and pericentromeric heterochromatin in Arabidopsis thaliana. Front Plant Sci. 2015;6:1049. doi: 10.3389/fpls.2015.01049
- Eyster C, Chuong HH, Lee CY, et al. The pericentromeric heterochromatin of homologous chromosomes remains associated after centromere pairing dissolves in mouse spermatocyte meiosis. Chromosoma. 2019;128(3):355–67. doi: 10.1007/s00412-019-00708-6
- Da Ines O, Abe K, Goubely C, et al. Differing requirements for RAD51 and DMC1 in meiotic pairing of centromeres and chromosome arms in Arabidopsis thaliana. PloS Genet. 2012;8(4):e1002636. doi: 10.1371/journal.pgen.1002636
- Dernburg AF, Sedat JW, Hawley RS. Direct evidence of a role for heterochromatin in meiotic chromosome segregation. Cell. 1996;86(1):135–46. doi: 10.1016/S0092-8674(00)80084-7
- Griffiths S, Sharp R, Foote TN, et al. Molecular characterization of Ph1 as a major chromosome pairing locus in polyploid wheat. Nature. 2006;439(7077):749–52. doi: 10.1038/nature04434
- Da Ines O, White CI. Centromere associations in meiotic chromosome pairing. Ann Rev Genet. 2015;49(1):95–114. doi: 10.1146/annurev-genet-112414-055107
- Zhang J, Han F. Centromere pairing precedes meiotic chromosome pairing in plants. Sci China Life Sci. 2017;60(11):1197–202. doi: 10.1007/s11427-017-9109-y
- Kurdzo EL, Dawson DS Centromere pairing – tethering partner chromosomes in meiosis I. FEBS J 2015; 282:2445–2457. 13 doi: 10.1111/febs.13280
- Kurdzo EL, Obeso D, Chuong H, et al. Meiotic centromere coupling and pairing function by two separate mechanisms in Saccharomyces cerevisiae. Genetics. 2017;205(2):657–71. doi: 10.1534/genetics.116.190264
- Hawley RS, Qiao H, Chen JK, et al. Interplay between synaptonemal complex, homologous recombination, and centromeres during Mammalian Meiosis. PloS Genet 2012; 8. 6 e1002790 doi: 10.1371/journal.pgen.1002790
- Hawley RS, Bisig CG, Guiraldelli MF, et al. Synaptonemal complex components persist at centromeres and are required for homologous centromere pairing in Mouse Spermatocytes. PloS Genet 2012; 8. 6 e1002701 doi: 10.1371/journal.pgen.1002701
- Hunter N, Hopkins J, Hwang G, et al. Meiosis-specific cohesin component, Stag3 is essential for maintaining centromere chromatid cohesion, and required for DNA repair and synapsis between homologous chromosomes. PloS Genet 2014; 10. 10 7 doi: 10.1371/journal.pgen.1004413
- Chuong H, Dawson DS, Stearns T. Meiotic cohesin promotes pairing of nonhomologous centromeres in early meiotic prophase. Mol Biol Cell. 2010;21(11):1799–809. doi: 10.1091/mbc.e09-05-0392
- Tsubouchi T, AJ M, Roeder GS Initiation of meiotic chromosome synapsis at centromeres in budding yeast. Genes Dev 2008; 22:3217–3226. 22 doi: 10.1101/gad.1709408
- Bolanos-Villegas P, De K, Pradillo M, et al. In favor of establishment: regulation of chromatid cohesion in plants. Front Plant Sci. 2017;8. doi: 10.3389/fpls.2017.00846
- Zhang J, Pawlowski WP, Han F. Centromere pairing in early meiotic prophase requires active centromeres and precedes installation of the synaptonemal complex in maize. Plant Cell. 2013;25(10):3900–9. doi: 10.1105/tpc.113.117846
- Martinez-Perez E, Shaw P, Moore G. The Ph1 locus is needed to ensure specific somatic and meiotic centromere association. Nature. 2001;411(6834):204–7. doi: 10.1038/35075597
- Martinez‐Perez E, Shaw P, Aragon‐Alcaide L, et al. Chromosomes form into seven groups in hexaploid and tetraploid wheat as a prelude to meiosis. Plant J. 2003;36(1):21–9. doi: 10.1046/j.1365-313X.2003.01853.x
- Greer E, Martin AC, Pendle A, et al. The Ph1 locus suppresses Cdk2-type activity during premeiosis and meiosis in wheat. Plant Cell 2012; 24:152–162. 1 10.1105/tpc.111.094771
- Shakirov EV, Chen JJ, Shippen DE Plant telomere biology: The green solution to the end-replication problem. Plant Cell 2022; 34:2492–2504. 7 10.1093/plcell/koac122
- Roberts NY, Osman K, Armstrong SJ. Telomere Distribution and Dynamics in Somatic and Meiotic Nuclei of Arabidopsis thaliana. Cytogenet Genome Res. 2009;124(3–4):193–201. doi: 10.1159/000218125
- Tomita K, Cooper JP. The telomere bouquet controls the meiotic spindle. Cell. 2007;130(1):113–26. doi: 10.1016/j.cell.2007.05.024
- Koszul R, Kleckner N. Dynamic chromosome movements during meiosis: a way to eliminate unwanted connections? Trends Cell Biol. 2009;19(12):716–24. doi: 10.1016/j.tcb.2009.09.007
- Mytlis A, Levy K, Elkouby YM. The many faces of the bouquet centrosome MTOC in meiosis and germ cell development. Curr Opin Cell Biol. 2023;81:102158. doi: 10.1016/j.ceb.2023.102158
- Klutstein M, Fennell A, Fernandez-Alvarez A, et al. The telomere bouquet regulates meiotic centromere assembly. Nat Cell Biol. 2015;17(4):458–69. doi: 10.1038/ncb3132
- Chikashige Y, Haraguchi T, Hiraoka Y. Another way to move chromosomes. Chromosoma. 2007;116(6):497–505. doi: 10.1007/s00412-007-0114-8
- Chikashige Y, Tsutsumi C, Yamane M, et al. Meiotic proteins bqt1 and bqt2 tether telomeres to form the bouquet arrangement of chromosomes. Cell. 2006;125(1):59–69. doi: 10.1016/j.cell.2006.01.048
- Chikashige Y, Yamane M, Okamasa K, et al. Membrane proteins Bqt3 and -4 anchor telomeres to the nuclear envelope to ensure chromosomal bouquet formation. J Cell Bio. 2009;187(3):413–27. doi: 10.1083/jcb.200902122
- Stewart CL, Burke B. The missing LINC: a mammalian KASH-domain protein coupling meiotic chromosomes to the cytoskeleton. Nucleus. 2014;5(1):3–10. doi: 10.4161/nucl.27819
- Horn HF, Kim DI, Wright GD, et al. A mammalian KASH domain protein coupling meiotic chromosomes to the cytoskeleton. J Cell Bio 2013; 202:1023–1039. 7 10.1083/jcb.201304004
- Ding X, Xu R, Yu J, et al. SUN1 is required for telomere attachment to nuclear envelope and gametogenesis in mice. Dev Cell. 2007;12(6):863–72. doi: 10.1016/j.devcel.2007.03.018
- Spindler M-C, Redolfi J, Helmprobst F, et al. Electron tomography of mouse LINC complexes at meiotic telomere attachment sites with and without microtubules. Commun Biol. 2019;2(1). doi: 10.1038/s42003-019-0621-1
- Jantsch V, Link J, Leubner M, et al. Analysis of meiosis in SUN1 deficient mice reveals a distinct role of SUN2 in Mammalian Meiotic LINC Complex Formation and Function. PloS Genet 2014; 10. 10 2 doi: 10.1371/journal.pgen.1004099
- Morimoto A, Shibuya H, Zhu X, et al. A conserved KASH domain protein associates with telomeres, SUN1, and dynactin during mammalian meiosis. J Cell Bio 2012; 198:165–172. 2 10.1083/jcb.201204085
- Garner KEL, Salter A, Lau CK, et al. The meiotic LINC complex component KASH5 is an activating adaptor for cytoplasmic dynein. J Cell Bio. 2023;222(5):222. doi: 10.1083/jcb.202204042
- Agrawal R, Gillies JP, Zang JL, et al. The KASH5 protein involved in meiotic chromosomal movements is a novel dynein activating adaptor. Elife. 2022;11:11. doi: 10.7554/eLife.78201
- Da Ines O, Gallego ME, White CI. Recombination-independent mechanisms and pairing of homologous chromosomes during meiosis in plants. Mol Plant. 2014;7(3):492–501. doi: 10.1093/mp/sst172
- Armstrong SJ, Franklin FC, Jones GH. Nucleolus-associated telomere clustering and pairing precede meiotic chromosome synapsis in Arabidopsis thaliana. J Cell Sci. 2001;114(23):4207–17. doi: 10.1242/jcs.114.23.4207
- Zhang F, Ma L, Zhang C, et al. The SUN Domain proteins OsSUN1 and OsSUN2 play critical but partially redundant roles in meiosis. Plant Physiol. 2020;183(4):1517–30. doi: 10.1104/pp.20.00140
- Varas J, Graumann K, Osman K, et al. Absence of SUN1 and SUN2 proteins in Arabidopsis thaliana leads to a delay in meiotic progression and defects in synapsis and recombination. Plant J. 2015;81(2):329–46. doi: 10.1111/tpj.12730
- Corredor E, Lukaszewski AJ, Pachon P, et al. Terminal Regions of Wheat Chromosomes Select Their Pairing Partners in Meiosis. Genetics 2007; 177:699–706. 2 doi: 10.1534/genetics.107.078121
- Higgins JD, Perry RM, Barakate A, et al. Spatiotemporal asymmetry of the meiotic program underlies the predominantly distal distribution of meiotic crossovers in barley. Plant Cell. 2012;24(10):4096–109. doi: 10.1105/tpc.112.102483
- Golubovskaya IN, Harper LC, Pawlowski WP, et al. The pam1 gene is required for meiotic bouquet formation and efficient homologous synapsis in maize (Zea mays L.). Genetics. 2002;162(4):1979–93. doi: 10.1093/genetics/162.4.1979
- Jiang P, Lian B, Liu C, et al. 21-nt phasiRnas direct target mRNA cleavage in rice male germ cells. Nat Commun. 2020;11(1):5191. doi: 10.1038/s41467-020-19034-y
- Wang Y, Cheng Z, Lu P, et al. Molecular cell biology of male meiotic chromosomes and isolation of male meiocytes in Arabidopsis thaliana. Methods Mol Biol 2014; 1110:217–230.
- Wang C, Li X, Huang J, et al. Isolation of meiocytes and cytological analyses of male meiotic chromosomes in Soybean, Lettuce, and Maize. Methods Mol Biol 2023; 2686:219–239.
- Long J, Walker J, She W, et al. Nurse cell–derived small RNAs define paternal epigenetic inheritance in Arabidopsis. Science. 2021;373(6550). doi: 10.1126/science.abh0556
- Walker J, Gao H, Zhang J, et al. Sexual-lineage-specific DNA methylation regulates meiosis in Arabidopsis. Nat Genet. 2018;50(1):130–7. doi: 10.1038/s41588-017-0008-5
- Smallwood SA, Lee HJ, Angermueller C, et al. Single-cell genome-wide bisulfite sequencing for assessing epigenetic heterogeneity. Nat Methods. 2014;11(8):817–20. doi: 10.1038/nmeth.3035
- Kaya-Okur HS, Wu SJ, Codomo CA, et al. CUT&Tag for efficient epigenomic profiling of small samples and single cells. Nat Commun. 2019;10(1):1930. doi: 10.1038/s41467-019-09982-5
- He S, Feng X. DNA methylation dynamics during germline development. J Integr Plant Biol. 2022;64(12):2240–51. doi: 10.1111/jipb.13422
- Wang L, Zheng K, Zeng L, et al. Reinforcement of CHH methylation through RNA-directed DNA methylation ensures sexual reproduction in rice. Plant Physiol. 2022 ;188(2):1189–1209. doi:10.1093/plphys/kiab531
- Huang J, Wang C, Li X, et al. Conservation and divergence in the Meiocyte sRnaomes of Arabidopsis, soybean, and cucumber. Plant Physiol. 2020;182(1):301–17. doi: 10.1104/pp.19.00807
- Huang J, Wang C, Wang H, et al. Meiocyte-Specific and AtSPO11-1–Dependent Small RNAs and Their Association with Meiotic Gene Expression and Recombination. Plant Cell 2019; 31:444–464. 2 doi: 10.1105/tpc.18.00511
- Wang L, Xu Z, Khawar MB, et al. The histone codes for meiosis. Reproduction. 2017;154(3):R65–R79. doi: 10.1530/REP-17-0153
- Gaysinskaya V, Miller BF, De Luca C, et al. Transient reduction of DNA methylation at the onset of meiosis in male mice. Epigenetics Chromatin 2018; 11:15. 1 doi: 10.1186/s13072-018-0186-0
- Shilo S, Melamed-Bessudo C, Dorone Y, et al. DNA crossover motifs associated with epigenetic modifications delineate open chromatin regions in Arabidopsis. Plant Cell. 2015;27(9):2427–36. doi: 10.1105/tpc.15.00391