ABSTRACT
The rapid increase in carbon dioxide levels in seawater is causing ocean acidification and is expected to have significant effects on marine life. To explore the ability of the harpacticoid copepod Tigriopus japonicus to adapt to an increased concentration of dissolved carbon dioxide (CO2) in seawater, we compared the survival rates of adult and nauplius stages at 400, 1000, and 1550 ppm pCO2 over a 14-day period. The survival rate of T. japonicus dramatically decreased over time with increase in pCO2 concentration. At 1550 ppm, the survival rate showed a decrease of more than 20% at the end of the experimental period over that at 400 ppm. Furthermore, the survival rate decreased by a greater amount at all concentrations in nauplii than in adults, with a greater effect in wild-collected specimens than in culture-derived individuals. The results suggest that future ocean acidification may negatively influence the sustainability of T. japonicus and thus may eventually influence benthic ecosystems.
Introduction
In the 200 years since the industrial revolution, the concentration of carbon dioxide (CO2) in the atmosphere has increased from 280 to 380 ppm due to human activity (Feely et al. Citation2004). The recent climate change report by the IPCC predicted that, at the current rate of increase in CO2 concentration in the atmosphere, the amount of CO2 in the ocean will increase to 851–1370 ppm by 2100, and to 1371–2900 ppm by 2150 (IPCC Citation2014).
The increase in the concentration of CO2 dissolved in the ocean will induce carbonate ion deficiency, which will reduce the productivity and survival of marine organisms that have calcium carbonate shells and frames (Gattuso et al. Citation1998; Riebesell et al. Citation2000) and also have an adverse effect on physiological functions such as development (Fabry et al. Citation2008), metabolism (Pörtner Citation2008), ion adjustment (Pörtner et al. Citation2004), and acid–base equilibrium (Widdicombe & Spicer Citation2008).
A number of studies have examined the effects of ocean acidification on marine life. However, most studies on benthic invertebrates have focused on macrobenthic organisms such as abalone (Kim et al. Citation2013), clams (Lee & Kim Citation2016), mussels, hermit crabs (Kim et al. Citation2016; Kim & Barry Citation2016), and sea urchins (Sung et al. Citation2014). Smaller benthic organisms such as the meiofauna are less well studied (Dupont & Thorndyke Citation2009; Przeslawski et al. Citation2009). Additionally, many of the studies on meiofauna addressed the effect of high concentrations of CO2 (>5000 ppm) in relation to CO2 capture and storage projects (Barry et al. Citation2005; Thistle et al. Citation2006; Pascal et al. Citation2010; Kita et al. Citation2013); there have been no studies on the effects of long-term exposure to relatively low CO2 concentrations (<1600 ppm).
Harpacticoid copepods form one of the dominant taxa in the meiobenthos along with nematodes, and are present in a range of habitats from the deep-ocean to coastal areas. They have a very important role in the food chain of coastal ecosystems. Harpacticoid copepods can be used as indicator species because they are very sensitive to environmental changes and pollution, and thus serve as an important tool for environmental assessment (Ito Citation1970; Kusk & Wollenberger Citation1999). The responses of harpacticoid copepods to environmental changes differ among life cycle stages; thus, both adult and nauplius stages need to be examined in experimental studies (Forget et al. Citation1998). Tigriopus japonicus is a harpacticoid copepod species that inhabits the coastal intertidal zone; it is present in large numbers at a site and can easily be cultured in the laboratory. As it is sensitive to environmental changes, T. japonicus is often used as an experimental organism (Lee Citation1991; Barka et al. Citation2001; Cao et al. Citation2015).
The purpose of the present study was to determine the effects of ocean acidification on T. japonicus and examine whether these effects differ among growth stages and environments. We determined changes in survival rates in adults and nauplii of T. japonicas which have acclimated to the different growth environments after exposure to increased seawater CO2 concentrations.
Materials and methods
Test organism
The experiments were carried out using two groups of T. japonicus, namely laboratory cultured copepods and wild-caught copepods. The former were provided by the copepod culture laboratory of Gangneung-Wonju National University, Republic of Korea in May 2012, and maintained in an incubator at a constant temperature (20°C) for one week. Seawater was used as the culture medium after filtration through 1.2 µm pore size GF/C filter paper. The photoperiod was adjusted to 12 h light:12 h dark and the salinity of the seawater was maintained at 28.0–30.0 psu. The T. japonicus were fed a mixture of green algae (Tetraselmis suecica) and haptophyta (Isochrysis galbana) in a ratio of 1:1; each copepod was supplied with 3 µL of the mixture once per day during the culture period. The wild-caught copepods were obtained from an intertidal zone on the eastern coast of Hupo harbor in Uljin in May 2012; they were acclimatized to a salinity concentration of 30.0 psu and temperature of 20°C for one day.
At the end of the experiment, wild-caught organisms were fixed in 70% ethanol; their identity was confirmed by dissection and analysis under a dissection microscope (Leica MZ16) and optical microscope (Olympus BX51).
CO2 supply and pCO2 control
For the experiment, seawater at a constant pCO2 concentration was supplied using the AICAL system, which is a high-accuracy CO2 automatic manipulation system (Fujita et al. Citation2011; Suwa et al. Citation2013). This system includes CO2 dissolution and measurement towers, which facilitate the effective dissolution of gaseous CO2 into seawater and the continuous logging of pCO2 levels and water temperature in the generated seawater. The desired levels of pCO2 gas were generated by blending pure CO2 gas with CO2-reduced air in a pCO2-regulation system (CGM-07 and DGG-07; Kimoto Electric, Osaka, Japan), which is part of the AICAL system. The pCO2 levels were logged every hour using a pCO2 control/monitoring system (CO2-07; Kimoto Electric). A range of pCO2 concentration was set from 400 ppm, which is the current average CO2 concentration in the atmosphere, to 1550 ppm, which is one of five predicted CO2 concentration values by the year 2100 (IPCC Citation2007). Three CO2 treatments were set: 400, 1000, and 1550 ppm. The pCO2 was monitored each hour during the experimental period. The average pCO2 concentration was 401.2 ± 7.9 (standard deviation) ppm for treatment 1 (control group), 999.3 ± 12.7 ppm for treatment 2, and 1553.7 ± 15.0 ppm for treatment 3 (, ).
Table 1. pCO2, pH, salinity, and temperature measured in each treatment during the experiment.
Experimental chamber
T. japonicus was exposed to the seawater of each experimental treatment in an experimental chamber. The chamber had an open top, a height of 4 cm, and a cylindrical diameter of 2.5 cm; the open top allowed quick observation of surviving and dead individuals through a dissection microscope. The chamber was constructed of transparent acrylic plastic and had a volume of 10 ml. A hole (13 mm diameter) was drilled into the bottom of the chamber, and two holes (10 mm diameter) were made in the sides of the chamber (). Each hole was covered by a protective mesh screen (pore size 63 µm) to ensure that the experimental organisms remained within the chamber. Each chamber was linked by a hose to the AICAL system and sweater was pumped continuously through the system at a constant rate of 300 ml/min. The seawater entered the chamber through the hole in the bottom and exited through the two side holes. Three chambers were prepared for each experimental treatment.
Survival rate measurements
The survival of adults and nauplii at each pCO2 concentration was examined under the same salinity, temperature, and photoperiod conditions as in the cultures. For the adult group, food was supplied in the same manner as in the cultures. Surviving nauplii grew throughout the experimental period; thus, the amount of food supplied differed at different growth stages, with 0.5 μL supplied to nauplii, 1 μL to copepodites, and 3 μL to adults.
To accurately identify complete adult individuals, brooding female were used. On the other hand, to obtain nauplius stage individuals, 30 brooding females were placed into a 6-well culture plate and stage 1 nauplii were collected and used. Five nauplii were placed in each chamber, and the experiment was carried out for 14 days. To reduce experimental errors, hatching nauplii from brooding females were removed from the adult chamber every 12 h. Measurements were made every 24 h for each concentration, counting dead and surviving individuals and converting these numbers to survival rates.
Statistical analysis
Significant differences in survival rates among the treatment groups with different pCO2 concentrations were tested using repeated-measures ANOVA with the SPSS program (IBM SPSS statistics 19.0, SPSS Inc., IBM company). If there was a significant difference in the repeated-measures ANOVA test, Tukey’s HSD (honestly significant differences) test was performed as a post-hoc test for the multiple comparison analysis.
Results
The survival rate did not differ significantly among treatments for adults of the culture groups (F = 0.86, df = 2, P > .05, (a)). However, in the nauplius group, the survival rate at 400 ppm (control) was significantly higher than that at 1550 ppm (F = 4.568, df = 1, P < .05, Tukey post hoc test, P < .05, (b)).
Figure 3. Changes in the survival rates of adult (a) and nauplius (b) of the culture-derived T. japonicus exposed to seawater with different pCO2 for 14 days.
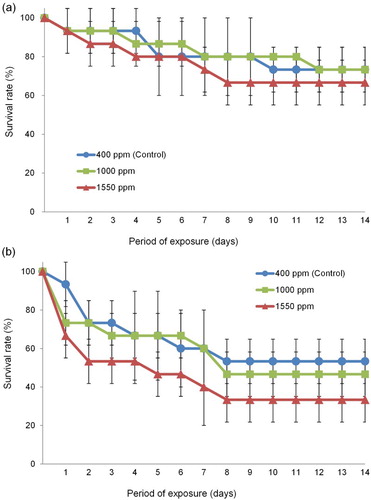
Significant variation in survival rates was found among wild-caught T. japonicus after 14 days exposure to different pCO2 levels (). Survival rates of both adults and nauplii were significantly lower at 1550 ppm than in the control (adult: F = 4.568, df = 1, P < .05, (a); nauplius: F = 7.636, df = 1, P < .05, (b)).
Discussion
The survival rate of T. japonicus was significantly lower at the highest pCO2 concentration tested here, indicating that pCO2 concentrations negatively affected the organisms. This result suggests that drastic environmental changes such as ocean acidification induce distinct changes to physiological functions in a harpacticoid copepod species that plays a role as a major secondary producer in coastal benthic ecosystems. Marine organisms may use the acid–base equilibrium for acidity control in the body, or limit reproduction and growth for long-term preservation of species (Langenbuch & Pörtner Citation2004). It has been reported that egg production in copepods is reduced when the organisms are exposed to CO2-induced acidified seawater over multiple generations (Mayor et al. Citation2007; Weydmann et al. Citation2012; Hildebrandt et al. Citation2014). The calanoid copepod Acartia tonsa showed delayed growth when exposed to a pCO2 of 1000 µatm that resulted in pH 7.8 (Cripps et al. Citation2014). In addition, when deep-sea sites are temporarily acidified by exposure to high CO2 concentrations, different species of harpacticoid copepod vary in their tolerance to the change (Barry et al. Citation2005; Thistle et al. Citation2006). Although there have been many studies on the effects of ocean acidification on copepods, particularly benthic harpacticoid copepods, in different regions in recent years, the amount of information available on their responses is still insufficient and the effects are not clearly defined.
This study showed that there was a significant difference in the survival rates of adult and nauplius T. japonicus exposed to 400 ppm from that in T. japonicus 1550 ppm (P < .05). Our results suggest that survival of T. japonicus is reduced at a CO2 concentration of 1550 ppm or more (less than pH 7.6). The mechanisms that underlie this difference in survival rate are currently unknown.
In this study, the survival rates of T. japonicus nauplii showed greater changes with CO2 concentration than did those of adult stages. The nauplius survival rate at 1550 ppm decreased dramatically to half that of the control in the first 2 days of the experiment in both culture-derived and wild-caught groups. The harpacticoid copepod species T. japonicus is widely used in toxicity assessment experiments (McAllen et al. Citation1999; Barka et al. Citation2001; McAllen & Taylor Citation2001). These copepods undergo several molts and one metamorphosis during the transformation into an adult after hatching. During growth, the morphological, physiological, and behavioral characteristics between each growth stage show major differences. Accordingly, experiments that include a range of growth stages are considered essential in analyses of the effects of environmental changes of pollutants (Pounds et al. Citation2002; Fitzer et al. Citation2012; Cao et al. Citation2015). Our findings here are consistent with those of previous studies. Nauplii of the harpacticoid copepod Amphiascus tenuiremis were shown to be 28 times more sensitive in terms of survival rate than adult individuals to exposure to the organophosphate pesticide chlorpyrifos (Green et al. Citation1996), while males of the copepod A. tonsa had a two-fold higher rate of reaction to toxicity than females (Barata et al. Citation2002). Moreover, early development stages showed more sensitivity to pollutants (Green et al. Citation1996; Lotufo Citation1997). Copepods at early developmental stages showed more sensitivity to CO2 storage in deep-sea sites (Pörtner & Farrell Citation2008), and invertebrates were especially affected at early development stages (Mayor et al. Citation2007; Dupont & Thorndyke Citation2009). In the current work, culture-derived and wild-caught nauplii showed a drastic decrease in survival rate for 8 days after the start of the experiment, whereas after 8 days does not decrease any more. This is considered to showing that only individuals with strong tolerance survived except for those which are vulnerable to the effects of acidification.
Our finding that wild-caught adults were more sensitive to increased CO2 than culture-derived adults suggests that the latter group is more acclimated to lower pH environments. The pH of the seawater from which the wild-caught groups were obtained was 7.93, whereas that of the cultured group was 7.76. If latter is already adapted to a lower pH environment, it is possible that they might be less sensitive to a decrease in pH. But, contrary to the results of this study, some other studies investigating adult harpacticoids, including T. japonicus, found strong resistance and tolerance even at very high concentrations of CO2 (Kurihara et al. Citation2007; Cao et al. Citation2015). However, referring studies clearly differed from ours in the target individuals used. It was not a taxonomical difference, but the difference between individuals collected at sea and cultivated for several generations in a laboratory. Many of the studies on harpacticoid copepods have used individuals derived from laboratory cultures maintained for many generations (McAllen & Taylor Citation2001; Fitzer et al. Citation2012; Kita et al. Citation2013). Indeed, the aforementioned studies that found a strong tolerance in T. japonicus to ocean acidification used culture-derived individuals. However, it is possible that these may have developed physiological differences to wild-living individuals. The results of the current study partially support this conclusion.
Our study of T. japonicus found a difference in CO2 tolerance between adults and nauplii, as well as between those from the wild and from culture. Our results confirm that T. japonicus is a useful experimental organism for investigating ocean acidification. The data showed a decrease in survival with increasing CO2 concentration, and this effect was greater in wild-collected individuals than in the culture-derived individuals. We suggest that copepods collected directly from the sea may be more suitable for ocean acidification experiments in consideration of the possibility of the field application and usefulness of the experimental results. Further work is required to determine the extent and nature of physiological changes between wild-living individuals and those in culture. Additionally, the effects of ocean acidification on a range of harpacticoid copepod species needs to be examined and further research into environmental factors that can act as co-stressors is needed to predict the shifts in harpacticoid copepod communities that may be induced in the future.
Acknowledgements
We are very grateful to the Feed Organism Laboratory at Gangneung-Wonju National University, who provided us with the cultivated adult of Tigriopus japonicus. We wish to thank our colleagues who helped in the sample collection and experiment.
Disclosure statement
No potential conflict of interest was reported by the authors.
ORCID
Wonchoel Lee http://orcid.org/0000-0002-9873-1033
Additional information
Funding
References
- Barata C, Medina M, Telfer T, Baird DJ. 2002. Determining demographic effects of cypermethrin in the marine copepod Acartia tonsa: stage-specific short tests versus life-table tests. Arch Environ Contam Toxicol. 43:373–378. doi: 10.1007/s00244-002-1268-2
- Barka S, Pavillon JF, Amiard JC. 2001. Influence of different essential and non-essential metals on MTLP levels in the copepod Tigriopus brevicornis. Comp Biochem Physiol. 128:497–493.
- Barry JP, Buck KR, Lovera C, Kuhnz L, Whaling PJ. 2005. Utility of deep sea CO2 release experiments the biology of a high-CO2 ocean: effect of hypercapnia on deep sea meiofauna. J Geophys Res. 110:09–12. doi: 10.1029/2004JC002629
- Cao Z, Mu F, Wei X, Sun Y. 2015. Influence of CO2-induced seawater acidification on the development and lifetime reproduction of Tigriopus japonicus Mori, 1938. J Nat Hist. 49:2813–2826. doi: 10.1080/00222933.2015.1034213
- Cripps G, Lindeque P, Flynn KJ. 2014. Have we been underestimating the effects of ocean acidification in zooplankton? Glob Change Biol. 20:3377–3385. doi: 10.1111/gcb.12582
- Dupont S, Thorndyke MC. 2009. Impact of CO2-driven ocean acidification on invertebrates early life-history. What we know, what we need to know and what we can do? Biogeosci Discuss. 6:3109–3131. doi: 10.5194/bgd-6-3109-2009
- Fabry VJ, Seibel BA, Feely RA, Orr JC. 2008. Impacts of ocean acidification on marine fauna and ecosystem processes. ICES J Mar Sci. 65:414–432. doi: 10.1093/icesjms/fsn048
- Feely RA, Sabine CL, Lee K, Berelson W, Kleypas JA, Fabry VJ, Millero FJ. 2004. Impact of anthropogenic CO2 on the CaCO3 system in the oceans. Science. 305:362–366. doi: 10.1126/science.1097329
- Fitzer SC, Caldwell GS, Close AJ, Clare AS, Upstill-Goddard RC, Bentley MG. 2012. Ocean acidification induces multi-generational decline in copepod naupliar production with possible conflict for reproductive resource allocation. J Exp Mar Biol Ecol. 418–419:30–36. doi: 10.1016/j.jembe.2012.03.009
- Forget J, Pavillon JF, Menasria MR, Bocquené G. 1998. Mortality and LC50 for several stages of marine copepod Tigriopve brevicornis (Müller) exposed to the metals arsenic and cadmium and the pesticides atrazine, carbofuran, dichlorvos, and malathion. Ecotoxicol Environ Saf. 40:239–244. doi: 10.1006/eesa.1998.1686
- Fujita K, Hikami M, Suzuki A, Kuroyanagi A, Sakai K, Kawahata H, Nojiri Y. 2011. Effects of ocean acidification on calcification of symbiont-bearing reef foraminifers. Biogeosciences. 8:2089–2098. doi: 10.5194/bg-8-2089-2011
- Gattuso JP, Frankignoulle M, Bourge I, Romaine S, Buddemeier RW. 1998. Effect of calcium carbonate saturation of seawater on coral calcification. Glob Planet Change. 18:37–46. doi: 10.1016/S0921-8181(98)00035-6
- Green AS, Chandler GT, Piegorsch WW. 1996. Life-stage-specific toxicity of sediment-associated chlorpyrifos to a marine, infaunal copepod. Environ Toxicol Chem. 15:1182–1188. doi: 10.1002/etc.5620150725
- Hildebrandt N, Niehoff B, Sartoris FJ. 2014. Long-term effects of elevated CO2 and temperature on the Arctic calanoid copepods Calanus glacialis and C. hyperboreus. Mar Pollut Bull. 80:59–70. doi: 10.1016/j.marpolbul.2014.01.050
- IPCC. 2007. Summary for policymakers in climate change 2007: the physical science basis. Contribution of working group I to the fourth assessment report of the Intergovernmental Panel on Climate Change. Cambridge (UK): University Press.
- IPCC. 2014. Synthesis report in climate change 2014: contribution of working groups I, II and III to the fifth assessment report of the Intergovernmental Panel on Climate Change. Geneva, Switzerland: IPCC.
- Ito T. 1970. The biology of the harpacticoid copepod Tigriopus japonicus Mori. J Fac Sci Hokkaido Univ Ser 6 Zool. 17:474–500.
- Kim TW, Barry JP. 2016. Boldness in a deep sea hermit crab to simulated tactile predator attacks is unaffected by ocean acidification. Ocean Sci J. 51:381–386. doi: 10.1007/s12601-016-0034-8
- Kim TW, Barry JP, Micheli F. 2013. The effects of intermittent exposure to low-pH and low-oxygen conditions on survival and growth of juvenile red abalone. Biogeosciences. 10:7255–7262. doi: 10.5194/bg-10-7255-2013
- Kim TW, Taylor J, Lovera C, Barry JP. 2016. CO2-driven decrease in pH disrupts olfactory behaviour and increases individual variation in deep-sea hermit crabs. ICES J Mar Sci. 73:613–619. doi: 10.1093/icesjms/fsv019
- Kita J, Kikkawa T, Asai T, Ishimatsu A. 2013. Effects of elevated pCO2 on reproductive properties of the benthic copepod Tigriopus japonicus and gastropod Babylonia japonica. Mar Pollut Bull. 73:402–408. doi: 10.1016/j.marpolbul.2013.06.026
- Kurihara H, Kato S, Ishimatsu A. 2007. Effects of increased seawater pCO2 on early development of the oyster Crassostrea gigas. Aquat Biol. 1:91–98. doi: 10.3354/ab00009
- Kusk K, Wollenberger L. 1999. Fully defined saltwater medium for cultivation of and toxicity testing with marine copepod Acartia tonsa. Environ Toxicol Chem. 18:1564–1567. doi: 10.1002/etc.5620180731
- Langenbuch M, Pörtner HO. 2004. High sensitivity to chronically elevated CO2 levels in a eurybathic marine sipunculid. Aquat Toxicol. 70:55–61. doi: 10.1016/j.aquatox.2004.07.006
- Lee WJ. 1991. Efficiency of various microbial foods for Tigriopus japonicus Mori. Bull Korean Fish Soc. 24:117–122.
- Lee JA, Kim TW. 2016. Effects of potential future CO2 levels in seawater on emerging behaviour and respiration of Manila clams, Venerupis philippinarum. ICES J Mar Sci. doi: 10.1093/icesjms/fsw124
- Lotufo GR. 1997. Toxicity of sediment-associated PAHs to an estuarine copepod: effect on survival, feeding, reproduction and behavior. Mar Environ Res. 44:149–166. doi: 10.1016/S0141-1136(96)00108-0
- Mayor DJ, Matthews C, Cook K, Zuur AF, Hay S. 2007. CO2-induced acidification affect hatching success in Calanus finmarchicus. Mar Ecol Prog Ser. 350:91–97. doi: 10.3354/meps07142
- McAllen R, Taylor A. 2001. The effect of salinity change on the oxygen consumption and swimming activity of the high-shore rock pool copepod Tigriopus brevicornis. J Exp Mar Biol Ecol. 263:227–240. doi: 10.1016/S0022-0981(01)00308-2
- McAllen R, Taylor AC, Davenport J. 1999. The effects of temperature and oxygen partial pressure on the rate of oxygen consumption of the high-shore rock pool copepod Tigriopus brevicornis. Comp Biochem Physiol. 123:195–202. doi: 10.1016/S1095-6433(99)00050-1
- Pascal PY, Fleeger JW, Galvez F, Carman KR. 2010. The toxicological interaction between ocean acidity and metals in coastal meiobenthic copepods. Mar Pollut Bull. 60:2201–2208. doi: 10.1016/j.marpolbul.2010.08.018
- Pörtner HO. 2008. Ecosystem effects of ocean acidification in times of ocean warming: a physiologist’s view. Mar Ecol Prog Ser. 373:203–217. doi: 10.3354/meps07768
- Pörtner HO, Farrell AP. 2008. Ecology: physiology and climate change. Science. 322:690–692. doi: 10.1126/science.1163156
- Pörtner HO, Langenbuch M, Reipschlager A. 2004. Biological impact of elevated ocean CO2 concentrations: lessons from animal physiology and earth history. J Oceanogr. 60:705–718. doi: 10.1007/s10872-004-5763-0
- Pounds NA, Hutchinson TH, Williams TD, Whiting P, Dinan L. 2002. Assessment of putative endocrine disrupters in an in vivo crustacean assay and an in vitro insect assay. Mar Environ Res. 54:709–713. doi: 10.1016/S0141-1136(02)00113-7
- Przeslawski R, Zhu Q, Aller R. 2009. Effects of abiotic stressors on infaunal burrowing and associated sediment characteristics. Mar Ecol Prog Ser. 392:33–42. doi: 10.3354/meps08221
- Riebesell U, Zondervan I, Rost B, Tortell PD, Zeebe RE, Morel FMM. 2000. Reduced calcification of marine plankton in response to increase atmospheric CO2. Nature. 407:364–367. doi: 10.1038/35030078
- Sung CJ, Kim TW, Park YG, Kang SG, Inaba K, Shiba K, Choi TS, Moon SD, Litvin S, Lee KT, Lee JS. 2014. Species and gamete-specific fertilization success of two sea urchins under near future levels of pCO2. J Mar Syst. 137:67–73. doi: 10.1016/j.jmarsys.2014.04.013
- Suwa R, Nojiri Y, Ono T, Shirayama Y. 2013. Effects of low pCO2 conditions on sea urchin larval size. Mar Ecol. 34:443–450. doi: 10.1111/maec.12044
- Thistle D, Sedlacek L, Carman KR, Fleeger JW, Brewer PG, Barry JP. 2006. Simulated sequestration of industrial carbon dioxide at a deep-sea site: effects on species of harpacticoid copepods. J Exp Mar Biol Ecol. 330:151–158. doi: 10.1016/j.jembe.2005.12.023
- Weydmann A, Søreide JE, Kwasniewski S, Widdicombe S. 2012. Influence of CO2-induced acidification on the reproduction of a key Arctic copepod Calanus glacialis. J Exp Mar Biol Ecol. 428:39–42. doi: 10.1016/j.jembe.2012.06.002
- Widdicombe S, Spicer JI. 2008. Predicting the impact of ocean acidification on benthic biodiversity: what can animal physiology tell us? J Exp Mar Biol Ecol. 366:187–197. doi: 10.1016/j.jembe.2008.07.024