ABSTRACT
Drosophila melanogaster expressing amyloid-β42 (Aβ42) transgenes have been used as models to study Alzheimer’s disease. Various Aβ42 transgenes with different structures induce different phenotypes, which make it difficult to compare data among studies which use different transgenic lines. In this study, we compared the phenotypes of four frequently used Aβ42 transgenic lines, UAS-Aβ422X, UAS-Aβ42BL33770, UAS-Aβ4211C39, and UAS-Aβ42H29.3. Among the four transgenic lines, only UAS-Aβ422X has two copies of the upstream activation sequence-amyloid-β42 (UAS-Aβ42) transgene, while remaining three have one copy. UAS-Aβ42BL33770 has the 3′ untranslated region of Drosophila α-tubulin, while the others have that of SV40. UAS-Aβ4211C39 and UAS-Aβ42H29.3 have the rat pre-proenkephalin signal peptide, while UAS-Aβ422X and UAS-Aβ42BL33770 have that of the fly argos protein. When the transgenes were expressed ectopically in the developing eyes of the flies, UAS-Aβ422X transgene resulted in a strongly reduced and rough eye phenotype, while UAS-Aβ42BL33770 only showed a strong rough eye phenotype; UAS-Aβ42H29.3 and UAS-Aβ4211C39 had mild rough eyes. The levels of cell death and reactive oxygen species (ROS) in the eye imaginal discs were consistently the highest in UAS-Aβ422X, followed by UAS-Aβ42BL33770, UAS-Aβ4211C39, and UAS-Aβ42H29.3. Surprisingly, the reduction in survival during the development of these lines did not correlate with cell death or ROS levels. The flies which expressed UAS-Aβ4211C39 or UAS-Aβ42H29.3 experienced greatly reduced survival rates, although low levels of ROS or cell death were detected. Collectively, our results demonstrated that different Drosophila AD models show different phenotypic severity, and suggested that different transgenes may have different modes of cytotoxicity.
Abbreviations: Aβ42: amyloid-β42; AD: Alzheimer’s disease; UAS: upstream activation sequence
Introduction
Alzheimer’s disease (AD) is the most common neurodegenerative disorder and is characterized by amyloid plaques, neurofibrillary tangles, and loss of neurons (Mattson Citation2004). There are several hypotheses to explain the cause of AD (Hardy & Higgins Citation1992; Markesbery Citation1997; Francis et al. Citation1999; Hardy & Selkoe Citation2002; Berridge Citation2010; Maccioni et al. Citation2010). Among them, the amyloid hypothesis states that most of AD pathologies are caused by deposition of amyloid-β42 (Aβ42) peptide, which is generated by proteolytic processing of the amyloid precursor protein (Hardy & Higgins Citation1992).
Based on well-developed genetic tools, such as the upstream activation sequence (UAS)-GAL4 system, by which the expression of desired genes can be regulated, Drosophila has been used as an animal model to study AD (Lee et al. Citation2014, Citation2016; Bang et al. Citation2016). To date, different groups have generated several different transgenic lines that can be used for the ectopic expression of human Aβ42. In the present study, we selected four lines to investigate the relationship between transgene structure and their functions ( and ). UAS-Aβ422X (Casas-Tinto et al. Citation2011) contains two serially concatenated copies of the transgene with an argos signal peptide and SV40 poly A tail (). UAS-Aβ42BL33770 (Singh & Mahoney Citation2011) contains one copy of the transgene with the argos signal peptide and the Drosophila α-tubulin 3′ untranslated region (UTR) (). The α-tubulin 3′ UTR is thought to provide stability to transgenes linked with it (Ollmann et al. Citation2000; Liu et al. Citation2015); it is supposed to increase Aβ42 protein levels by prolonging the RNA half-life. UAS-Aβ4211C39 (Iijima et al. Citation2008) and UAS-Aβ42H29.3 (Finelli et al. Citation2004) both contain a copy of same transgene with a pre-proenkephalin signal peptide and an SV40 poly A tail ().
Figure 1. Constructs in four different UAS-Aβ42 lines. The schematic figures show the constructs in the four UAS-Aβ42 lines, UAS-Aβ422X, UAS-Aβ42BL33770, UAS-Aβ4211C39, and UAS-Aβ42H29.3, which have differences in the number of copies, signal peptides, and poly A tails. UAS-Aβ422X has two copies of the UAS-Aβ42 sequence, while the others have one copy. UAS-Aβ422X and UAS-Aβ42BL33770 have the signal peptide-encoding region of the fly argos gene, whereas UAS-Aβ4211C39 and UAS-Aβ42H29.3 have that of the rat pre-proenkephalin gene. UAS-Aβ42BL33770 carries the poly A tail of α-tubulin, and the others contain that of SV40.
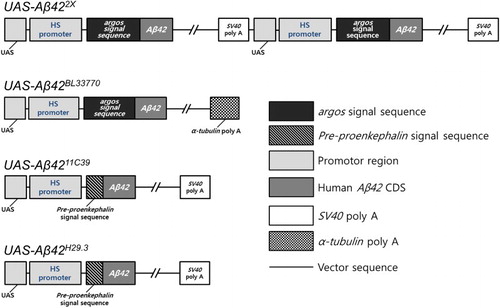
Table 1. The list of studies in that used the UAS-Aβ42 transgenic lines.
Although several Drosophila Aβ42 transgenic lines were developed and used in a variety of studies, their phenotypic differences have not been studied in detail. Therefore, we compared the phenotypes of the four representative UAS-Aβ42 lines under the same experimental conditions. They showed different Aβ42 expression levels and phenotypic severity in eyes and neurons. Interestingly, the level of reactive oxygen species (ROS) generation did not correlate with survival rate in this comparative study.
Materials and methods
Drosophila strains
Glass multimer reporter (GMR)-GAL4 (BL9146), embryonic lethal abnormal vision (elav)-GAL4 (BL458), and UAS-Aβ42BL33770 (BL33770) were acquired from the Bloomington Drosophila Stock Center. UAS-Aβ422X, UAS-Aβ42H29.3, and UAS-Aβ4211C39 were provided by Dr Pedro Fernandez-Funez (University of Florida, USA), Dr Mary Konsolaki (University of Rutgers, USA), and Dr Koichi M. Iijima (University of Thomas Jefferson, USA), respectively.
Thioflavin S staining
Thioflavin S staining was performed as described previously by Iijima et al. (Citation2004). Whole brains were dissected, permeabilized, and incubated overnight at 4°C in 50% ethanol containing 0.125% thioflavin S (Sigma-Aldrich). The samples were rinsed with 50% ethanol and phosphate buffered saline (PBS) containing 0.5% Triton X-100, and examined using confocal microscopy.
Immunohistochemistry
Immunohistochemistry was performed as described previously by Jeong et al. (Citation2015). Whole brains were dissected and blocked with 5% normal goat serum and 2% bovine serum albumin in PBS containing 0.5% Triton X-100. They were incubated for 48 h with anti-Aβ42 antibodies (1:200; Santa Cruz Biotechnology) at 4°C and washed four times with PBS containing 0.5% Triton X-100. Samples were then incubated overnight with Alexa-Fluor-488-labeled anti-mouse antibody (1:200; Invitrogen) at 4°C and washed four times with PBS containing 0.5% Triton X-100.
Acridine Orange staining
Acridine orange (AO) staining was performed as described previously by Hong et al. (Citation2012) and Park et al. (Citation2013). The eye discs of stage L3 larvae were dissected rapidly in PBS and incubated for 5 min with 1.6 × 10−6 M AO (Sigma-Aldrich). After rinsing twice for 5 min in PBS, the samples were analyzed using a fluorescence microscope (Carl Zeiss).
Dihydroethidium staining
For dihydroethidium (DHE) staining, the eye discs of stage L3 larvae were dissected in Schneider’s medium at room temperature, and incubated with Schneider’s medium containing the 3.0 × 10−6 M DHE dye (Invitrogen Molecular Probes) for 5 min in the dark. They were then washed with Schneider’s medium, and observed under a fluorescence microscope (Carl Zeiss).
Analysis of Drosophila development
Fifty embryos of each genotype were collected in vials that contained standard cornmeal media and incubated at 25°C. Survival scores (the ratio of the number of adult male flies raised from collected embryos against half the total number of collected embryos) were obtained for each group. The experiment was repeated six times.
Climbing assay
The climbing assay was performed as previously reported by Hwang et al. (Citation2013) with some modifications. The experiment was conducted with 80 male flies. Ten male flies were collected in each climbing assay vial, and the flies were tapped down to the bottom of the vial. Then, the number of flies that climbed to the top of the vial within 15 s was counted. Ten trials were performed for each group. Climbing scores (the ratio of the number of flies that reached the top against the total number of flies) were obtained for each group, and the mean climbing scores for the 10 repeated tests were compared.
Statistics
In all experiments, data were analyzed using one-way ANOVA followed by a Tukey–Kramer multiple comparison test. Statistical results were exhibited as means ± SEM. Decisive values were expressed by asterisks (*p < .05, **p < .01, and ***p < .001). Eye size was gauged using ImageJ software (National Institutes of Health).
Results
The levels of Aβ42 protein and its aggregates in the developing eyes and brains of Drosophila AD models
To characterize the four different Aβ42 transgenic lines, we measured the levels of Aβ42 aggregates and its protein abundance. As expected, the Aβ42 protein and its aggregation level in both the developing eyes and brain were the highest in the UAS-Aβ422X line (), which contains two copies of the Aβ42 transgene (). The second highest was the UAS-Aβ42BL33770 line (), which has an argos signal peptide and poly A tail of fly α-tubulin (). The expression level of Aβ42 protein by the UAS-Aβ4211C39 line was higher than that of UAS-Aβ42H29.3 (), despite having Aβ42 transgenes with the same structure (), which suggested that their difference might be caused by a position effect.
Figure 2. The Aβ42 levels and extent of aggregation in the eye imaginal discs (a–c) and the adult brains (d–f) of the flies expressing different Aβ42 transgenes. Representative images of thioflavin S staining (a, b, d, e) and Aβ42-immunostaining (c, f) in eye imaginal discs and adult brains. (b) and (c) correspond to the dotted area in (a), while (e) and (f) correspond to the dotted area in (d). The human Aβ42 transgenes were expressed in Drosophila eye imaginal discs at 29°C and neurons at 25°C, respectively. Magnification of the pictures: (a) ×200, (b, c, e, f) ×400, and (d) ×100.
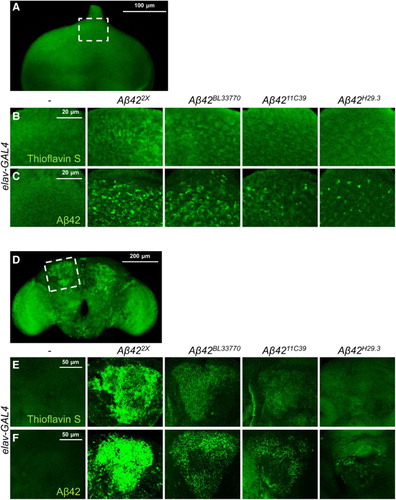
We also measured Aβ42 aggregate levels by thioflavin S staining, which is a commonly used method to detect amyloid fibrils, but not monomers (Yamamoto & Hirano Citation1986). The levels of Aβ42 aggregates were proportional to the protein levels (), which indicated that the aggregation properties of the protein produced by the transgenes were similar.
The levels of cell death induced by the four different Aβ42 transgenes
Next, we examined the cell death induced by the transgenes in developing eyes, which have been used frequently to measure cell death (Lee et al. Citation2014). Ectopic Aβ42 expression resulted in severely reduced and rough eyes in the UAS-Aβ422X lines when reared at both 25°C and 29°C ((a)–(d)). However, the eye phenotype of flies expressing the UAS-Aβ42BL33770 transgene depended on the rearing temperature. The reduced and rough eye phenotype appeared only at 29°C, while the rough eye phenotype without size reduction appeared at 25°C ((a)–(d)). The flies expressing UAS-Aβ4211C39 and UAS-Aβ42H29.3 showed very mild rough eye phenotype at 29°C ((b)). The number of dead cells in the developing eyes was consistently the highest in UAS-Aβ422X, followed by UAS-Aβ42BL33770, UAS-Aβ4211C39, and UAS-Aβ42H29.3 ((e) and 3(f)).
Figure 3. The morphology and cell death of Drosophila eyes expressing four Aβ42 transgenes. (a, b) Pictures showing the eyes of flies expressing different Aβ42 transgenes at different temperatures. Magnification of the pictures, ×50. (c, d) Graphs showing the relative sizes of the eyes of each experimental group (Tukey–Kramer test, n ≥ 19, ***p < .001, NS, not significant). (e) Fluorescent microscopic images of AO-stained eye imaginal discs expressing human Aβ42 using four different transgenic lines at 29°C. Magnification of the pictures, ×200. (f) A graph showing the relative number of AO-positive signals in the eye imaginal disc of each experimental group (Tukey–Kramer test, n ≥ 17, ***p < .001).
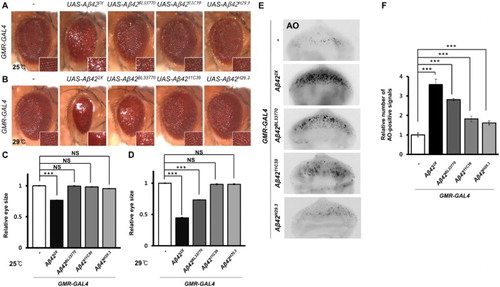
The levels of ROS in the flies expressing the four different Aβ42 transgenes
ROS generation is an important pathological characteristic of AD, and ROS is closely associated with neuronal cell death (Markesbery Citation1997); therefore, we also measured the ROS levels using DHE staining in the eye imaginal discs expressing the Aβ42 transgenes. A prominent amount of ROS was detected in the eye imaginal discs expressing UAS-Aβ422X and UAS-Aβ42BL33770, while little was observed in the discs expressing UAS-Aβ4211C39 and UAS-Aβ42H29.3 ().
Figure 4. The ROS levels in the flies expressing four different Aβ42 transgenes. (a) Fluorescent microscopic images of DHE-stained eye imaginal discs expressing human Aβ42 using four different transgenic lines at 29°C. Magnification of the pictures, ×200. (b) A graph showing ROS levels, which were detected by DHE staining (Tukey–Kramer test, n ≥ 18, **p < .01, ***p < .001; NS, not significant).
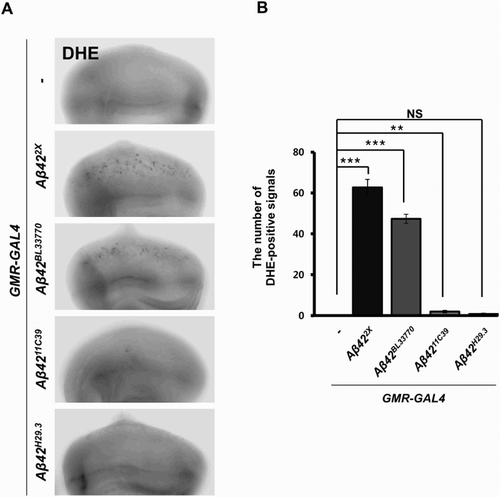
The phenotypes of the flies expressing the Aβ42 transgenes in neurons
We also examined the effects of transgene expression in neurons during development by calculating the survival rates, which were the ratio of emerged adults from eggs. Interestingly, the trend of decreased survival in each Aβ42-expressing line was different from the levels of Aβ42 expression or the eye phenotype ((a)). The survival rate of the UAS-Aβ42BL33770 flies was the lowest, while the UAS-Aβ4211C39 and UAS-Aβ42H29.3 flies also showed significantly reduced survival ((a)).
Figure 5. Survival rates and climbing ability of neuronal Aβ42-expressing flies with four different Aβ42 transgenes. (a) A graph showing the survival rates of Drosophila expressing human Aβ42 in their brains using four different transgenic lines at 25°C (Tukey–Kramer test, n ≥ 180, ***p < .001). (b) A graph showing the climbing ability of Aβ42-expressing flies at 25°C (Tukey–Kramer test, n ≥ 80, *p < .05, ***p < .001).
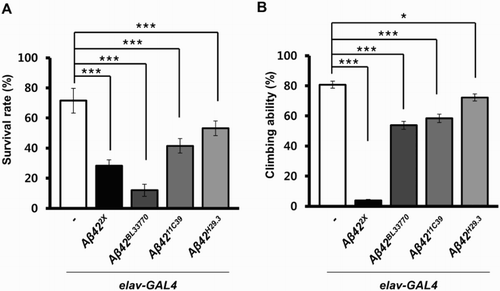
To compare the effects of Aβ42 expression on adult neurological function, the locomotor activities of the flies expressing the transgenes were measured. Surprisingly, the trend in the locomotor dysfunction levels in the UAS-Aβ422X and UAS-Aβ42BL33770 lines was quite different from that of their survival rates ((b)). Although the survival rate of UAS-Aβ42BL33770 flies was extremely low (12%), upon emerging, they only showed a moderate locomotor defect ((b)), which suggested that the surviving flies may be relatively healthy.
Discussion
In this study, we compared the expression levels of Aβ42 and the phenotypes of flies expressing four frequently used UAS-Aβ42 transgenes. The relative expression levels of Aβ42 in the transgenic lines are similar in both the developing eyes and brain. Both the Aβ42 proteins and its aggregation levels were consistently the highest in the developing eyes and brain of UAS-Aβ422X line, followed by UAS-Aβ42BL33770, UAS-Aβ4211C39, and UAS-Aβ42H29.3. However, the effects of Aβ42 expression on the phenotypes in these lines were different in these tissues. The eyes of flies expressing UAS-Aβ422X or UAS-Aβ42BL33770 showed severe defects, while UAS-Aβ4211C39 or UAS-Aβ42H29.3 flies had very mild rough eye phenotypes, which correlated with Aβ42 protein levels. In contrast, the severity of neuronal phenotypes in each transgenic line did not correlate with Aβ42 protein levels. When the transgenes were expressed pan-neuronally using the elav-GAL4 driver, the survival rate was reduced significantly in both UAS-Aβ4211C39 and UAS-Aβ42H29.3, unlike their eye phenotypes. This discrepancy in the effects of Aβ42 in the different tissues might be caused by the difference in susceptibility between neurons and non-neuronal cells. In support of this notion, a previous study showed that Aβ oligomer administration induced cell death in primary cultures of rat cortical neurons, but not in astrocytes (Ebenezer et al. Citation2010). The hypersensitivity of neuronal cells to Aβ oligomers might reflect the high level of Aβ oligomer receptors, such as the receptor for advanced glycation end products (Du Yan et al. Citation1996) and prions (Laurén et al. Citation2009), or erroneous cell cycle activation by the Aβ protein in neurons (Caricasole et al. Citation2003). Although the detailed mechanism is not clear, our data suggest that the Aβ hypersensitivity of neuronal cells is conserved in Drosophila.
We also found that the survival rate of the flies expressing UAS-Aβ42BL33770 in neurons was the lowest, while Aβ42 expression levels of these flies are much lower than that of flies expressing UAS-Aβ422X transgene. This result suggests that the neurotoxicity of Aβ42 is not simply determined by Aβ42 levels. This phenomenon is also well known in human brain. That is, the degree of cognitive impairment in AD patients does not correlate well with the brain Aβ deposits number (Hardy & Selkoe Citation2002). However, the soluble Aβ concentrations were inversely correlated with synapse loss in AD patients and distinguished AD patients from high pathology control patients (Lue et al. Citation1999), which suggests that soluble Aβ42 oligomers, but not insoluble Aβ42 deposits, are responsible for AD pathology such as synapse loss. Therefore, the unexpected highly decreased survival rate of Aβ42BL33770-expressing flies would be the result from the high level of soluble Aβ42 oligomer generation in this line.
The difference between the constructs of the transgenes in different UAS-Aβ42 lines might also be associated with their phenotypic variation. The different secretory abilities of the Aβ42 peptide expressed from each transgenic line might explain the unexpected strong reduction of survival during the development of flies expressing UAS-Aβ4211C39 or UAS-Aβ42H29.3 in neurons. As these lines contain a mammalian signal peptide, Aβ42 proteins might be secreted less efficiently in these lines compared to UAS-Aβ422X and UAS-Aβ42BL33770 lines, which contain a Drosophila signal peptide. In that case, flies with UAS-Aβ4211C39 and UAS-Aβ42H29.3 might secrete little Aβ42 out of the cells, resulting in intracellular Aβ42 accumulation that would damage mitochondria. In contrast, the UAS-Aβ422X and UAS-Aβ42BL33770 lines secreted most of the Aβ42 proteins outside the cells, while relatively little accumulates in the cytoplasm. Further studies on the Aβ42 secretion for each transgenic line are needed to reveal the detailed mechanism of Aβ42 cytotoxicity.
In addition, the effect of different genetic backgrounds should be considered. Although we used the same GAL4 lines to express the four different Aβ42 transgenes ectopically, the transgenic lines have different genetic backgrounds, which could affect the phenotypes produced by the transgenes. Therefore, to exclude this possibility completely, further studies should be conducted with the new transgenes with clear genetic backgrounds, which can be achieved by backcrossing to the same control line, such as w1118.
In conclusion, our data demonstrate that different Drosophila AD models show different phenotypic severity in different tissues, and suggest that different Aβ42 transgenes might have different modes of cytotoxicity. Therefore, AD models should be designed for the specific aims of each study.
Disclosure statement
No potential conflict of interest was reported by the authors.
Additional information
Funding
References
- Ambegaokar SS, Jackson GR. 2011. Functional genomic screen and network analysis reveal novel modifiers of tauopathy dissociated from tau phosphorylation. Hum Mol Genet. 20:4947–4977. doi: 10.1093/hmg/ddr432
- Ando K, Maruko-Otake A, Ohtake Y, Hayashishita M, Sekiya M, Iijima KM. 2016. Stabilization of microtubule-unbound Tau via Tau phosphorylation at Ser262/356 by Par-1/MARK contributes to augmentation of AD-related phosphorylation and Aβ42-induced Tau toxicity. PLoS Genet. 12:e1005917. doi: 10.1371/journal.pgen.1005917
- Bang SM, Lee S, Jeong H, Hong YK, Lee JH, Hwang S, Suh YS, Lee K, Cho KS. 2016. Effects of sarah/nebula knockdown on Aβ42-induced phenotypes during Drosophila development. Genes Genomics. 38:479–487. doi: 10.1007/s13258-016-0407-5
- Berridge MJ. 2010. Calcium hypothesis of Alzheimer’s disease. Pflugers Arch. 459:441–449. doi: 10.1007/s00424-009-0736-1
- Cao W, Song HJ, Gangi T, Kelkar A, Antani I, Garza D, Konsolaki M. 2008. Identification of novel genes that modify phenotypes induced by Alzheimer’s β-amyloid overexpression in Drosophila. Genetics. 178:1457–1471. doi: 10.1534/genetics.107.078394
- Caricasole A, Copani A, Caruso A, Caraci F, Iacovelli L, Sortino MA, Terstappen GC, Nicoletti F. 2003. The Wnt pathway, cell-cycle activation and β-amyloid: novel therapeutic strategies in Alzheimer’s disease? Trends Pharmacol Sci. 24:233–238. doi: 10.1016/S0165-6147(03)00100-7
- Casas-Tinto S, Zhang Y, Sanchez-Garcia J, Gomez-Velazquez M, Rincon-Limas DE, Fernandez-Funez P. 2011. The ER stress factor XBP1s prevents amyloid-β neurotoxicity. Hum Mol Genet. 20:2144–2160. doi: 10.1093/hmg/ddr100
- Chiang HC, Iijima K, Hakker I, Zhong Y. 2009. Distinctive roles of different β-amyloid 42 aggregates in modulation of synaptic functions. FASEB J. 23:1969–1977. doi: 10.1096/fj.08-121152
- Chiang HC, Wang L, Xie Z, Yau A, Zhong Y. 2010. PI3 kinase signaling is involved in Aβ-induced memory loss in Drosophila. Proc Natl Acad Sci U S A. 107:7060–7065. doi: 10.1073/pnas.0909314107
- Chouhan AK, Guo C, Hsieh YC, Ye H, Senturk M, Zuo Z, Li Y, Chatterjee S, Botas J, Jackson GR, et al. 2016. Uncoupling neuronal death and dysfunction in Drosophila models of neurodegenerative disease. Acta Neuropathol Commun. 4:62. doi: 10.1186/s40478-016-0333-4
- Du Yan S, Chen X, Fu J, Chen M, Zhu H, Roher A, Slattery T, Zhao L, Nagashima M, Morser J, et al. 1996. RAGE and amyloid-β peptide neurotoxicity in Alzheimer’s disease. Nature. 382:685–691. doi: 10.1038/382685a0
- Ebenezer PJ, Weidner AM, LeVine H, Markesbery WR, Murphy MP, Zhang L, Dasuri K, Fernandez-Kim SO, Bruce-Keller AJ, Gavilan E, et al. 2010. Neuron specific toxicity of oligomeric amyloid-beta: role for JUN-kinase and oxidative stress. J Alzheimers Dis. 22:839–848. doi: 10.3233/JAD-2010-101161
- Fernandez-Funez P, Zhang Y, Sanchez-Garcia J, de Mena L, Khare S, Golde TE, Levites Y, Rincon-Limas DE. 2015. Anti-Aβ single-chain variable fragment antibodies exert synergistic neuroprotective activities in Drosophila models of Alzheimer’s disease. Hum Mol Genet. 24:6093–6105. doi: 10.1093/hmg/ddv321
- Finelli A, Kelkar A, Song H-J, Yang H, Konsolaki M. 2004. A model for studying Alzheimer’s Aβ42-induced toxicity in Drosophila melanogaster. Mol Cell Neurosci. 26:365–375. doi: 10.1016/j.mcn.2004.03.001
- Francis PT, Palmer AM, Snape M, Wilcock GK. 1999. The cholinergic hypothesis of Alzheimer’s disease: a review of progress. J Neurol Neurosurg Psychiatr. 66:137–147. doi: 10.1136/jnnp.66.2.137
- Gerstner JR, Lenz O, Vanderheyden WM, Chan MT, Pfeiffenberger C, Pack AI. 2016. Amyloid-β induces sleep fragmentation that is rescued by fatty acid binding proteins in Drosophila. J Neurosci Res. [Epub ahead of print] doi:10.1002/jnr.23778.
- Hardy J, Selkoe DJ. 2002. The amyloid hypothesis of Alzheimer’s disease: progress and problems on the road to therapeutics. Science. 297:353–356. doi: 10.1126/science.1072994
- Hardy JA, Higgins GA. 1992. Alzheimer's disease: the amyloid cascade hypothesis. Science. 256:184–185. doi: 10.1126/science.1566067
- Hong YK, Lee S, Park SH, Lee JH, Han SY, Kim ST, Kim Y-K, Jeon S, Koo B-S, Cho KS. 2012. Inhibition of JNK/dFOXO pathway and caspases rescues neurological impairments in Drosophila Alzheimer’s disease model. Biochem Biophys Res Commun. 419:49–53. doi: 10.1016/j.bbrc.2012.01.122
- Hwang S, Song S, Hong YK, Choi G, Suh YS, Han SY, Lee M, Park SH, Lee JH, Lee S, et al. 2013. Drosophila DJ-1 decreases neural sensitivity to stress by negatively regulating Daxx-like protein through dFOXO. PLoS Genet. 9:e1003412. doi: 10.1371/journal.pgen.1003412
- Iijima-Ando K, Hearn SA, Granger L, Shenton C, Gatt A, Chiang H-C, Hakker I, Zhong Y, Iijima K. 2008. Overexpression of neprilysin reduces Alzheimer amyloid-β42 (Aβ42)-induced neuron loss and intraneuronal Aβ42 deposits but causes a reduction in cAMP-responsive element-binding protein-mediated transcription, age-dependent axon pathology, and premature death in Drosophila. J Biol Chem. 283:19066–19076. doi: 10.1074/jbc.M710509200
- Iijima K, Chiang H-C, Hearn SA, Hakker I, Gatt A, Shenton C, Granger L, Leung A, Iijima-Ando K, Zhong Y. 2008. Aβ42 mutants with different aggregation profiles induce distinct pathologies in Drosophila. PloS One. 3:e1703. doi: 10.1371/journal.pone.0001703
- Iijima K, Gatt A, Iijima-Ando K. 2010. Tau Ser262 phosphorylation is critical for Aβ42-induced tau toxicity in a transgenic Drosophila model of Alzheimer’s disease. Hum Mol Genet. 19:2947–2957. doi: 10.1093/hmg/ddq200
- Iijima K, Liu HP, Chiang AS, Hearn SA, Konsolaki M, Zhong Y. 2004. Dissecting the pathological effects of human Abeta40 and Abeta42 in Drosophila: a potential model for Alzheimer’s disease. Proc Natl Acad Sci U S A. 101:6623–6628. doi: 10.1073/pnas.0400895101
- Jeong H, Han SY, Lee M, Lee S, Shin M, Jeon Y, Lee K, Cho KS. 2015. Roles of Tsp66E and Tsp74F in border cell migration and the maintenance of border cell adhesion in Drosophila. Genes Genomics. 37:559–565. doi: 10.1007/s13258-015-0285-2
- Lang M, Fan Q, Wang L, Zheng Y, Xiao G, Wang X, Wang W, Zhong Y, B Z. 2013. Inhibition of human high-affinity copper importer Ctr1 orthologous in the nervous system of Drosophila ameliorates Abeta42-induced Alzheimer’s disease-like symptoms. Neurobiol Aging. 34:2604–2612. doi: 10.1016/j.neurobiolaging.2013.05.029
- Lang M, Wang L, Fan Q, Xiao G, Wang X, Zhong Y, Zhou B. 2012. Genetic inhibition of solute-linked carrier 39 family transporter 1 ameliorates aβ pathology in a Drosophila model of Alzheimer’s disease. PLoS Genet. 8:e1002683. doi: 10.1371/journal.pgen.1002683
- Laurén J, Gimbel DA, Nygaard HB, Gilbert JW, Strittmatter SM. 2009. Cellular prion protein mediates impairment of synaptic plasticity by amyloid-beta oligomers. Nature. 457:1128–1132. doi: 10.1038/nature07761
- Lee MJ, Park SH, Han JH, Hong YK, Hwang S, Lee S, Kim D, Han SY, Kim ES, Cho KS. 2011. The effects of hempseed meal intake and linoleic acid on Drosophila models of neurodegenerative diseases and hypercholesterolemia. Mol Cells. 31:337–342. doi: 10.1007/s10059-011-0042-6
- Lee S, Bang SM, Hong YK, Lee JH, Jeong H, Park SH, Liu QF, Lee IS, Cho KS. 2016. The calcineurin inhibitor sarah (nebula) exacerbates Abeta42 phenotypes in a Drosophila model of Alzheimer’s disease. Dis Model Mech. 9:295–306. doi: 10.1242/dmm.018069
- Lee S, Bang SM, Lee JW, Cho KS. 2014. Evaluation of traditional medicines for neurodegenerative diseases using Drosophila models. Evid Based Complement Alternat Med. eCAM.2014:967462.
- Lee S, Wang JW, Yu W, Lu B. 2012. Phospho-dependent ubiquitination and degradation of PAR-1 regulates synaptic morphology and tau-mediated Aβ toxicity in Drosophila. Nat Commun. 3:1312. doi: 10.1038/ncomms2278
- Lin R, Angelin A, Da Settimo F, Martini C, Taliani S, Zhu S, Wallace DC. 2014. Genetic analysis of dTSPO, an outer mitochondrial membrane protein, reveals its functions in apoptosis, longevity, and Aβ42-induced neurodegeneration. Aging Cell. 13:507–518. doi: 10.1111/acel.12200
- Ling D, Salvaterra PM. 2011. Brain aging and Aβ1–42 neurotoxicity converge via deterioration in autophagy–lysosomal system: a conditional Drosophila model linking Alzheimer’s neurodegeneration with aging. Acta Neuropathol. 121:183–191. doi: 10.1007/s00401-010-0772-0
- Ling D, Song H-J, Garza D, Neufeld TP, Salvaterra PM. 2009. Abeta42-induced neurodegeneration via an age-dependent autophagic-lysosomal injury in Drosophila. PLoS One. 4:e4201. doi: 10.1371/journal.pone.0004201
- Liu QF, Jeong H, Lee JH, Hong YK, Oh Y, Kim YM, Suh YS, Bang S, Yun HS, Lee K, et al. 2016. Coriandrum sativum suppresses Aβ42-induced ROS increases, glial cell proliferation, and ERK activation. Am J Chin Med. 44:1325–1347. doi: 10.1142/S0192415X16500749
- Liu QF, Lee JH, Kim Y-M, Lee S, Hong YK, Hwang S, Oh Y, Lee K, Yun HS, Lee IS, et al. 2015. In vivo screening of traditional medicinal plants for neuroprotective activity against Aβ42 cytotoxicity by using Drosophila models of Alzheimer’s disease. Biol Pharm Bull. 38:1891–1901. doi: 10.1248/bpb.b15-00459
- Lüchtenborg AM, Katanaev VL. 2014. Lack of evidence of the interaction of the Aβ peptide with the Wnt signaling cascade in Drosophila models of Alzheimer’s disease. Mol Brain. 7:81.
- Lue LF, Kuo YM, Roher AE, Brachova L, Shen Y, Sue L, Beach T, Kurth JH, Rydel RE, Rogers J. 1999. Soluble amyloid β peptide concentration as a predictor of synaptic change in Alzheimer’s disease. Am J Pathol. 155:853–862. doi: 10.1016/S0002-9440(10)65184-X
- Maccioni RB, Farias G, Morales I, Navarrete L. 2010. The revitalized tau hypothesis on Alzheimer’s disease. Arch Med Res. 41:226–231. doi: 10.1016/j.arcmed.2010.03.007
- Markesbery WR. 1997. Oxidative stress hypothesis in Alzheimer’s disease. Free Radic Biol Med. 23:134–147. doi: 10.1016/S0891-5849(96)00629-6
- Mattson MP. 2004. Pathways towards and away from Alzheimer’s disease. Nature. 430:631–639. doi: 10.1038/nature02621
- Ollmann M, Young LM, Di Como CJ, Karim F, Belvin M, Robertson S, Whittaker K, Demsky M, Fisher WW, Buchman A, et al. 2000. Drosophila p53 is a structural and functional homolog of the tumor suppressor p53. Cell. 101:91–101. doi: 10.1016/S0092-8674(00)80626-1
- Park SH, Lee S, Hong YK, Hwang S, Lee JH, Bang SM, Kim YK, Koo BS, Lee IS, Cho KS. 2013. Suppressive effects of SuHeXiang Wan on amyloid-β42-induced extracellular signal-regulated kinase hyperactivation and glial cell proliferation in a transgenic Drosophila model of Alzheimer’s disease. Biol Pharm Bull. 36:390–398. doi: 10.1248/bpb.b12-00792
- Sanokawa-Akakura R, Cao W, Allan K, Patel K, Ganesh A, Heiman G, Burke R, Kemp FW, Bogden JD, Camakaris J, et al. 2010. Control of Alzheimer’s amyloid beta toxicity by the high molecular weight immunophilin FKBP52 and copper homeostasis in Drosophila. PLoS One. 5:e8626. doi: 10.1371/journal.pone.0008626
- Singh C, Mahoney M. 2011. UAS-APP and APP-based constructs and insertions from Vitruvean. Flybase. Personal communication to Flybase:FBrf0213105.
- Wang L, Chiang HC, Wu W, Liang B, Xie Z, Yao X, Ma W, Du S, Zhong Y. 2012. Epidermal growth factor receptor is a preferred target for treating amyloid-β-induced memory loss. Proc Natl Acad Sci U S A. 109:16743–16748. doi: 10.1073/pnas.1208011109
- Yamamoto T, Hirano A. 1986. A comparative study of modified Bielschowsky, Bodian and thioflavin S stains on Alzheimer’s neurofibrillary tangles. Neuropathol Appl Neurobiol. 12:3–9. doi: 10.1111/j.1365-2990.1986.tb00677.x