ABSTRACT
Tauopathies as one of the typical neurodegenerative diseases are characterized by pathologically aggregated tau in neuron and glia, which causes dysfunction, synapse loss, and cognitive impairments. There are more than 26 cases that are classified according to major tau isoform aggregates production or abnormal tau generation processes. Although the various concepts for targeting tau mechanisms have been investigated, the obvious limitations still remain and consequently, hindering their applicability. Therefore, additional strategies are required in the therapeutics and diagnosis. Here, we briefly summarized the etiological tau in the hyperphosphorylation, and the present concepts of tau-targeted disease therapies and diagnosis. We suggest the potential of approaches from deep learning-based single-cell transcriptome to microbiome and discuss the encountered challenges for the applicability and practicality.
Introduction
In recent years, neurodegenerative diseases influence more than 50 millions of people worldwide (Ehrenberg et al. Citation2020). The number of patients is increasing as the aging population is growing (Kim et al. Citation2023; Van Schependom and D’Haeseleer Citation2023; Wilson et al. Citation2023). In the effort to develop treatment and preventive methods, numerous studies have attempted to uncover underlying mechanisms of neurodegenerative diseases, including Alzheimer’s disease (AD), Parkinson’s disease (PD) and Huntington’s disease (HD), revealing the common risk factors such as tau, α-synuclein, and amyloid β (Aβ) (Hansson Citation2021; Khan et al. Citation2023).
The microtubule-associated protein tau (MAPT) gene, which consists of 16 exons on human chromosome 17q21, encodes tau protein organized with four main domains: N-terminal domain, proline-rich region, microtubule-binding repeat region (MTBR) and C-terminal domain (Garcia-Escudero et al. Citation2017; Barbier et al. Citation2019; Sallaberry et al. Citation2021). Alternative splicing-derived six isoforms of tau are determined by exon 2 and 3 insertion in the N-terminal (0N, 1N or 2N) and exon 10 insertion as the second repeat region that leads to three or four repeat regions (3R or 4R) in the MTBR (Lester et al. Citation2021; Sexton et al. Citation2022) (). Tau 3R and 4R isoforms are normally detected at a 1:1 ratio in the brain. However, the imbalanced ratio may cause tauopathies with cognitive impairment (Espindola et al. Citation2018). Furthermore, when dysregulated, common post-translational modifications such as phosphorylation, acetylation, glycosylation and methylation can play a role in the acceleration of tau aggregation. Aggregated tau reduces binding affinity to microtubules and leads to neurofibrillary tangles (NFTs) in the neuron and glia, causing the synaptic dysfunction, cognitive impairment, and neuronal loss (Wang and Mandelkow Citation2016).
Figure 1. Tau aggregation-associated with diverse mechanisms. There are six tau isoforms, and unregulated 3R or 4R isoform promote the tau accumulation, which is grouped into the 3R or 4R tauopathies. Dysregulated post-translational modification of tau involving the phosphorylation, acetylation, glycosylation, and methylation, also caused the aggregation, especially hyperphosphorylation is known as typical tau pathology.
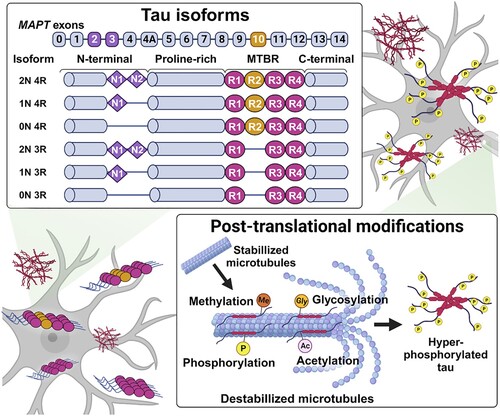
Throughout the last decade, multifaceted methods involving imaging or fluid-based diagnosis, inhibitor drugs, immunization, antisense oligonucleotides (ASOs), and CRISPR systems have been reported for tau-directed diagnostics or therapeutics (Jadhav et al. Citation2019; Sexton et al. Citation2022). Several tau-targeting therapies have entered clinical trials phase II. Unfortunately, limitations such as the toxicity, off-target effect, and complications associated with the delivery through blood–brain barrier (BBB) make tauopathies incurable, thus additional therapeutic approaches are required to advance (Zhang et al. Citation2022).
Conspicuously progressed sequencing technology and genome-wide association studies (GWAS) have guided the comprehensive elucidation of genetic interactions between tau and several disease-specific genes that can be potential candidates in neurodegenerative diseases involving tauopathies (Kim et al. Citation2021; Lim et al. Citation2021a, Citation2021b; Kim et al. Citation2023). To date, many studies have focused on the relevance between neurodegenerative diseases and individual cells to explain the brain region-specific pathological features through the sequencing analysis, including single-cell transcriptome analysis (Luo et al. Citation2020; Olah et al. Citation2020; Park and Jung Citation2022).
On the other hand, the recent studies are reported the microbiota-gut-brain axis, which is the complex communication of direct or indirect signaling network through the neuronal metabolic, endocrine, and immunity in the brain development and pathology of neurodegenerative diseases (Dash et al. Citation2022; Damiani et al. Citation2023). The microbiome has been highlighted to be diagnostic biomarkers and therapeutic objectives in neurodegenerative diseases (Zhu et al. Citation2021; Chandra et al. Citation2023). These efforts have revealed the branches of broader mechanism in tauopathies, which may provide promising directions in the practical applications.
In this review, we summarize the interaction between hyperphosphorylation of tau proteins and tau-associated factors in tauopathies and overview the current therapeutic and diagnostic strategies to discuss their advantages. Finally, we discuss the potential of meaningful approaches using the deep learning-based single-cell transcriptome and microbiome.
Tau pathology
Tauopathies are categorized into a broad range of neurodegenerative diseases, possessing a hallmark of insoluble tau fibrils and diverse spatial patterns of deposits. Because native tau itself rarely folds or promotes aggregate formation, it can be detected when the native tau structure is compromised due to multiple causes, including dysregulated phosphorylation (Wang and Mandelkow Citation2016). Subsequent to these tau abnormalities, tau proteins are accumulated and knotted inside neurons, called NFTs. Tau NFTs are strongly associated with local atrophy and the exacerbation of neurodegenerative manifestations, including neuronal loss, synaptic weakening, cognitive impairment, and eventual clinical decline (Bejanin et al. Citation2017; Xie et al. Citation2018; Berron et al. Citation2021). The spreading and propagation of tau tangles, which characterize tauopathies, have been observed in both human patients and tau-mice through brain imaging, and in the overall regions of the human brain using a predictive model (Fung et al. Citation2020; Vogel et al. Citation2020; Buciuc et al. Citation2023). Tangles have long been considered a proximate cause of pathological functioning in neurodegenerative diseases. However, the exact mechanisms and further relations remain elusive and also debated as insufficient to induce neurodegeneration.
The precise etiologies remain the subject of controversial, however, numerous studies have described tau hyperphosphorylation as an essential clue. Microtubule structural integrity enables neurons to maintain proper morphological dynamics throughout their axons and dendrites (Iwanski and Kapitein Citation2023). Tau phosphorylation occurs in the context of representative amino acid residues, such as serine (S), tyrosine (Y), or threonine (T), and counts for over 85 potential sites (Xia et al. Citation2021). The primary phospho-epitope on tau has been identified as 17 distinct sites consisting of serine-proline or threonine-proline, which are suspected to regulate tau and microtubule disassembly due to their interdependency. The initial phosphorylated sites on tau (e.g. tau-181) named ‘master sites’ were demonstrated to enhance distal phosphorylation at phospho-available sites that note a hierarchy among phospho-sites (Stefanoska et al. Citation2022). When tau is hyperphosphorylated at specific sites, its affinity for microtubules decreases, with the risk of microtubule destabilization consequently increasing. Tau homeostasis is ascribed to the balance of tau-associated kinases and phosphatases, whereby overactivated kinases empower phosphorylation rather than dephosphorylation (Wainaina et al. Citation2014).
Several classes of kinases and phosphatases can add or remove phosphate groups from tau. Phosphate is also added by tyrosine kinases (e.g. Fyn, LCK, SYK, and ABL1), proline-directed kinases (e.g. GSK3, CDK5, MAPK, and JNK), and non-proline-directed kinases (e.g. PKA, TTBK and MARK) (Hanger et al. Citation2009). Glycogen synthase kinase 3 (GSK3), which mostly attaches a phosphate group to S/T residues, is a ubiquitously expressed kinase that is particularly enriched in the brain. The regulatory cascade of both isoform GSK3α and β to tau has been examined regarding tau phosphorylation at specific residues and its subsequent physiological effects, such as promotion of neuronal impairment and cognitive dysfunction (Fan et al. Citation2022; Zhou et al. Citation2022). Fyn is another tau regulatory-associated kinase that implicates a phosphorylated tyrosine site known as Tyr-18, which has been shown to decrease calcium influx and tau accumulation in synapse (Miyamoto et al. Citation2017; Briner et al. Citation2020). The cAMP-dependent protein kinase (PKA) also targets tau to phosphorylate, which results in NFTs formation and calcium dysregulation (Carlyle et al. Citation2014). The intriguing aspect is that PKA primes tau for subsequent phosphorylation by GSK3 and CDK5 catalytic activity (Liu et al. Citation2006). Otherwise, tau dephosphorylation is performed by protein phosphatase 2A (PP2A; dominant activity of nearly 71%), PP1, PP5, and PP2B (Noble et al. Citation2013; Taleski and Sontag Citation2018). PP2A is a response to diminished tau phosphorylate activity and is considered to be linked to Alzheimer’s disease (Rudrabhatla and Pant Citation2011). PP2A imposes dephosphorylation at its specific S/T sites on tau (e.g. S214, S262, T205, and T212), which shows different catalytic efficiencies and indirect correlations with other kinases (Kruse et al. Citation2020).
In addition to hyperphosphorylation, multiple classes of RNAs and RNA-binding proteins (RBPs) can also contribute to tau aggregates formation, accumulation, and mislocalization, and comprise an emerging field of study (Patrick et al. Citation2017; Kavanagh et al. Citation2022). Nuclear speckle-related RNA, snRNAs, and snoRNAs are revealed to be enriched in tau aggregates and a colocalization-mediated alteration in soma has been identified (Lester et al. Citation2021). The long noncoding RNA, NEAT1, has also been investigated to participate in the tau pathway by regulating microtubule stabilization (Zhao et al. Citation2020). The GTPase-activating protein-binding protein 2 (G3BP2) has recently been demonstrated to inhibit tau inclusion following its direct binding to the MTBR (Wang et al. Citation2023). With regard to the tau regulatory cascade, multiple factors, such as the residue of phosphorylation, the participating kinases/phosphatases, and other molecules orchestrate tau pathophysiology. However, the mechanisms of pathogenesis and further progress of tau still remain unclear, despite the intensive research, which has been figured out dynamic correlations in tau cell biology.
Current approaches to tauopathies
In recent years, many therapeutic and diagnostic avenues have been suggested to target the tau to regulate the abnormal isoform and expression, aggregation, and several kinases through the four therapeutic concepts; tau immunotherapy, inhibitor drugs, ASOs, CRISPR/Cas9 and tau clearance (), as well as one diagnosis concept; deep learning-based images (Vega et al. Citation2021; Sexton et al. Citation2022) ().
Figure 2. Summary of current tau therapies and diagnosis. The graphical diagram summarizes the current tau-targeting therapies and diagnosis methods. Therapeutic concepts are composed the ASOs, CRISR/Cas9, inhibitor drugs, tau immunotherapy, and clearance. Diagnosis concepts are applied to various tau images, including the hyperspectral images, PET, tau-immunostaining, and fluorescence resonance energy transfer (FRET) (Kim et al. Citation2023) that can be used to train the deep learning for the prediction of tau pathology.
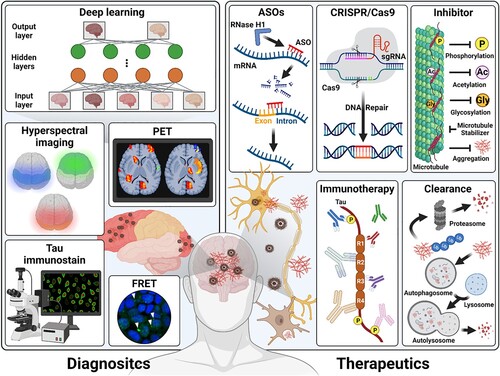
Table 1. Current mechanisms and clinical phases of tau-targeting therapeutic strategies.
Tau immunotherapy and inhibitor drugs
Tau immunotherapy utilizes the passive or active immunization strategies to decrease the hyperphosphorylation and accumulation of tau (Vaz and Silvestre Citation2020). The tau vaccine is the active immunotherapy that facilitates to produce endogenous antibody to response the pathogenic tau proteins through several tau-specific epitopes carrying antigens. The AADvac1 and ACI-35 are representative tau vaccine, which only remained clinical research in a decade, and have been entered the phase II trial (Novak et al. Citation2021; Song et al. Citation2022). Anti-tau antibodies are the passive immunotherapy that designed to bind the specific tau epitopes and can interact the intracellular tau after endocytosis. Semorinemab, Gosuranemab, Tilavonemab, Bepranemab, Zagotenemab, JNJ-63733657, PNT001, LuAF87908, and RG7345 has been investigated in phase I or II trial up to date. Consequently, both tau vaccine and anti-tau antibodies reduced the total tau or diverse p-tau through their specific binding motif to tau protein that also inhibited the diffusion of extracellular tau. However, they need to understand the exact timing for treatment and therapeutic efficiency (Plotkin and Cashman Citation2020; Panza and Lozupone Citation2022).
Inhibitor drugs are designed to target the post-translational modifications of tau to disturb the progression of tauopathies. They have been investigated to confirm the efficiency in clinical trials; The kinase inhibitors are the Fyn inhibitor (Saracatinb), GSK3β inhibitor (Tideglusib), and p75NTR inhibitor (LM11A) in trial phase II; The glycosylation inhibitors are MK-8719, and ASN120290 in trial phase I or II; The acetylation inhibitor is Salsalate in trial phase I; The aggregation inhibitors are leuco-methylthioninium-bis (LMTM), and grape seed polyphenolic extract (GPSE) in trial phase II or III; The microtubule stabilizers are Epothilone D, and Davunetide in trial phase I or II (Islam et al. Citation2022).
ASOs and CRISPR/Cas9
The ASOs are the synthesized short single-stranded oligodeoxynucleotides, which are designed to pair complementary to target mRNA, and this complex allows to degrade by the endonuclease RNase H1, or interrupt the interaction with RBPs involving the ribosomal subunits via blocking the AUG start site, thereby inhibit translation to subsequent target protein (Rinaldi and Wood Citation2018). Additionally, ASOs can alter splicing during RNA processing to bind the splice site or factors, thus it manipulates the target exon to be skipped or retention. Therefore, it has been developed to target MAPT gene to regulate the tau expression or isoform. However, ASOs have a common considerable impediment for the clinical application in brain disorders, which are their poor permeability across the BBB because of negative charge and size. Thereby, they are used to deliver via intrathecal and intraventricular administration (Chakravarthy et al. Citation2020; Damianich et al. Citation2021; Easton et al. Citation2022).
CRISPR/Cas9 is a remarkable gene-editing technology from the prokaryotic adaptive immune system. It is composed of single guide RNA (sgRNA) and Cas9 protein; the sgRNA guides Cas9 to bind target sequences, and the Cas9 works as the CRISPR-associated endonuclease to cleave the DNA to edit or repair the sequence (Konermann et al. Citation2018; Song and Koo Citation2021). It has been investigated to apply the AD therapeutics and developed to modify the construct to cross the BBB, such as adeno-associated virus and nanocomplex (Park et al. Citation2019; Bhardwaj et al. Citation2022).
Tau clearance
Eliminating aggregated tau is an expeditious method to suppress the progression in tauopathies. The various mechanisms of tau clearance have been reported in the degradation of protein pathway; ubiquitin-proteasome system and autophagy-lysosome system in intracellular tau, as well as microglia and astrocytes uptakes in extracellular tau (Tang et al. Citation2019). The ubiquitin-proteasome system components have been investigated for therapies, including the ubiquitin-specific peptidase 12 (USP12), USP13, USP14, USP30, E3 enzymes, and ubiquitin C-terminal hydrolase L1 (UCHL1). USP13 knockdown reduces the p-tau level via activating tau clearance in AD (Liu et al. Citation2019; Schmidt et al. Citation2021; Kim et al. Citation2023). The autophagy-lysosome system targeting the mammalian targets of rapamycin (mTOR) kinase shows reduced the autophagy signal, in turn, the mTOR kinase inhibitors, such as AZD2014, AZD8055, OSI-027 and rapamycin, alleviated aggregation of tau (Salama et al. Citation2019; Silva et al. Citation2020). The endocytosis has been reported to activate glial cells cascades through tau tangles attachment to microglia surface factors, the CX3C motif chemokine receptor 1 (CX3CR1) in microglia, and integrins on astrocytes (Perea et al. Citation2020; Seitkazina et al. Citation2022).
Diagnosis with tau images
Diagnostic methods for tau using the brain tissue have high accuracy, but collecting tissue from living organisms is not suitable for routine or early diagnosis. Imaging and fluid-based tau biomarkers have been used to diagnostic strategies in patients with tauopathies (Lee et al. Citation2019; Bergauer et al. Citation2022). Deep learning-based imaging analysis has been raised a remarkable approach that trained the deep learning using the diverse images of tau to understand better the tau aggregation and localization across tauopathies in diagnosis (Ushizima et al. Citation2022). Current studies were exhibited the applicability the diagnosis in tauopathies using the deep learning-based positron emission tomography (PET) hyperspectral imaging, and tau-immunostaining (Jo et al. Citation2020; Du et al. Citation2022; Koga et al. Citation2022). In addition, recent studies have been developed the fluorescence resonance energy transfer (FRET)-based biosensor cell lines, which are stably expressed the tau protein with cyan fluorescent protein (CFP) and yellow fluorescent protein (YFP). The aggregated tau has been excited by the YFP signal by the emission of CFP as the FRET signal. Therefore, the analysis of FRET-based tau aggregation may be a diagnostic avenue (Kim et al. Citation2023; Maina et al. Citation2023).
Novel applicability to tau therapy
Deep learning-based single-cell transcriptome
Intriguingly, several studies were demonstrated the distinct tau lesions in the brain and different cell types of accumulated tau within AD, corticobasal degeneration (CBD), and progressive supranuclear palsy (PSP). These showed the cell-to-cell interaction and spread over several brain compartment of tau (Narasimhan et al. Citation2020; Mate de Gerando et al. Citation2021). Several genetic signatures have revealed that susceptibility against abnormal tau depends on regional and cellular vulnerability. For example, Bcl2-associated athanogene 3 (BAG3) gene known to be involved in tau homeostasis was a preferential expression in excitatory neuron compare to inhibitory neuron (Fu et al. Citation2019). These evidences are suggested the individualized properties of deposited tau that may cause distinct effect on cell types or brain regions ().
Figure 3. Potency of deep learning-based single-cell transcriptome and microbiome in tauopathies. Schematic diagram showed the procedures of deep learning-based single-cell transcriptome and microbiota-gut-brain axis interaction in brain to enhance the comprehensive understand of tau pathology. These approaches suggested the applicability of tauopathies therapy that the cell type-specific properties and genetic interaction of tau, as well as crosstalk between brain and metabolites of gut microbiota, such as SCFAs, AAM, and TMAO.
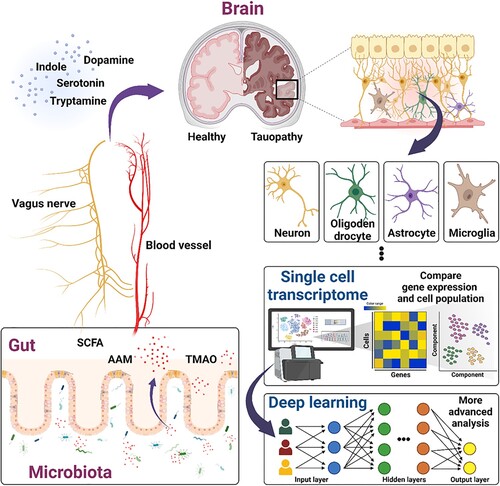
Single-cell transcriptome analysis is a more specific technology than the traditional whole genome sequencing from bulk sampling, especially in the gene expression, thus it provides a crucial understanding of the cell type, state or unique distribution. Currently, various evidences have been reported that the cell type-specific differences of pathological gene expression may be associated with clinical severity in neurodegenerative diseases (Han and Luo Citation2023). Using the single-cell transcriptome analysis from the prefrontal cortex of 48 individuals with diverse conditioned AD, 1031 differentially expressed genes (DEGs) were separated from the excitatory, inhibitory neurons, astrocytes, oligodendrocytes, oligodendrocyte precursor cells, and microglia; high percentages of DEGs are 75% downregulated in excitatory neurons and 95% in inhibitory neurons, while 53–63% of DEGs are upregulated in astrocytes, oligodendrocytes, and microglia. Moreover, the myelination-related genes expression was showed mainly the cell type-specific DEGs. For example, the LINGO1 was commonly altered the expression in almost all cell types, while BEX1, CNTNAP2, ERBIN, NEGR1, and PRNP were only changed in neurons, as well as CRTAB in only glia. Similarly, APOE as the AD risk marker, was significantly increased in microglia, while decreased in astrocytes. Besides, the cell subpopulation of specific markers expression also changed in AD (Mathys et al. Citation2019; Luo et al. Citation2020). In 2022, novel subtypes of early stage AD-associated microglia (EADAM), and late stage AD-associated microglia (LADAM) were identified from the mice with Aβ and tau, as well as several siglec genes were selectively regulated in the EADAM or LADMA that related to functions of immune systems (Kim et al. Citation2022). These cell type-specific DEGs or disease-specific cell population was identified not only in the neuronal cells but also in the immune cells in AD. Using the 36,849 peripheral blood mononuclear cells (PBMCs) from AD patients, different subpopulation of five immune cells was reported that CD4+ T cells were upregulated, while CD8+ T cells, natural killer cells, and monocytes-macrophages cells were downregulated. In the B cells, there were not specific changes. There were also showed several key of DEGs, but need to further studies to explain the exact mechanisms (Xu and Jia Citation2021). While other study was showed the decrease of B cell in the PBMCs of both early and late stage AD patients. In the B cells of early and late stages AD, KIR3DL2, QPCT, PPP2R2B genes were increased and FRAT2, WWC3, SPG20 were decreased commonly. These DEGs in the late stage of AD were more significantly changed the expression than the early stage of AD in the B cells (Xiong et al. Citation2021).
Although they found the specific DEGs, there were limitations to define their functions because of the lack of cerebrospinal fluid (CSF) or brain samples from diverse stages, inaccuracy due to background noise during the single-cell isolation, and unintegrated massive sequencing data. To improve the problems, the deep learning-based approach has been suggested for the single-cell transcriptome analysis (Yan et al. Citation2021). Deep learning belonging the artificial intelligence (AI) has deep neural networks composed of multiple layers that lead to learn from large datasets as the human brain, thereby it can perform more complicated functions than machine learning (Yang et al. Citation2023). Application of deep learning in single-cell transcriptome analysis has operated to obtain more accurate and enhanced information from the massive complex, noisy, and heterogeneous single-cell transcriptome data. Then, it allows to overcome the crucial challenges of single-cell transcriptome analysis, including the upstream process as the production of quality samples, standardized diverse noisy and low quality cells, as well as downstream process as the analysis of biological features with cell, gene, and pathway from a large amount of data. However, since its relatively lack of datasets, it is insufficient to demonstrate the correlation within the cell or disease-specific properties of tau and biological mechanisms as described as cell-tocell connection and DEGs, thus further studies are still required (Brendel et al. Citation2022; Erfanian et al. Citation2023).
Microbiome and tau pathology
Examining the microbiota-gut-brain axis has been suggested as a valuable insight regarding the role of microbiota in numerous physiological regulations in the brain, including neurodevelopment, microglial immune function, myelination, and other fundamental activities (Cryan et al. Citation2019) (). Commensal gut microbes are known to perform several physiological functions in the host and comprise a highly diverse and complex community (Thursby and Juge Citation2017). The uniqueness of gut microbiomes due to their compositional pattern has been demonstrated to reflect a condition of human. In this context, comparisons among healthy, aging, pathogenic and diseased people throughout the entire body can reveal distinct in regulatory effects on microbiota and microbiota-derivatives dependent manner (Wilmanski et al. Citation2021; Hou et al. Citation2022).
Several distinctive microbial taxa have been known to exist comparatively more abundant in AD patients’ stool than in normal individuals that mostly contain thousands of species. Such taxa include Escherichia/Shigella and Eubacterium rectale, which potentially lead to progressively more detrimental AD phenomena (Cattaneo et al. Citation2017). A recent study has shown the relation between the AD preclinical signature tau by PET and the gut microbiome, by examining the altered phosphorylation of tau-181 in CSF (Ferreiro et al. Citation2023). Distortions in the controlling system of microbiota throughout the gastrointestinal tract and brain axis result from the production of unbeneficial metabolites for the host. Certain bacterial metabolites can permeate a loosened BBB, while other bacterial signals can be conveyed through the vagus nerve via the enteric nervous system (Braniste et al. Citation2014; Bonaz et al. Citation2018). The vagus nerve provides a direct communication route with microbiota to the brain facilitated by neuroactive molecule-mediated regulation, which may stimulate cellular signaling in the central nervous system. The vagus nerve recognizes microbiota-derivatives through its afferent fibers, and the delivered information can perpetuate dysfunctional conditions, such as the production and secretion of microglial cytokine as a consequence (Bonaz et al. Citation2018; Han et al. Citation2022).
Metabolites of gut microbiota have been exerted to be involved in the brain via their absorbance through the gut-brain axis cognate receptors, by which short-chain fatty acids (SCFAs), amino acid metabolites (AAM), trimethylamine N-Oxide (TMAO), and other metabolites can manipulate the crosstalk with the brain system (Swer et al. Citation2023) (). SCFAs representing butyric acid, acetic acid, and propionic acid, are small organic compounds produced by microbiota in the gastrointestinal environment, which confer local protection. In addition, SCFAs can modulate enterochromaffin cells to increase 5-HT synthesis cascades and may cross the BBB by circulation in the bloodstream with the support of monocarboxylate transporters (MCTs). Following the SCFAs infiltration into the brain, G protein-coupled receptors (FFAR2 and FFAR3) activation and histone deacetylase (HDAC) inhibition have been reported as their major mechanisms (Guo et al. Citation2022). SCFAs have been associated with the underlying mechanisms in several brain and neuronal disorders, and exacerbating amyloid beta deposition (Colombo et al. Citation2021). More recent research has highlighted an interdependency between SCFAs and tau-mediated disorder through ApoE isoform carrying tau transgenic mice model (Seo et al. Citation2023). AAM is amino acids from gut bacterial fermentation, are converted by metabolizing phenylalanine and tryptophan, which transduce signals related to host physiological regulation. These two essential amino acids are processed by the host-microbial metabolic pathway; for example, phenylalanine is a precursor of several neurotransmitters, such as dopamine, whereas tryptophan is a precursor of serotonin and indole (Dodd et al. Citation2017; Liu et al. Citation2020). Tryptamine from tryptophan can easily cross BBB and has been detected to induce NFTs in human cells and mice (Paley et al. Citation2007). The TMAO has also been reported to have positively correlated with tau phosphorylation in the CSF, but not in NFTs (Vogt et al. Citation2018).
Table 2. Microbiota-derived metabolites and relations to tauopathies and brain.
Regarding the microbiota-gut-brain dependency in disease research, methods for visceral bacterial composition changes through fecal microbiota transplantation (FMT) have been used (Matheson and Holsinger Citation2023). This method has mostly been employed to transit the healthy microbiota into a pathological environment for non-healthy recipients. Multiple studies have supported the positive results deriving from this method, for example, mouse models presenting with amyloid and NFTs display a distinct attenuation of NFTs formation following FMT, together with improvement in cognitive function (Kim et al. Citation2020). Similarly, APPswe/PSEN1dE9 mice exhibit alleviated tau phosphorylation after FMT (Sun et al. Citation2019). Moreover, FMT from young donor mice was found to reverse age-associated exceedingly harmful effects in the nervous system of recipient mice by modulating microglial activity, inflammatory cytokines, and metabolic pathways (Jing et al. Citation2021; Parker et al. Citation2022). Meanwhile, systemic dysbiosis may occur via an indeterminate mechanism-mediated cascade, resulting in adverse outcomes when a detailed understanding of the microbiota community composition in different contexts is lacking with substantial strategies (Marrs and Walter Citation2021).
Although the results of the microbiota transition are controversial, the unique composition of the microbiome depending on host physiology has been verified by metagenomic sequencing and profiling (Zhu et al. Citation2021). Since the axis focuses on bidirectional communication-derived influence, coupling longitudinal genetic analysis to other molecular disciplinary omics is expected to further delineate the interaction machinery of the microbiota-gut-brain axis (Cryan and Mazmanian Citation2022; Diener et al. Citation2022). Relativeness with several neurological disorders including psychiatric, developmental, and degenerative has also been accumulating through emerging evidence (Warner Citation2019; Socala et al. Citation2021; Cutuli et al. Citation2022). Here, functional characterization using next-generation sequencing (NGS) was utilized in order to obtain high-throughput data for extensive analysis (Wensel et al. Citation2022). Although certain limitations, such as bias and missing information of the unique taxon reference sequence database remain. Advanced sequencing technologies are continuously improving the access to contextual information, by which spatial resolution of the microbiome, metagenomics at the single-cell scale, and gut mucosal-specific IgA antibody-based shotgun sequencing (Yen and Johnson Citation2021).
One of the ultimate goals of interconnectivity studies is to reveal underlying causality and address the clinical treatment of brain disorders. Across the highly complex microbiota ecosystem, blood-secreted metabolites, their particular compositions, and molecular intervention could contribute as signatures for diagnosis and further therapies (Zhan et al. Citation2023), and phenylacetylglutamine (PAGln) has been found as a cardiovascular disease marker (Huynh Citation2020; Nemet et al. Citation2020). Numerous questions remain unanswered, especially in terms of the microbiota-dependent signal transmission for tau phosphorylation and NFTs manifestation. A hitherto research on the largely unknown interplay between tauopathies and the microbiome is still in its infancy and requires further insights.
Conclusion
Through the continuous research regarding on tauopathies, numerous methods have been made and attempted to evolve the tau-targeted disease-modifying therapy as a promising therapeutics. The deep learning-based single-cell transcriptome and microbiome have been suggested the novel strategies that showed the potent cell type-specific properties and genetic cooperation with tau in tauopathies, as well as the interplay between the microbiota and aberrant tau. Nevertheless, these approaches are still in the stage of infancy and are not enough to explain the circumstantial connection with tau pathology. In addition, abnormal tau-induced DEGs, unique cell populations or microbiota in tauopathies need to demonstrate the exact pathway what the main functions are among the protective response or pathogenic response. Moreover, longitudinal research are also required to discriminate the properties of pathological tau that depend on the stages of progression in diseases. Taken together, while these approaches need further comprehensive understanding, it is carefully suggested the strategic avenues that may provide meaningful criteria for tauopathies.
Declaration of interest
The authors report no declarations of interest.
Acknowledgements
Conceptualization, J. Y. Joo; data curation, M. Kang; writing – original draft preparation, S. H. Kim and S. Yang; writing – review and editing, S. Yang and E. Yang; visualization, S. H. Kim and E. Yang; supervision, project administration and funding acquisition, J. Y. Joo. All authors have read and agreed to the published version of the manuscript. We thank Jeehye Jung for assistance of graphical diagrams. The graphical figures were made with biorender.com.
Disclosure statement
No potential conflict of interest was reported by the author(s).
Additional information
Funding
References
- Barbier P, Zejneli O, Martinho M, Lasorsa A, Belle V, Smet-Nocca C, Tsvetkov PO, Devred F, Landrieu I. 2019. Role of tau as a microtubule-associated protein: structural and functional aspects. Front Aging Neurosci. 11:204. doi:10.3389/fnagi.2019.00204.
- Bejanin A, Schonhaut DR, La Joie R, Kramer JH, Baker SL, Sosa N, Ayakta N, Cantwell A, Janabi M, Lauriola M, et al. 2017. Tau pathology and neurodegeneration contribute to cognitive impairment in Alzheimer’s disease. Brain. 140(12):3286–3300. doi:10.1093/brain/awx243.
- Bergauer A, van Osch R, van Elferen S, Gyllvik S, Venkatesh H, Schreiber R. 2022. The diagnostic potential of fluid and imaging biomarkers in chronic traumatic encephalopathy (CTE). Biomed Pharmacother. 146:112602. doi:10.1016/j.biopha.2021.112602.
- Berron D, Vogel JW, Insel PS, Pereira JB, Xie L, Wisse LEM, Yushkevich PA, Palmqvist S, Mattsson-Carlgren N, Stomrud E, et al. 2021. Early stages of tau pathology and its associations with functional connectivity, atrophy and memory. Brain. 144(9):2771–2783. doi:10.1093/brain/awab114.
- Bhardwaj S, Kesari KK, Rachamalla M, Mani S, Ashraf GM, Jha SK, Kumar P, Ambasta RK, Dureja H, Devkota HP, et al. 2022. CRISPR/Cas9 gene editing: new hope for Alzheimer’s disease therapeutics. J Adv Res. 40:207–221. doi:10.1016/j.jare.2021.07.001.
- Bonaz B, Bazin T, Pellissier S. 2018. The vagus nerve at the interface of the microbiota-gut-brain axis. Front Neurosci. 12:49. doi:10.3389/fnins.2018.00049.
- Bourassa MW, Alim I, Bultman SJ, Ratan RR. 2016. Butyrate, neuroepigenetics and the gut microbiome: can a high fiber diet improve brain health? Neurosci Lett. 625:56–63. doi:10.1016/j.neulet.2016.02.009.
- Braniste V, Al-Asmakh M, Kowal C, Anuar F, Abbaspour A, Toth M, Korecka A, Bakocevic N, Ng LG, Kundu P, et al. 2014. The gut microbiota influences blood-brain barrier permeability in mice. Sci Transl Med. 6(263):263ra158. doi:10.1126/scitranslmed.3009759.
- Brendel M, Su C, Bai Z, Zhang H, Elemento O, Wang F. 2022. Application of deep learning on single-cell RNA sequencing data analysis: a review. Genom Proteom Bioinform. 20(5):814–835. doi:10.1016/j.gpb.2022.11.011.
- Briner A, Gotz J, Polanco JC. 2020. Fyn kinase controls tau aggregation in vivo. Cell Rep. 32(7):108045. doi:10.1016/j.celrep.2020.108045.
- Buciuc M, Koga S, Pham NTT, Duffy JR, Knopman DS, Ali F, Boeve BF, Graff-Radford J, Botha H, Lowe VJ, et al. 2023. The many faces of globular glial tauopathy: a clinical and imaging study. Eur J Neurol. 30(2):321–333. doi:10.1111/ene.15603.
- Cao T, Zhou X, Zheng X, Cui Y, Tsien JZ, Li C, Wang H. 2018. Histone deacetylase inhibitor alleviates the neurodegenerative phenotypes and histone dysregulation in presenilins-deficient mice. Front Aging Neurosci. 10:137. doi:10.3389/fnagi.2018.00137.
- Carlyle BC, Nairn AC, Wang M, Yang Y, Jin LE, Simen AA, Ramos BP, Bordner KA, Craft GE, Davies P, et al. 2014. cAMP-PKA phosphorylation of tau confers risk for degeneration in aging association cortex. Proc Natl Acad Sci U S A. 111(13):5036–5041. doi:10.1073/pnas.1322360111.
- Cattaneo A, Cattane N, Galluzzi S, Provasi S, Lopizzo N, Festari C, Ferrari C, Guerra UP, Paghera B, Muscio C, et al. 2017. Association of brain amyloidosis with pro-inflammatory gut bacterial taxa and peripheral inflammation markers in cognitively impaired elderly. Neurobiol Aging. 49:60–68. doi:10.1016/j.neurobiolaging.2016.08.019.
- Chakravarthy M, Chen S, Wang T, Veedu RN. 2020. Development of novel chemically-modified nucleic acid molecules for efficient inhibition of human MAPT gene expression. Genes (Basel). 11(6):667. doi:10.3390/genes11060667.
- Chandra S, Sisodia SS, Vassar RJ. 2023. The gut microbiome in Alzheimer’s disease: what we know and what remains to be explored. Mol Neurodegener. 18(1):9. doi:10.1186/s13024-023-00595-7.
- Chapelet G, Beguin N, Castellano B, Grit I, de Coppet P, Oullier T, Neunlist M, Blottiere H, Rolli-Derkinderen M, Le Drean G, et al. 2023. Tau expression and phosphorylation in enteroendocrine cells. Front Neurosci. 17:1166848. doi:10.3389/fnins.2023.1166848.
- Colombo AV, Sadler RK, Llovera G, Singh V, Roth S, Heindl S, Sebastian Monasor L, Verhoeven A, Peters F, Parhizkar S, et al. 2021. Microbiota-derived short chain fatty acids modulate microglia and promote Aβ plaque deposition. Elife. 10. doi:10.7554/eLife.59826.
- Cryan JF, Mazmanian SK. 2022. Microbiota-brain axis: context and causality. Science. 376(6596):938–939. doi:10.1126/science.abo4442.
- Cryan JF, O’Riordan KJ, Cowan CSM, Sandhu KV, Bastiaanssen TFS, Boehme M, Codagnone MG, Cussotto S, Fulling C, Golubeva AV, et al. 2019. The microbiota-gut-brain axis. Physiol Rev. 99(4):1877–2013. doi:10.1152/physrev.00018.2018.
- Cutuli D, Giacovazzo G, Decandia D, Coccurello R. 2022. Alzheimer’s disease and depression in the elderly: a trajectory linking gut microbiota and serotonin signaling. Front Psychiatry. 13:1010169. doi:10.3389/fpsyt.2022.1010169.
- Damiani F, Cornuti S, Tognini P. 2023. The gut-brain connection: exploring the influence of the gut microbiota on neuroplasticity and neurodevelopmental disorders. Neuropharmacology. 231:109491. doi:10.1016/j.neuropharm.2023.109491.
- Damianich A, Facal CL, Muniz JA, Mininni C, Soiza-Reilly M, Ponce De Leon M, Urrutia L, Falasco G, Ferrario JE, Avale ME. 2021. Tau mis-splicing correlates with motor impairments and striatal dysfunction in a model of tauopathy. Brain. 144(8):2302–2309. doi:10.1093/brain/awab130.
- Dash S, Syed YA, Khan MR. 2022. Understanding the role of the gut microbiome in brain development and its association with neurodevelopmental psychiatric disorders. Front Cell Dev Biol. 10:880544. doi:10.3389/fcell.2022.880544.
- Diener C, Dai CL, Wilmanski T, Baloni P, Smith B, Rappaport N, Hood L, Magis AT, Gibbons SM. 2022. Genome-microbiome interplay provides insight into the determinants of the human blood metabolome. Nat Metab. 4(11):1560–1572. doi:10.1038/s42255-022-00670-1.
- Dodd D, Spitzer MH, Van Treuren W, Merrill BD, Hryckowian AJ, Higginbottom SK, Le A, Cowan TM, Nolan GP, Fischbach MA, et al. 2017. A gut bacterial pathway metabolizes aromatic amino acids into nine circulating metabolites. Nature. 551(7682):648–652. doi:10.1038/nature24661.
- Du X, Koronyo Y, Mirzaei N, Yang C, Fuchs DT, Black KL, Koronyo-Hamaoui M, Gao L. 2022. Label-free hyperspectral imaging and deep-learning prediction of retinal amyloid beta-protein and phosphorylated tau. PNAS Nexus. 1(4):pgac164. doi:10.1093/pnasnexus/pgac164.
- Easton A, Jensen ML, Wang C, Hagedorn PH, Li Y, Weed M, Meredith JE, Guss V, Jones K, Gill M, et al. 2022. Identification and characterization of a MAPT-targeting locked nucleic acid antisense oligonucleotide therapeutic for tauopathies. Mol Ther Nucleic Acids. 29:625–642. doi:10.1016/j.omtn.2022.07.027.
- Ehrenberg AJ, Khatun A, Coomans E, Betts MJ, Capraro F, Thijssen EH, Senkevich K, Bharucha T, Jafarpour M, Young PNE, et al. 2020. Relevance of biomarkers across different neurodegenerative diseases. Alzheimers Res Ther. 12(1):56. doi:10.1186/s13195-020-00601-w.
- Erfanian N, Heydari AA, Feriz AM, Ianez P, Derakhshani A, Ghasemigol M, Farahpour M, Razavi SM, Nasseri S, Safarpour H, et al. 2023. Deep learning applications in single-cell genomics and transcriptomics data analysis. Biomed Pharmacother. 165:115077. doi:10.1016/j.biopha.2023.115077.
- Erny D, Dokalis N, Mezo C, Castoldi A, Mossad O, Staszewski O, Frosch M, Villa M, Fuchs V, Mayer A, et al. 2021. Microbiota-derived acetate enables the metabolic fitness of the brain innate immune system during health and disease. Cell Metab. 33(11):2260–2276.e7. doi:10.1016/j.cmet.2021.10.010.
- Espindola SL, Damianich A, Alvarez RJ, Sartor M, Belforte JE, Ferrario JE, Gallo JM, Avale ME. 2018. Modulation of tau isoforms imbalance precludes tau pathology and cognitive decline in a mouse model of tauopathy. Cell Rep. 23(3):709–715. doi:10.1016/j.celrep.2018.03.079.
- Fan X, Xia L, Zhou Z, Qiu Y, Zhao C, Yin X, Qian W. 2022. Tau acts in concert with kinase/phosphatase underlying synaptic dysfunction. Front Aging Neurosci. 14:908881. doi:10.3389/fnagi.2022.908881.
- Ferreiro AL, Choi J, Ryou J, Newcomer EP, Thompson R, Bollinger RM, Hall-Moore C, Ndao IM, Sax L, Benzinger TLS, et al. 2023. Gut microbiome composition may be an indicator of preclinical Alzheimer’s disease. Sci Transl Med. 15(700):eabo2984. doi:10.1126/scitranslmed.abo2984.
- Fu H, Possenti A, Freer R, Nakano Y, Hernandez Villegas NC, Tang M, Cauhy PVM, Lassus BA, Chen S, Fowler SL, et al. 2019. A tau homeostasis signature is linked with the cellular and regional vulnerability of excitatory neurons to tau pathology. Nat Neurosci. 22(1):47–56. doi:10.1038/s41593-018-0298-7.
- Fung CW, Guo J, Fu H, Figueroa HY, Konofagou EE, Duff KE. 2020. Atrophy associated with tau pathology precedes overt cell death in a mouse model of progressive tauopathy. Sci Adv. 6:42.
- Garcia-Escudero V, Gargini R, Martin-Maestro P, Garcia E, Garcia-Escudero R, Avila J. 2017. Tau mRNA 3′UTR-to-CDS ratio is increased in Alzheimer disease. Neurosci Lett. 655:101–108. doi:10.1016/j.neulet.2017.07.007.
- Govindarajulu M, Pinky PD, Steinke I, Bloemer J, Ramesh S, Kariharan T, Rella RT, Bhattacharya S, Dhanasekaran M, Suppiramaniam V, et al. 2020. Gut metabolite TMAO induces synaptic plasticity deficits by promoting endoplasmic reticulum stress. Front Mol Neurosci. 13:138. doi:10.3389/fnmol.2020.00138.
- Guo C, Huo YJ, Li Y, Han Y, Zhou D. 2022. Gut-brain axis: focus on gut metabolites short-chain fatty acids. World J Clin Cases. 10(6):1754–1763. doi:10.12998/wjcc.v10.i6.1754.
- Hamamah S, Aghazarian A, Nazaryan A, Hajnal A, Covasa M. 2022. Role of microbiota-gut-brain axis in regulating dopaminergic signaling. Biomedicines. 10(2). doi:10.3390/biomedicines10020436.
- Han X, Luo FH. 2023. Application of single-cell RNA sequencing in probing oligodendroglia heterogeneity and neurological disorders. Yi Chuan. 45(3):198–211.
- Han Y, Wang B, Gao H, He C, Hua R, Liang C, Zhang S, Wang Y, Xin S, Xu J. 2022. Vagus nerve and underlying impact on the gut microbiota-brain axis in behavior and neurodegenerative diseases. J Inflamm Res. 15:6213–6230. doi:10.2147/JIR.S384949.
- Hanger DP, Anderton BH, Noble W. 2009. Tau phosphorylation: the therapeutic challenge for neurodegenerative disease. Trends Mol Med. 15(3):112–119. doi:10.1016/j.molmed.2009.01.003.
- Hansson O. 2021. Biomarkers for neurodegenerative diseases. Nat Med. 27(6):954–963. doi:10.1038/s41591-021-01382-x.
- Hou K, Wu ZX, Chen XY, Wang JQ, Zhang D, Xiao C, Zhu D, Koya JB, Wei L, Li J, et al. 2022. Microbiota in health and diseases. Signal Transduct Target Ther. 7(1):135. doi:10.1038/s41392-022-00974-4.
- Hoyles L, Snelling T, Umlai UK, Nicholson JK, Carding SR, Glen RC, McArthur S. 2018. Microbiome-host systems interactions: protective effects of propionate upon the blood-brain barrier. Microbiome. 6(1):55. doi:10.1186/s40168-018-0439-y.
- Huynh K. 2020. Novel gut microbiota-derived metabolite promotes platelet thrombosis via adrenergic receptor signalling. Nat Rev Cardiol. 17(5):265. doi:10.1038/s41569-020-0367-y.
- Islam M, Shen F, Regmi D, Du D. 2022. Therapeutic strategies for tauopathies and drug repurposing as a potential approach. Biochem Pharmacol. 198:114979. doi:10.1016/j.bcp.2022.114979.
- Iwanski MK, Kapitein LC. 2023. Cellular cartography: towards an atlas of the neuronal microtubule cytoskeleton. Front Cell Dev Biol. 11:1052245. doi:10.3389/fcell.2023.1052245.
- Jadhav S, Avila J, Scholl M, Kovacs GG, Kovari E, Skrabana R, Evans LD, Kontsekova E, Malawska B, de Silva R, et al. 2019. A walk through tau therapeutic strategies. Acta Neuropathol Commun. 7(1):22. doi:10.1186/s40478-019-0664-z.
- Jaglin M, Rhimi M, Philippe C, Pons N, Bruneau A, Goustard B, Dauge V, Maguin E, Naudon L, Rabot S. 2018. Indole, a signaling molecule produced by the gut microbiota, negatively impacts emotional behaviors in rats. Front Neurosci. 12:216. doi:10.3389/fnins.2018.00216.
- Jiang Y, Li K, Li X, Xu L, Yang Z. 2021. Sodium butyrate ameliorates the impairment of synaptic plasticity by inhibiting the neuroinflammation in 5XFAD mice. Chem Biol Interact. 341:109452. doi:10.1016/j.cbi.2021.109452.
- Jing Y, Yu Y, Bai F, Wang L, Yang D, Zhang C, Qin C, Yang M, Zhang D, Zhu Y, et al. 2021. Effect of fecal microbiota transplantation on neurological restoration in a spinal cord injury mouse model: involvement of brain-gut axis. Microbiome. 9(1):59. doi:10.1186/s40168-021-01007-y.
- Jo T, Nho K, Risacher SL, Saykin AJ, Alzheimer’s Neuroimaging Initiative. 2020. Deep learning detection of informative features in tau PET for Alzheimer’s disease classification. BMC Bioinformatics. 21(Suppl 21):496. doi:10.1186/s12859-020-03848-0.
- Kavanagh T, Halder A, Drummond E. 2022. Tau interactome and RNA binding proteins in neurodegenerative diseases. Mol Neurodegener. 17(1):66. doi:10.1186/s13024-022-00572-6.
- Khan S, Hassan MI, Shahid M, Islam A. 2023. Nature’s toolbox against tau aggregation: an updated review of current research. Ageing Res Rev. 87:101924. doi:10.1016/j.arr.2023.101924.
- Killingsworth J, Sawmiller D, Shytle RD. 2020. Propionate and Alzheimer’s disease. Front Aging Neurosci. 12:580001. doi:10.3389/fnagi.2020.580001.
- Kim DW, Tu KJ, Wei A, Lau AJ, Gonzalez-Gil A, Cao T, Braunstein K, Ling JP, Troncoso JC, Wong PC, et al. 2022. Amyloid-beta and tau pathologies act synergistically to induce novel disease stage-specific microglia subtypes. Mol Neurodegener. 17(1):83. doi:10.1186/s13024-022-00589-x.
- Kim JY, Kim W, Lee KH. 2023. The role of microRNAs in the molecular link between circadian rhythm and autism spectrum disorder. Anim Cells Syst. 27(1):38–52. doi:10.1080/19768354.2023.2180535.
- Kim MS, Kim Y, Choi H, Kim W, Park S, Lee D, Kim DK, Kim HJ, Choi H, Hyun DW, et al. 2020. Transfer of a healthy microbiota reduces amyloid and tau pathology in an Alzheimer’s disease animal model. Gut. 69(2):283–294. doi:10.1136/gutjnl-2018-317431.
- Kim SH, Lim KH, Yang S, Joo JY. 2023. Boosting of tau protein aggregation by CD40 and CD48 gene expression in Alzheimer’s disease. FASEB J. 37(1):e22702.
- Kim SH, Yang S, Lim KH, Ko E, Jang HJ, Kang M, Suh PG, Joo JY. 2021. Prediction of Alzheimer’s disease-specific phospholipase c gamma-1 SNV by deep learning-based approach for high-throughput screening. Proc Natl Acad Sci U S A. 118(3).
- Kim YJ, Lee Y, Shin H, Hwang S, Park J, Song EJ. 2023. Ubiquitin-proteasome system as a target for anticancer treatment-an update. Arch Pharm Res. 46(7):573–597. doi:10.1007/s12272-023-01455-0.
- Koga S, Ikeda A, Dickson DW. 2022. Deep learning-based model for diagnosing Alzheimer’s disease and tauopathies. Neuropathol Appl Neurobiol. 48(1):e12759. doi:10.1111/nan.12759.
- Konermann S, Lotfy P, Brideau NJ, Oki J, Shokhirev MN, Hsu PD. 2018. Transcriptome engineering with RNA-targeting type VI-D CRISPR effectors. Cell. 173(3):665–676.e14. doi:10.1016/j.cell.2018.02.033.
- Kruse T, Gnosa SP, Nasa I, Garvanska DH, Hein JB, Nguyen H, Samsoe-Petersen J, Lopez-Mendez B, Hertz EPT, Schwarz J, et al. 2020. Mechanisms of site-specific dephosphorylation and kinase opposition imposed by PP2A regulatory subunits. EMBO J. 39(13):e103695. doi:10.15252/embj.2019103695.
- Lee JC, Kim SJ, Hong S, Kim Y. 2019. Diagnosis of Alzheimer’s disease utilizing amyloid and tau as fluid biomarkers. Exp Mol Med. 51(5):1–10.
- Lee Y, Kang JS, Ham OJ, Son MY, Lee MO. 2022. Gut metabolite trimethylamine N-oxide induces aging-associated phenotype of midbrain organoids for the induced pluripotent stem cell-based modeling of late-onset disease. Front Aging Neurosci. 14:925227. doi:10.3389/fnagi.2022.925227.
- Lester E, Ooi FK, Bakkar N, Ayers J, Woerman AL, Wheeler J, Bowser R, Carlson GA, Prusiner SB, Parker R. 2021. Tau aggregates are RNA-protein assemblies that mislocalize multiple nuclear speckle components. Neuron. 109(10):1675–1691.e9. doi:10.1016/j.neuron.2021.03.026.
- Lim KH, Kim SH, Yang S, Chun S, Joo JY. 2021a. Advances in multiplex PCR for Alzheimer’s disease diagnostics targeting CDK genes. Neurosci Lett. 749:135715. doi:10.1016/j.neulet.2021.135715.
- Lim KH, Yang S, Kim SH, Joo JY. 2021b. Identifying new COVID-19 receptor neuropilin-1 in severe Alzheimer’s disease patients group brain using genome-wide association study approach. Front Genet. 12:741175. doi:10.3389/fgene.2021.741175.
- Liu F, Liang Z, Shi J, Yin D, El-Akkad E, Grundke-Iqbal I, Iqbal K, Gong CX. 2006. PKA modulates GSK-3beta- and cdk5-catalyzed phosphorylation of tau in site- and kinase-specific manners. FEBS Lett. 580(26):6269–6274. doi:10.1016/j.febslet.2006.10.033.
- Liu X, Hebron ML, Mulki S, Wang C, Lekah E, Ferrante D, Shi W, Kurd-Misto B, Moussa C. 2019. Ubiquitin specific protease 13 regulates tau accumulation and clearance in models of Alzheimer’s disease. J Alzheimers Dis. 72(2):425–441. doi:10.3233/JAD-190635.
- Liu Y, Hou Y, Wang G, Zheng X, Hao H. 2020. Gut microbial metabolites of aromatic amino acids as signals in host-microbe interplay. Trends Endocrinol Metab. 31(11):818–834. doi:10.1016/j.tem.2020.02.012.
- Luo ZG, Peng J, Li T. 2020. Single-Cell RNA sequencing reveals cell-type-specific mechanisms of neurological diseases. Neurosci Bull. 36(7):821–824. doi:10.1007/s12264-020-00496-5.
- Ma J, Wang R, Chen Y, Wang Z, Dong Y. 2023. 5-HT attenuates chronic stress-induced cognitive impairment in mice through intestinal flora disruption. J Neuroinflammation. 20(1):23. doi:10.1186/s12974-023-02693-1.
- Maina KN, Smet-Nocca C, Bitan G. 2023. Using FRET-based biosensor cells to study the seeding activity of tau and alpha-synuclein. Methods Mol Biol. 2551:125–145. doi:10.1007/978-1-0716-2597-2_10.
- Marrs T, Walter J. 2021. Pros and cons: is faecal microbiota transplantation a safe and efficient treatment option for gut dysbiosis? Allergy. 76(7):2312–2317. doi:10.1111/all.14750.
- Mate de Gerando A, d’Orange M, Augustin E, Josephine C, Auregan G, Gaudin-Guerif M, Guillermier M, Herard AS, Stimmer L, Petit F, et al. 2021. Neuronal tau species transfer to astrocytes and induce their loss according to tau aggregation state. Brain. 144(4):1167–1182. doi:10.1093/brain/awab011.
- Matheson JT, Holsinger RMD. 2023. The role of fecal microbiota transplantation in the treatment of neurodegenerative diseases: a review. Int J Mol Sci. 24(2). doi:10.3390/ijms24021001.
- Mathys H, Davila-Velderrain J, Peng Z, Gao F, Mohammadi S, Young JZ, Menon M, He L, Abdurrob F, Jiang X, et al. 2019. Single-cell transcriptomic analysis of Alzheimer’s disease. Nature. 570(7761):332–337. doi:10.1038/s41586-019-1195-2.
- Matt SM, Allen JM, Lawson MA, Mailing LJ, Woods JA, Johnson RW. 2018. Butyrate and dietary soluble fiber improve neuroinflammation associated with aging in mice. Front Immunol. 9:1832. doi:10.3389/fimmu.2018.01832.
- Miyamoto T, Stein L, Thomas R, Djukic B, Taneja P, Knox J, Vossel K, Mucke L. 2017. Phosphorylation of tau at Y18, but not tau-fyn binding, is required for tau to modulate NMDA receptor-dependent excitotoxicity in primary neuronal culture. Mol Neurodegener. 12(1):41. doi:10.1186/s13024-017-0176-x.
- Narasimhan S, Changolkar L, Riddle DM, Kats A, Stieber A, Weitzman SA, Zhang B, Li Z, Roberson ED, Trojanowski JQ, et al. 2020. Human tau pathology transmits glial tau aggregates in the absence of neuronal tau. J Exp Med. 217(2). doi:10.1084/jem.20190783.
- Nemet I, Saha PP, Gupta N, Zhu W, Romano KA, Skye SM, Cajka T, Mohan ML, Li L, Wu Y, et al. 2020. A cardiovascular disease-linked gut microbial metabolite acts via adrenergic receptors. Cell. 180(5):862–877 e822. doi:10.1016/j.cell.2020.02.016.
- Noble W, Hanger DP, Miller CC, Lovestone S. 2013. The importance of tau phosphorylation for neurodegenerative diseases. Front Neurol. 4:83. doi:10.3389/fneur.2013.00083.
- Novak P, Kovacech B, Katina S, Schmidt R, Scheltens P, Kontsekova E, Ropele S, Fialova L, Kramberger M, Paulenka-Ivanovova N, et al. 2021. ADAMANT: a placebo-controlled randomized phase 2 study of AADvac1, an active immunotherapy against pathological tau in Alzheimer’s disease. Nat Aging. 1(6):521–534. doi:10.1038/s43587-021-00070-2.
- Olah M, Menon V, Habib N, Taga MF, Ma Y, Yung CJ, Cimpean M, Khairallah A, Coronas-Samano G, Sankowski R, et al. 2020. Single cell RNA sequencing of human microglia uncovers a subset associated with Alzheimer’s disease. Nat Commun. 11(1):6129. doi:10.1038/s41467-020-19737-2.
- Paley EL. 2011. Tryptamine-induced tryptophanyl-tRNAtrp deficiency in neurodifferentiation and neurodegeneration interplay: progenitor activation with neurite growth terminated in Alzheimer’s disease neuronal vesicularization and fragmentation. J Alzheimers Dis. 26(2):263–298. doi:10.3233/JAD-2011-110176.
- Paley EL, Denisova G, Sokolova O, Posternak N, Wang X, Brownell AL. 2007. Tryptamine induces tryptophanyl-tRNA synthetase-mediated neurodegeneration with neurofibrillary tangles in human cell and mouse models. Neuromolecular Med. 9(1):55–82. doi:10.1385/NMM:9:1:55.
- Panza F, Lozupone M. 2022. The challenges of anti-tau therapeutics in Alzheimer disease. Nat Rev Neurol. 18(10):577–578. doi:10.1038/s41582-022-00702-0.
- Park H, Oh J, Shim G, Cho B, Chang Y, Kim S, Baek S, Kim H, Shin J, Choi H, et al. 2019. In vivo neuronal gene editing via CRISPR-Cas9 amphiphilic nanocomplexes alleviates deficits in mouse models of Alzheimer’s disease. Nat Neurosci. 22(4):524–528. doi:10.1038/s41593-019-0352-0.
- Park HJ, Jung H. 2022. Neuro-immune interactions at single-cell resolution in neurodevelopmental, infectious, and neurodegenerative diseases. Anim Cells Syst. 26(4):137–147. doi:10.1080/19768354.2022.2110937.
- Parker A, Romano S, Ansorge R, Aboelnour A, Le Gall G, Savva GM, Pontifex MG, Telatin A, Baker D, Jones E, et al. 2022. Fecal microbiota transfer between young and aged mice reverses hallmarks of the aging gut, eye, and brain. Microbiome. 10(1):68. doi:10.1186/s40168-022-01243-w.
- Patrick E, Rajagopal S, Wong HA, McCabe C, Xu J, Tang A, Imboywa SH, Schneider JA, Pochet N, Krichevsky AM, et al. 2017. Dissecting the role of non-coding RNAs in the accumulation of amyloid and tau neuropathologies in Alzheimer’s disease. Mol Neurodegener. 12(1):51. doi:10.1186/s13024-017-0191-y.
- Perea JR, Bolos M, Avila J. 2020. Microglia in Alzheimer’s disease in the context of tau pathology. Biomolecules. 10(10). doi:10.3390/biom10101439.
- Plotkin SS, Cashman NR. 2020. Passive immunotherapies targeting Aβ and tau in Alzheimer’s disease. Neurobiol Dis. 144:105010. doi:10.1016/j.nbd.2020.105010.
- Rinaldi C, Wood MJA. 2018. Antisense oligonucleotides: the next frontier for treatment of neurological disorders. Nat Rev Neurol. 14(1):9–21. doi:10.1038/nrneurol.2017.148.
- Rudrabhatla P, Pant HC. 2011. Role of protein phosphatase 2A in Alzheimer’s disease. Curr Alzheimer Res. 8(6):623–632. doi:10.2174/156720511796717168.
- Salama M, El-Desouky S, Alsayed A, El-Hussiny M, Magdy K, Fekry E, Shabka O, El-Khodery SA, Youssef MA, Sobh M, et al. 2019. siRNA blocking of mammalian target of rapamycin (mTOR) attenuates pathology in annonacin-induced tauopathy in mice. Neurotox Res. 35(4):987–992. doi:10.1007/s12640-018-9974-3.
- Sallaberry CA, Voss BJ, Majewski J, Biernat J, Mandelkow E, Chi EY, Vander Zanden CM. 2021. Tau and membranes: interactions that promote folding and condensation. Front Cell Dev Biol. 9:725241. doi:10.3389/fcell.2021.725241.
- Salminen A. 2023. Activation of aryl hydrocarbon receptor (AhR) in Alzheimer’s disease: role of tryptophan metabolites generated by gut host-microbiota. J Mol Med. 101(3):201–222. doi:10.1007/s00109-023-02289-5.
- Schmidt MF, Gan ZY, Komander D, Dewson G. 2021. Ubiquitin signalling in neurodegeneration: mechanisms and therapeutic opportunities. Cell Death Differ. 28(2):570–590. doi:10.1038/s41418-020-00706-7.
- Seitkazina A, Kim KH, Fagan E, Sung Y, Kim YK, Lim S. 2022. The fate of tau aggregates between clearance and transmission. Front Aging Neurosci. 14:932541. doi:10.3389/fnagi.2022.932541.
- Seo DO, O’Donnell D, Jain N, Ulrich JD, Herz J, Li Y, Lemieux M, Cheng J, Hu H, Serrano JR, et al. 2023. ApoE isoform- and microbiota-dependent progression of neurodegeneration in a mouse model of tauopathy. Science. 379(6628):eadd1236. doi:10.1126/science.add1236.
- Sexton C, Snyder H, Beher D, Boxer AL, Brannelly P, Brion JP, Buee L, Cacace AM, Chetelat G, Citron M, et al. 2022. Current directions in tau research: highlights from tau 2020. Alzheimers Dement. 18(5):988–1007. doi:10.1002/alz.12452.
- Silva MC, Nandi GA, Tentarelli S, Gurrell IK, Jamier T, Lucente D, Dickerson BC, Brown DG, Brandon NJ, Haggarty SJ. 2020. Prolonged tau clearance and stress vulnerability rescue by pharmacological activation of autophagy in tauopathy neurons. Nat Commun. 11(1):3258. doi:10.1038/s41467-020-16984-1.
- Socala K, Doboszewska U, Szopa A, Serefko A, Wlodarczyk M, Zielinska A, Poleszak E, Fichna J, Wlaz P. 2021. The role of microbiota-gut-brain axis in neuropsychiatric and neurological disorders. Pharmacol Res. 172:105840. doi:10.1016/j.phrs.2021.105840.
- Song C, Shi J, Zhang P, Zhang Y, Xu J, Zhao L, Zhang R, Wang H, Chen H. 2022. Immunotherapy for Alzheimer’s disease: targeting beta-amyloid and beyond. Transl Neurodegener. 11(1):18. doi:10.1186/s40035-022-00292-3.
- Song M, Koo T. 2021. Recent advances in CRISPR technologies for genome editing. Arch Pharm Res. 44(6):537–552. doi:10.1007/s12272-021-01336-4.
- Stefanoska K, Gajwani M, Tan ARP, Ahel HI, Asih PR, Volkerling A, Poljak A, Ittner A. 2022. Alzheimer’s disease: ablating single master site abolishes tau hyperphosphorylation. Sci Adv. 8(27):eabl8809. doi:10.1126/sciadv.abl8809.
- Sun J, Xu J, Ling Y, Wang F, Gong T, Yang C, Ye S, Ye K, Wei D, Song Z, et al. 2019. Fecal microbiota transplantation alleviated Alzheimer’s disease-like pathogenesis in APP/PS1 transgenic mice. Transl Psychiatry. 9(1):189. doi:10.1038/s41398-019-0525-3.
- Sun J, Zhang Y, Kong Y, Ye T, Yu Q, Kumaran Satyanarayanan S, Su KP, Liu J. 2022. Microbiota-derived metabolite indoles induced aryl hydrocarbon receptor activation and inhibited neuroinflammation in APP/PS1 mice. Brain Behav Immun. 106:76–88. doi:10.1016/j.bbi.2022.08.003.
- Swer NM, Venkidesh BS, Murali TS, Mumbrekar KD. 2023. Gut microbiota-derived metabolites and their importance in neurological disorders. Mol Biol Rep. 50(2):1663–1675. doi:10.1007/s11033-022-08038-0.
- Taleski G, Sontag E. 2018. Protein phosphatase 2A and tau: an orchestrated ‘Pas de deux’. FEBS Lett. 592(7):1079–1095. doi:10.1002/1873-3468.12907.
- Tang M, Harrison J, Deaton CA, Johnson GVW. 2019. Tau clearance mechanisms. Adv Exp Med Biol. 1184:57–68. doi:10.1007/978-981-32-9358-8_5.
- Thursby E, Juge N. 2017. Introduction to the human gut microbiota. Biochem J. 474(11):1823–1836. doi:10.1042/BCJ20160510.
- Tian H, Wang J, Feng R, Zhang R, Liu H, Qin C, Meng L, Chen Y, Fu Y, Liang D, et al. 2023. Efficacy of faecal microbiota transplantation in patients with progressive supranuclear palsy-Richardson’s syndrome: a phase 2, single centre, randomised clinical trial. EClinicalMedicine. 58:101888. doi:10.1016/j.eclinm.2023.101888.
- Ushizima D, Chen Y, Alegro M, Ovando D, Eser R, Lee W, Poon K, Shankar A, Kantamneni N, Satrawada S, et al. 2022. Deep learning for Alzheimer’s disease: mapping large-scale histological tau protein for neuroimaging biomarker validation. Neuroimage. 248:118790. doi:10.1016/j.neuroimage.2021.118790.
- Van Schependom J, D’Haeseleer M. 2023. Advances in neurodegenerative diseases. J Clin Med. 12(5). doi:10.3390/jcm12051709.
- Vaz M, Silvestre S. 2020. Alzheimer’s disease: recent treatment strategies. Eur J Pharmacol. 887:173554. doi:10.1016/j.ejphar.2020.173554.
- Vega AR, Chkheidze R, Jarmale V, Shang P, Foong C, Diamond MI, White CL, 3rd, Rajaram S. 2021. Deep learning reveals disease-specific signatures of white matter pathology in tauopathies. Acta Neuropathol Commun. 9(1):170. doi:10.1186/s40478-021-01271-x.
- Vogel JW, Iturria-Medina Y, Strandberg OT, Smith R, Levitis E, Evans AC, Hansson O, Alzheimer’s Disease Neuroimaging Initiative, Swedish BioFinder Study. 2020. Spread of pathological tau proteins through communicating neurons in human Alzheimer’s disease. Nat Commun. 11(1):2612. doi:10.1038/s41467-020-15701-2.
- Vogt NM, Romano KA, Darst BF, Engelman CD, Johnson SC, Carlsson CM, Asthana S, Blennow K, Zetterberg H, Bendlin BB, et al. 2018. The gut microbiota-derived metabolite trimethylamine N-oxide is elevated in Alzheimer’s disease. Alzheimers Res Ther. 10(1):124. doi:10.1186/s13195-018-0451-2.
- Wainaina MN, Chen Z, Zhong C. 2014. Environmental factors in the development and progression of late-onset Alzheimer’s disease. Neurosci Bull. 30(2):253–270. doi:10.1007/s12264-013-1425-9.
- Wang C, Terrigno M, Li J, Distler T, Pandya NJ, Ebeling M, Tyanova S, Hoozemans JJM, Dijkstra AA, Fuchs L, et al. 2023. Increased G3BP2-tau interaction in tauopathies is a natural defense against Tau aggregation. Neuron. 111(17):2660–2674.e9. doi:10.1016/j.neuron.2023.05.033.
- Wang Y, Mandelkow E. 2016. Tau in physiology and pathology. Nat Rev Neurosci. 17(1):5–21. doi:10.1038/nrn.2015.1.
- Warner BB. 2019. The contribution of the gut microbiome to neurodevelopment and neuropsychiatric disorders. Pediatr Res. 85(2):216–224. doi:10.1038/s41390-018-0191-9.
- Wei GZ, Martin KA, Xing PY, Agrawal R, Whiley L, Wood TK, Hejndorf S, Ng YZ, Low JZY, Rossant J, et al. 2021. Tryptophan-metabolizing gut microbes regulate adult neurogenesis via the aryl hydrocarbon receptor. Proc Natl Acad Sci U S A. 118(27):e2021091118. doi:10.1073/pnas.2021091118.
- Wensel CR, Pluznick JL, Salzberg SL, Sears CL. 2022. Next-generation sequencing: insights to advance clinical investigations of the microbiome. J Clin Invest. 132(7). doi:10.1172/JCI154944.
- Wilmanski T, Diener C, Rappaport N, Patwardhan S, Wiedrick J, Lapidus J, Earls JC, Zimmer A, Glusman G, Robinson M, et al. 2021. Gut microbiome pattern reflects healthy ageing and predicts survival in humans. Nat Metab. 3(2):274–286. doi:10.1038/s42255-021-00348-0.
- Wilson DM, 3rd, Cookson MR, Van Den Bosch L, Zetterberg H, Holtzman DM, Dewachter I. 2023. Hallmarks of neurodegenerative diseases. Cell. 186(4):693–714. doi:10.1016/j.cell.2022.12.032.
- Xia Y, Prokop S, Giasson BI. 2021. ‘Don’t phos over tau’: recent developments in clinical biomarkers and therapies targeting tau phosphorylation in Alzheimer’s disease and other tauopathies. Mol Neurodegener. 16(1):37. doi:10.1186/s13024-021-00460-5.
- Xiao L, Yan J, Yang T, Zhu J, Li T, Wei H, Chen J. 2021. Fecal microbiome transplantation from children with autism spectrum disorder modulates tryptophan and serotonergic synapse metabolism and induces altered behaviors in germ-free mice. mSystems. 6(2). doi:10.1128/mSystems.01343-20.
- Xie L, Das SR, Wisse LEM, Ittyerah R, Yushkevich PA, Wolk DA, Alzheimer’s Disease Neuroimaging Initiative. 2018. Early tau burden correlates with higher rate of atrophy in transentorhinal cortex. J Alzheimers Dis. 62(1):85–92. doi:10.3233/JAD-170945.
- Xiong LL, Xue LL, Du RL, Niu RZ, Chen L, Chen J, Hu Q, Tan YX, Shang HF, Liu J, et al. 2021. Single-cell RNA sequencing reveals B cell-related molecular biomarkers for Alzheimer’s disease. Exp Mol Med. 53(12):1888–1901. doi:10.1038/s12276-021-00714-8.
- Xu H, Jia J. 2021. Single-cell RNA sequencing of peripheral blood reveals immune cell signatures in Alzheimer’s disease. Front Immunol. 12:645666. doi:10.3389/fimmu.2021.645666.
- Yan R, Fan C, Yin Z, Wang T, Chen X. 2021. Potential applications of deep learning in single-cell RNA sequencing analysis for cell therapy and regenerative medicine. Stem Cells. 39(5):511–521. doi:10.1002/stem.3336.
- Yang S, Kim SH, Kang M, Joo JY. 2023. Harnessing deep learning into hidden mutations of neurological disorders for therapeutic challenges. Arch Pharm Res. 46(6):535–549. doi:10.1007/s12272-023-01450-5.
- Yen S, Johnson JS. 2021. Metagenomics: a path to understanding the gut microbiome. Mamm Genome. 32(4):282–296. doi:10.1007/s00335-021-09889-x.
- Zhan Y, Al-Nusaif M, Ding C, Zhao L, Dong C. 2023. The potential of the gut microbiome for identifying Alzheimer’s disease diagnostic biomarkers and future therapies. Front Neurosci. 17:1130730. doi:10.3389/fnins.2023.1130730.
- Zhang Y, Wu KM, Yang L, Dong Q, Yu JT. 2022. Tauopathies: new perspectives and challenges. Mol Neurodegener. 17(1):28. doi:10.1186/s13024-022-00533-z.
- Zhao Y, Wang Z, Mao Y, Li B, Zhu Y, Zhang S, Wang S, Jiang Y, Xu N, Xie Y, et al. 2020. NEAT1 regulates microtubule stabilization via FZD3/GSK3beta/P-tau pathway in SH-SY5Y cells and APP/PS1 mice. Aging. 12(22):23233–23250.
- Zheng H, Xu P, Jiang Q, Xu Q, Zheng Y, Yan J, Ji H, Ning J, Zhang X, Li C, et al. 2021. Depletion of acetate-producing bacteria from the gut microbiota facilitates cognitive impairment through the gut-brain neural mechanism in diabetic mice. Microbiome. 9(1):145. doi:10.1186/s40168-021-01088-9.
- Zhou Q, Li S, Li M, Ke D, Wang Q, Yang Y, Liu GP, Wang XC, Liu E, Wang JZ. 2022. Human tau accumulation promotes glycogen synthase kinase-3beta acetylation and thus upregulates the kinase: a vicious cycle in Alzheimer neurodegeneration. EBioMedicine. 78:103970. doi:10.1016/j.ebiom.2022.103970.
- Zhu X, Li B, Lou P, Dai T, Chen Y, Zhuge A, Yuan Y, Li L. 2021. The relationship between the gut microbiome and neurodegenerative diseases. Neurosci Bull. 37(10):1510–1522. doi:10.1007/s12264-021-00730-8.