ABSTRACT
Objectives
To evaluate the effect and mechanism of action of the flavonoid phloretin on the growth and sucrose-dependent biofilm formation of Streptococcus mutans.
Methods
Minimum inhibitory concentration, viability, and biofilm susceptibility assays were conducted to assess antimicrobial and antibiofilm effect of phloretin. Biofilm composition and structure were analysed with scanning electron microscopy (SEM) and confocal laser scanning microscopy (CLSM). Water-soluble (WSG) and water-insoluble glucan (WIG) were determined using anthrone method. Lactic acid measurements and acid tolerance assay were performed to assess acidogenicity and aciduricity. Reverse transcription quantitative PCR (RT-qPCR) was used to measure the expression of virulence genes essential for surface attachment, biofilm formation, and quorum sensing.
Results
Phloretin inhibited S. mutans growth and viability in a dose-dependent manner. Furthermore, it reduced gtfB and gtfC gene expression, correlating with the reduction of extracellular polysaccharides (EPS)/bacteria and WIG/WSG ratio. Inhibition of comED and luxS gene expression, involved in stress tolerance, was associated with compromised acidogenicity and aciduricity of S. mutans.
Conclusions
Phloretin exhibits antibacterial properties against S. mutans, modulates acid production and tolerance, and reduces biofilm formation.
Clinical significance
Phloretin is a promising natural compound with pronounced inhibitory effect on key virulence factors of the cariogenic pathogen, S. mutans.
Introduction
Dental caries is one of the most prevalent biofilm-mediated diseases worldwide modulated by multiple risk factors including consumption of dietary sugars, inadequate fluoride exposure, reduced saliva flow, poor oral hygiene and deleterious shift in oral microbiota [Citation1,Citation2]. Prevalence of cariogenic bacteria such as Streptococcus mutans in dental biofilm plays a discriminatory role in disease establishment and progression [Citation3,Citation4]. S. mutans gained a significant evolutionary advantage for persistence and surface colonisation via its ability to metabolize a large variety of carbohydrates present in human diet. Among them, sucrose has proven to be the most cariogenic [Citation5]. S. mutans has evolved several pathogenic traits essential for dental biofilm development and caries progression. First, it produces virulence enzymes such as fructosyltransferase (Ftf) and glucosyltransferase (Gtfs) to synthesise extracellular polysaccharides from dietary sugars. Thus, Gtfs convert sucrose into water-soluble glucans (WSG) and water-insoluble glucans (WIG) essential for structured biofilm matrix formation [Citation4,Citation6,Citation7]. Second, it uses several cell wall-associated proteins (e.g. SpaP, WapA and GbpC) to promote initial surface colonization and interbacterial interaction [Citation8,Citation9]. Third, S. mutans can metabolize dietary sugars for acid production (acidogenicity), which promotes further enamel demineralization and tooth decay [Citation5,Citation10]. Finally, S. mutans can tolerate various environmental stress conditions, such as an acidic environment in dental biofilm [Citation11,Citation12], particularly by exploiting two types of quorum sensing systems, ComCDE and LuxS-AI-2 [Citation13–15]. Therefore, therapeutic strategies, which target virulence factors of S. mutans, are of great interest for caries prevention and maintenance of oral health.
Currently available antimicrobial compounds used in oral healthcare products (e.g. chlorhexidine [Citation16], metal nanoparticles [Citation17] or anti-microbial peptides [Citation18,Citation19]) are broad-spectrum antibacterial agents that are often associated with side effects and limitations, such as risk of resistance development [Citation20], altered sensation, cellular toxicity or compound stability [Citation21]. In recent years, plant polyphenols and flavonoids have gained increased attention as alternative or adjunctive anti-caries compounds based on their broad availability, low toxicity and prominent antimicrobial and antibiofilm capacity including direct inhibition of key virulence factors of cariogenic bacteria [Citation22,Citation23].
The flavonoid family includes more than 6000 compounds, and based on their chemical structure, they are divided into eight main subgroups, such as flavones, flavonols, flavonones, flavononols, flavan-3-ols, anthocyanins, isoflavones and chalcones [Citation24]. Thus, extracts from Camelia sinensis enriched in flavan-3-ols (used for black, green and oolong tea production) exhibited antibacterial and antibiofilm activities against S. mutans and Streptococcus sobrinus [Citation25–27]. Flavone apigenin, an abundant component found in propolis, is an effective inhibitor of S. mutans virulence gene expression and one of the most potent inhibitors of Gtfs enzymes [Citation28–32]. Flavonols quercetin and kaempferol destroy S. mutans biofilm via multiple mechanisms including cytotoxic effect and reduction of insoluble and soluble glucan formation [Citation33]. Naringenin, a flavanone from citrus plants, exhibits antibacterial effects against S. mutans and suppresses the adhesion and maturation stages of S. mutans biofilm formation [Citation34]. Non-toxic cranberry flavonoids, such as A-type proanthocyanidins (PACs) and myricetin, have been shown to inhibit the activity of S. mutans Gtfs [Citation35,Citation36], sortase A (SrtA) [Citation37] and EPS-mediated bacterial adhesion in multispecies dental biofilm [Citation38]. Moreover, a few recent studies have indicated that flavonoids could potentially exhibit selectivity against oral pathogens. Thus, plant extract enriched with apigenin, quercetin and their derivatives selectively reduced S. mutans population in a dual-species biofilm with S. sanguinis in the presence of glucose [Citation39]. Flavonol morin leads to a decreased viability of aciduric bacteria in polymicrobial biofilms obtained from saliva [Citation40]. Additionally, disk diffusion assay showed that morin, luteolin and naringin effectively inhibited the growth of pathogens found in dental plaque including Aggregatibacter actinomycetemcomitans, Enterococcus faecalis, Escherichia coli, Staphylococcus aureus and Candida albicans, while remained inactive against commensal bacteria such as Streptococcus sanguinis and Streptococcus oralis [Citation41].
Identification and functional characterization of flavonoids with inhibitory properties against cariogenic pathogen S. mutans are essential to select potential candidates for an effective anti-caries therapy. Phloretin is a dihydrochalcone (, upper panel) found mainly in apple, pear and strawberry fruit and has been shown to possess a strong antimicrobial and antibiofilm activity against various bacteria including Bacillus subtilis [Citation42], Pseudomonas aeruginosa [Citation43] enterohemorrhagic E. coli O157:H7 strain [Citation44] and Streptococcus pyogenes [Citation45]. However, its effect on S. mutans remains unknown. In this study, using a broad spectrum of assays, we demonstrate that phloretin exhibits strong inhibitory effect on S. mutans growth and biofilm formation by targeting its key virulence factors such as acid production and tolerance. These results warrant further exploration of phloretin as an antimicrobial and anti-biofilm compound relevant for dental medicine.
Figure 1. Antibacterial and antibiofilm effect of phloretin on S. mutans. (a) growth curves of S. mutans in the presence of phloretin (coloured curves) in comparison to non-treated control (black curve) and solvent control (5% DMSO, open symbols). (b) the effect of phloretin on early (4 h, light grey) and late (24 h, dark grey) biofilm of S. mutans. Biofilm mass was quantified by CV staining and measuring absorbance at 595 nm. Bars represent the mean of three biological replicates. Error bars show standard deviation. (c) the effect of phloretin on 24 h biofilms of S. mutans formed on sHA. Representative sHA disks with S. mutans biofilm treated with 50 μg/ml, 100 μg/ml and 200 μg/ml of phloretin for 24 h and stained with crystal violet. NC, negative control (bacteria treated with 5% DMSO).
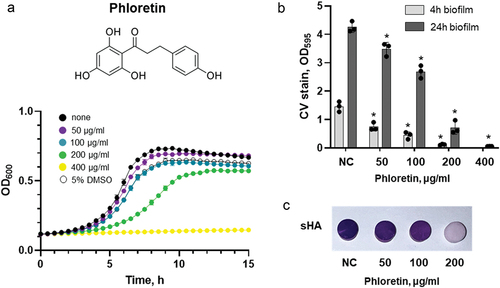
Materials and methods
Bacterial strains and growth conditions
S. mutans strain ATCC 25,175 was used in this study (Leibniz Institute DSMZ-German Collection of Microorganisms and Cell Cultures, DSM 20,523, serotype c, isolated from carious dentine). The bacteria were routinely cultured in Brain Heart Infusion Broth (BHI, Oxoid, Thermo Scientific) aerobically at 37°C in 5% CO2. For the biofilm assay, BHI was additionally supplemented with 1% (wt/vol) sucrose (BHIS). Phloretin was purchased from Sigma Aldrich, dissolved in 100% dimethyl sulfoxide (DMSO) to a stock concentration of 100 mg/ml, aliquoted and stored at −20°C.
Bacterial susceptibility assay
The minimal inhibitory concentration (MIC) and minimal bactericidal concentration (MBC) of phloretin were determined using a broth microdilution assay in 96-well polystyrene plates. Fresh S. mutans culture (OD600 = 0.5–0.6) was diluted 1:100 into 96-well plate using BHI supplemented with serial dilutions of phloretin and DMSO. The final concentration of DMSO in the assay did not exceed 5%. BHI supplemented with 5% DMSO was used as a negative control. The microplate was incubated aerobically at 37°C for 24 h. Optical density was measured at 600 nm using a microplate reader (Synergy HTX, Biotek, Agilent Technologies, Basel, Switzerland). MIC was determined by the lowest concentration of phloretin required to inhibit detectable bacterial growth. To assess the phloretin effect on S. mutans viability and determine MBC, 10-fold serial dilutions were made for each condition from the MIC microplate and 5 µl aliquots were spotted on BHI agar plate. MBC was determined by the lowest concentration of phloretin required to completely prevent bacterial growth on BHI agar plate. MIC and MBC assays were performed independently for three bacterial cultures.
Biofilm inhibition assay
Crystal violet staining assay was used to evaluate the inhibitory effect of flavonoids on biofilm formed on polystyrene microplate or on saliva-coated hydroxyapatite disks. For biofilm assay in microplate, a fresh S. mutans culture (OD600 = 0.5–0.6) was diluted 1:100 using BHIS supplemented with DMSO and, when indicated, two-fold serial dilutions of phloretin in DMSO. Final concentration of DMSO did not exceed 5%. Bacterial suspension was incubated aerobically at 37°C. After 4 h or 24 h of incubation, excess medium including the planktonic cells were removed, the plate was washed twice with sterile water and air-dried for 20 min. An equal volume of 0.1% (wt/vol) crystal violet solution was added to each well and incubated for 10 min at room temperature. The crystal violet solution was then removed and the plate was washed with water. To dissolve the dye, an equal volume of 25% acetic acid was added to each well, and absorbance at 595 nm was measured using a microplate reader (Synergy HTX, Biotek, Agilent Technologies, Basel, Switzerland).
For biofilm assay on hydroxyapatite, hydroxyapatite disks (5 mm diameter, HiMed Inc., USA) were coated with pooled filter-sterilized clarified human saliva at room temperature for 30 min to simulate the formation of an acquired pellicle-like layer (sHA). sHA disks were placed into the wells in a 96-well culture plate containing bacteria in BHIS with flavonoid dilutions. The culture plate was incubated for 24 h as described above. After incubation, sHA disks were washed with water to remove loosely attached cells and stained with crystal violet. The crystal violet solution was then removed, and sHA disks were thoroughly washed with water. An equal volume of 25% acetic acid was used to dissolve the dye for further quantification.
Saliva preparation
Whole saliva stimulated by chewing paraffin tablets was collected on ice from healthy donors free from systemic or oral topical medications, filtered through a 70-μm filter and clarified by centrifugation (21,000 g, 4°C, 40 min). The supernatant was further filtered through a double filter system of 0.45μm and 0.22 μm (Millex Millipore, Switzerland), aliquoted and stored at −20°C. Pooled saliva was prepared by mixing saliva from seven volunteers. All samples collected for this study involving human volunteers were stored anonymously and in accordance with the ethical standards of the institutional and national research committee and with the 1964 Helsinki declaration. All volunteers were informed about the study and upon agreeing to participate, their consent was registered.
Biofilm analysis and structural imaging
For the biofilm analysis by confocal laser scanning microscopy, the culture of S. mutans treated either with 5% DMSO (negative control) or phloretin was prepared as described above and 0.5 ml was added to the eight-well chambered borosilicate coverglass plate. Plates were incubated aerobically for 24 h. For quantification of bacteria and EPS composition in biofilm, S. mutans was cultured with 1 µM Alexa Fluor 647-labeled dextran conjugate (Invitrogen) for 24 h, while bacterial cells were labelled with SYTO 9 (Invitrogen) after the incubation, according to the manufacturer’s instruction. For viability assay in biofilm, bacteria were stained with SYTO 9 and Propidium iodide (PI) after incubation according to the manufacturer’s instructions (LIVE/DEAD BacLight bacterial viability staining kit, Invitrogen). Biofilm composition analysis and live/dead staining were performed separately on independent biofilms. The biofilms were visualized on Leica SP8 confocal laser scanning microscope (Leica Microsystem, Switzerland) using x63 oil immersion objective. The images were collected using 500/618 nm, 575/656 nm and 650/778 nm spectra for SYTO9, PI and Alexa Fluor 647, respectively. Z-stacks of biofilm images were acquired with 0.46 μm intervals at five arbitrary positions per sample. Biofilm scanning from the bottom to the top resulted in 75–78 slices for control biofilms and decreased to 35–40 slices for biofilm treated with 200 μg/ml of phloretin. Three-dimensional reconstruction and analysis of the biofilms were performed with Imaris 9.0.0 (Bitplane, Switzerland).
Scanning electron microscopy was used to analyse the architecture of S. mutans formed on sHA. The biofilms were prepared as described above. After 24-h incubation, the biofilms were washed three times with sterile PBS to remove planktonic and loosely adherent cells, and then fixed with 4% glutaraldehyde aqueous solution at 4°C overnight. The disks were washed once with PBS, and the samples were dehydrated in increasing concentrations of ethanol (30%, 50%, 70%, 90% and 100%), 10 min each. Samples were additionally dried using critical point drying (CPD) with liquid carbon dioxide and finally coated with a 20 nm gold layer. Disks were observed by SEM imaging (ESEM XL30, Philips, Eindhoven, The Netherlands) at magnifications of 200×, 2,000× and 8,000×. Each sample was scanned at three arbitrary selected locations. Image analysis was performed using ImageJ software (National Institutes of Health, USA). To analyse the cell morphology, at least three SEM images scanned with a magnification of 8,000× and 20,000× were used.
Water-soluble and water-insoluble EPS measurement
The effect of 100 μg/ml and 200 μg/ml of phloretin on water-soluble glucan (WSG) and water-insoluble glucan (WIG) production was estimated by the anthrone-sulphuric methods as described previously [Citation46] with modifications. Briefly, a fresh S. mutans culture (OD600 = 0.5–0.6) was diluted in 24-well plates in BHIS with phloretin. Biofilm was formed for 24 h at 37°C. After incubation, the culture medium was removed, and the biofilm was carefully rinsed with sterile PBS. One millilitre of sterile water was added to each well, and the biofilm was scraped from the bottom of the well with a sterile spatula. Water-soluble glucan from the suspension was dissolved by vigorous vortexing. The suspension was centrifuged at 6,000 g for 10 min, and the supernatant was collected in a separate tube for WSG measurement. WIGs was extracted from the cell sediment in 2 ml of 0.4 M NaOH with constant agitation for 2 h at 37°C. After incubation, cells were collected by centrifugation (6,000 g, 10 min). Supernatants from aqueous and alkaline extraction were mixed with 3 volumes of anthrone-sulfuric acid reagent and heated at 95°C for 6 min. The absorbance at 625 nm was measured in a 96-well plate using a microplate reader (Synergy HTX, Biotek, Agilent Technologies, Basel, Switzerland). The concentrations of WSG and WIG were calculated according to the standard curve prepared with the glucose standards (Figure S4). The experiment was performed in triplicates (independent bacterial cultures). Biofilm formed in BHIS with 5% DMSO was used as a negative control.
Lactic acid measurement
The effect of phloretin on S. mutans lactic acid production was evaluated for 50 μg/ml, 100 μg/ml, 200 μg/ml, and 400 μg/ml. A fresh S. mutans culture (OD600 = 0.5–0.6) was harvested and washed twice with PBS. Cells were resuspended 1:10 in buffered peptone water (BPW) supplemented with 0.2% sucrose in a 24-well plate. Two-fold serial dilutions of phloretin in DMSO were added to the wells. Bacterial suspension supplemented with DMSO was used as a negative control. Bacteria were incubated aerobically at 37°C for 2 h. S. mutans cells were then removed by centrifugation (8,000 g, 5 min, 4°C), and the supernatant was used to measure lactic acid concentration with a lactate assay kit (MAK064, Sigma-Aldrich, Switzerland), according to the manufacturer’s instruction. The measurements were performed with three biological replicates.
Acid tolerance assay
The effect of phloretin on the acid tolerance of S. mutans was determined by measuring its viability after 2 h of exposure to a pH of 5.0. The S. mutans cells were grown in BHI until the mid-log phase, harvested, washed with sterile PBS and then resuspended at a final concentration of 1.0 × 107 CFU/ml in buffered BHI, pH 5.0. Phloretin was added at concentrations 50 μg/ml, 100 μg/ml, 200 μg/ml and 400 μg/ml. Untreated bacterial suspension supplemented with 5% DMSO was used as the negative control. S. mutans cells grown in BHI pH 7.2 treated with the same phloretin concentration were used as a viability control. After incubation at 37°C for 2 h, aliquots from each condition were taken and serially diluted 10-fold in sterile PBS for further CFU counts. Aliquots of 100 μl were spread on BHI agar plate and incubated aerobically at 37°C for 2 days (Figure S5). The assays were independently performed with three biological replicates.
Transcriptional analysis of virulence genes
To determine the effect of phloretin on the gene expression levels in biofilm, S. mutans cells were cultured in 12-well plate for 24 h with 200 μg/ml phloretin as described above for the biofilm assay. Culture without treatment served as a control. After 24-h incubation, supernatants were carefully removed from the wells and biofilms were scraped with sterile spatula and resuspended in 1 ml RNAprotect bacterial reagent (Qiagen). After an incubation at RT for 5 min, pellets were collected by centrifugation for 10 min at 10,000 × g. Stabilized pellet was resuspended in 5 ml cold PBS and homogenized by sonication on ice to remove soluble polysaccharides. Three cycles of ultrasonic washing were performed with 30 s pulse. Next, the cells were resuspended in a lysis buffer with 15 mg/ml lysozyme and incubated for 15 min at RT. One millilitre TRIzol reagent (Invitrogen) was added to the suspension, and then transferred to the 2ml screw-cap tubes with 0.5 mm acid-washed glass beads. The cells were lysed by vertexing for 10 min at the highest speed. Total RNA was extracted using RNeasy kit (Qiagen) with an on-column DNase digestion step. The cDNA was synthesized using GoScript™ Reverse Transcriptase system with random primers (Promega, Switzerland). To quantify the mRNA expression, qRT – PCR was performed using FastStart Universal SYBR Green Master (ROX) (Roche Diagnostics, Mannheim, Germany). The PCR was performed in CFX96 Real-Time System (BioRad) in accordance with the manufacturer’s recommendations for determining the threshold cycle values (Ct). 2−ΔΔCt method was used to analyse the data. The sequences of the primers used in this study are listed in Supplementary Table 1. 16S rRNA was used as an internal control.
Statistical analysis
All assays were performed in biological replicates (independent bacterial cultures). The results are presented as mean ± standard deviation (SD). Statistically significant differences were determined with a one-way analysis of variance (ANOVA) followed by Dunnett’s multiple comparisons test: *P < 0.05. The results were graphed using GraphPad Prism version 9.0.2 (GraphPad Software, LLC, USA).
Results
Phloretin inhibited S. mutans viability and biofilm formation
The MIC and MBC of phloretin tested on planktonic S. mutans culture was 400 μg/ml. At sub-inhibitory concentration, gradual growth attenuation and reduction of cell viability were observed indicating a dose-dependent inhibitory effect of phloretin (, Figure S1). Dose-dependent biofilm inhibition was also observed in 4 h and 24 h biofilm assay with MBIC of 200 μg/ml and 400 μg/ml, respectively (). Similarly, on saliva-coated hydroxyapatite (sHA) surfaces, a condition mimicking a human oral environment, in 24 h, 200 ug/ml of phloretin drastically reduced biofilm formation (, Figure S2) resulting in a scarce number of surface-associated cells detected by SEM (). These cells also underwent a notable change in shape and structure (, Figure S3). In comparison to non-treated cells with symmetric ellipsoidal shape, in the presence of 200 µg/mL phloretin, bacteria appeared elongated, with multiple dysmorphic or swollen cells and asymmetric division sign (Figure S3A, red arrows). On average, non-treated control cells were 0.72 ± 0.11 µm long, while the length of the cells treated with 200 µg/ml phloretin was 1.1 ± 0.2 µm (Figure S3B). These observations might potentially indicate a perturbation in the coordination of cell elongation and cell division which is also reflected in compromised growth kinetics (). As assessed by the live/dead staining technique combined with CLSM, phloretin significantly reduced the proportion of live cells in 24 h biofilm, while the proportion of cells with compromised membrane, stained with PI, increased with increasing phloretin concentration ().
Figure 2. Phloretin effect on S. mutans adherence to HA. Representative scanning electron microscopy images (SEM) of S. mutans biofilms treated with 50 μg/ml, 100 μg/ml and 200 μg/ml of phloretin compared to negative control containing 5% DMSO. Images were taken at ×200, ×2 000 and ×8000 magnifications.
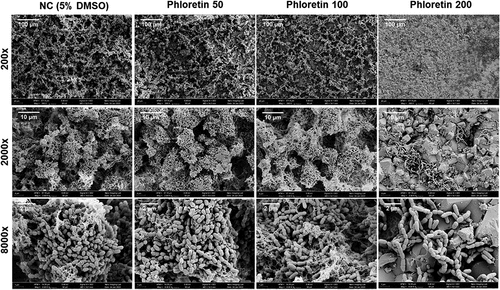
Figure 3. Antimicrobial effect of phloretin on S. mutans biofilm based on confocal laser scanning microscopy. (a) representative images of live/dead-stained 24-h biofilms. Live bacteria are shown in green and dead (PI-stained) in blue. Three-dimensional reconstructions were performed with Imaris 9.0.0. (b) right panel: bacterial biomasses as calculated by Imaris. Left panel: the proportion of live/dead cells. In all panels, bars represent the mean of five values calculated for arbitrary selected positions per sample. Error bars show standard deviation. NC, negative control (bacteria treated with 5% DMSO).
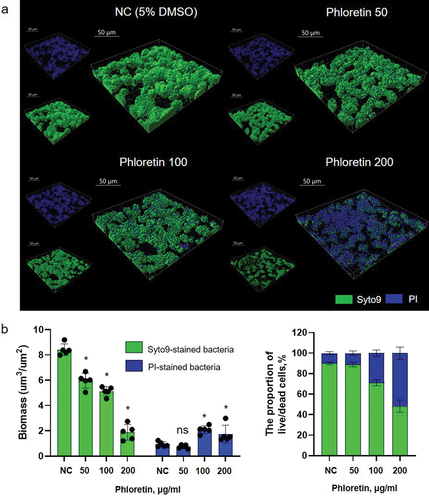
Phloretin inhibited EPS production in biofilm
To evaluate the effect of phloretin at concentrations of 50 μg/ml, 100 μg/ml and 200 μg/ml on biofilm structure and the biomass of bacteria and EPS, CLSM was used combined with a double-staining, where bacteria were labelled with green fluorescent SYTO 9 and EPS were stained with red-labelled fluorescent dextran. For the efficient image analysis, biofilms were cultured in an eight-well chambered borosilicate coverglass system for 24 h. Three-dimensional biofilm images indicated that, with increasing concentration, phloretin gradually reduced bacterial and EPS biomass. Treatment with 50 μg/ml and 100 μg/ml resulted in thinner and less compact biofilms, while 200 μg/ml prevented surface attachment of the majority of cells in comparison to thick biofilms grown under non-treated conditions (). Notably, at concentration 50 μg/ml and 100 μg/ml of phloretin, there was no substantial change in EPS/bacteria or WIG/WSG ratio between treated and non-treated conditions. However, at concentration 200 μg/ml, phloretin reduced EPS/bacteria ratio and slightly increased the proportion of WSG against WIG ().
Figure 4. Biofilm structure and composition affected by phloretin. (a) representative images of the double-labelled 24-h biofilms. Bacterial cells are shown in green and EPS in red. Three-dimensional reconstructions were performed with Imaris 9.0.0. (b) bacterial and EPS biomasses as calculated by Imaris. (c) EPS/bacteria biomass ratio as calculated by Imaris. (d) phloretin effect on biofilm-associated water-soluble and water insoluble glucan ratio, as measured by anthrone method. In all panels, bars represent the mean of five values calculated for arbitrary selected positions per sample. Error bars show standard deviation. NC, negative control (bacteria treated with 5% DMSO).
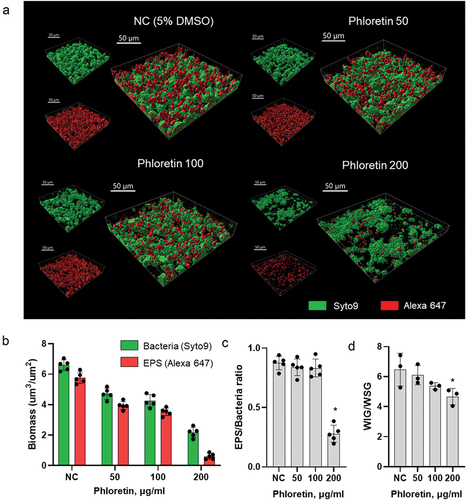
Phloretin modulated S. mutans lactic acid production and acid tolerance
To assess the effect of phloretin on S. mutans acidogenicity, lactic acid production in bacterial cultures that were treated with 50 μg/ml, 100 μg/ml, 200 μg/ml and 400 μg/ml of phloretin for 2 h was monitored. As shown in , phloretin reduced lactic acid production in a dose-dependent manner. Thus, 100 μg/ml of phloretin reduced lactic acid production by 50%, while 200 μg/ml and 400 μg/ml, concentrations used to prevent early and late biofilm formation, reduced up to 80% of lactic acid produced by S. mutans. Similarly, 200 μg/ml and 400 μg/ml of phloretin strongly inhibited S. mutans aciduricity (). The survival of bacterial cells in acidic environment was markedly reduced in the presence of phloretin compared to the control bacteria treated at a pH 7.2.
Figure 5. Effect of phloretin on lactic acid production and acid tolerance by S. mutans. (a) production of lactic acid by S. mutans treated with 50 μg/ml, 100 μg/ml, 200 μg/ml and 400 μg/ml of phloretin. (b) survival of S. mutans cells after 2 h of treatment with phloretin at pH 7.2 and pH 5.0 as measured by colony forming units (CFU/ml). Bars represent the mean of three biological replicates. Error bars show standard deviation. NC, negative control (bacteria treated with 5% DMSO).
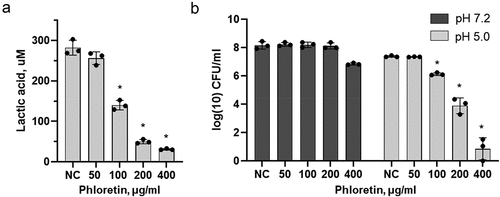
Phloretin reduced virulence gene expression of S. mutans
To further investigate the effect of phloretin on S. mutans, gene expression levels of three glycosyltransferases, gtfBCD, cell wall-anchored adhesins spaP, gbpC and their transpeptidases srtA as well as the main quorum sensing components, comED and luxS, were analysed. To this end, biofilms were treated with 200 μg/ml phloretin, the concentration at which phloretin had a pronounced effect on S. mutans viability, biofilm formation, and acid production and tolerance. The results () revealed a reduction in transcriptional levels of gtfB and gtfC, responsible for WIG production, but not gtfD responsible for WSG synthesis. The results also revealed that 200 μg/ml phloretin significantly reduced gene expression of tested cell wall-associated adhesins and their modifying enzyme SrtA, with the strongest effect on gbpC gene expression. Both comED and luxS quorum sensing systems were also significantly downregulated upon phloretin treatment.
Figure 6. Inhibitory effect of phloretin on the virulence gene expression levels in 24-hour biofilm. The relative expression levels were quantified by real-time PCR with 16S rRNA as an internal control. Black bar represents non-treated control, grey bars stand for biofilm treated with 200 μg/ml of phloretin. Bars represent the mean of three biological replicate. Error bars show standard deviation. NC, negative control (bacteria treated with 5% DMSO).
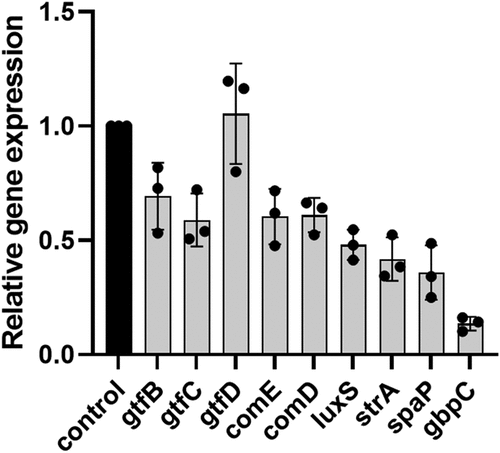
Discussion
Natural flavonoids represent an attractive selection of structurally and functionally diverse molecules with potent antibacterial and antibiofilm properties. Given their abundancy, low toxicity in human cells and pleiotropic effect on bacterial physiology, flavonoids seem to be prominent compounds for antibacterial and antibiofilm therapies in dental research. Thus, plant extracts and essential oils enriched in flavonoids as well as single purified compounds have demonstrated high efficacy against S. mutans biofilm formation and its virulence factors in multiple in vitro studies [Citation47–50]. Several flavonoids, e.g. apigenin, quercetin, naringenin and proanthocyanins, have been successfully used to reduce caries in animal studies [Citation30,Citation51,Citation52]. Finally, despite very limited and heterogeneous data from clinical investigations [Citation53], some studies have demonstrated an inhibitory effect of flavonoid-rich mouth rinse on dental plaque accumulation [Citation54]. However, more multidisciplinary research and clinically relevant studies are needed to explore the full potential of flavonoids in anticaries strategies.
In order to gain more knowledge on antibacterial mechanisms of flavonoids and to explore their structure-activity relationship against one of the main cariogenic bacterium, S. mutans, a flavonoid with a strong antimicrobial and antibiofilm properties against several pathogenic microbes, phloretin [Citation55], has been tested in this study. Phloretin is a dihydrochalcone, a natural plant-defence antioxidant compound found mainly in apples, pears, and strawberry fruit. Dihydrochalcones, similar to other flavonoids with antibacterial properties, act mainly by damaging cell membranes via direct interaction of their free hydroxyl groups with phospholipids and other biological molecules in bacterial membranes and cytoplasm [Citation56]. This correlates well with the documented antibacterial activity of phloretin against multiple Gram-positive bacteria including S. aureus, L. monocytogenes and S. typhimurium [Citation57]. In this study, phloretin showed a prominent concentration-dependent antibacterial, and, therefore, antibiofilm effect against S. mutans. At concentrations above 400 μg/ml, it completely diminished bacterial growth and viability in planktonic culture upon 24 h of incubation (, S1). CLSM also revealed the impact of phloretin on S. mutans viability in biofilm (). Staining of surface-associated S. mutans cells with two fluorescent nucleic acid stains, PI and SYTO 9 for dead and live cells, respectively, revealed that, in concentration-dependent manner, phloretin substantially reduced the proportion of live cells and increased the proportion of cells with damaged cellular membrane.
Besides their antimicrobial activity, many flavonoids specifically influence bacterial biofilm formation mechanisms. Thus, morin inhibits adhesion potential of S. mutans and S. aureus via direct inhibition of SrtA enzyme, a transpeptidase responsible for anchoring surface adhesins and virulence factors to the cell wall [Citation58,Citation59]. Many flavonoids such as apigenin, quercetin or their derivatives disturb EPS production and quorum sensing in many bacteria including S. aureus and E. coli [Citation60], and P. aeruginosa [Citation43] via direct interaction with effector proteins. In S. mutans, apigenin remains one of the most potent inhibitors of Gtfs enzymes [Citation29]. In addition to this, flavonoids could suppress gene expression of biofilm-associated virulence factors [Citation28,Citation61,Citation62]. The molecular mechanism of this regulation remains largely unknown; however, a recent study indicated that flavonoids can directly modulate the activity of transcriptional regulator from a two-component signal transduction system [Citation63]. This study revealed that in a dose-dependent mode, phloretin reduced S. mutans biofilm formation on various surfaces including sHA representing conditions similar to teeth in a natural oral environment (, Figure S2). The antibiofilm activity of phloretin, most probably, worked via the inhibition of bacterial growth and viability since a reduced number of planktonic cells limits biofilm formation. However, at a concentration of 200 μg/ml, it significantly reduced EPS-to-bacteria and WIG-to-WSG proportion, which indicates that phloretin could specifically target EPS synthesis. This was further corroborated with gene expression analysis where phloretin inhibited mRNA levels of gtfB and gtfC, glycosyltransferases responsible for WIG production, but not gtfD, responsible for WSG synthesis (). Additionally, its inhibitory effect on srtA, spaP and gbpC gene expression could contribute to the reduction in surface adhesion and interbacterial communication in biofilms.
Acidogenic and aciduric properties are among the most critical virulence factors of S. mutans. Its ability to metabolise dietary sugars to lactic acid and survive in low-pH environment promotes pathological changes in dental biofilms with dominance of acid-tolerant species and subsequent tooth enamel demineralization. Inhibition of S. mutans acidogenicity and aciduricity is one of the key aspects to reduce its virulence and to promote non-cariogenic oral biofilm. Phloretin tested in this study exhibited dose-dependent reduction of S. mutans acidogenicity and survival in acidic environment with the most prominent effect at 200 μg/ml and 400 μg/ml (). Essentially, its inhibitory effect was detected within a few hours of S. mutans exposure to phloretin and is not attributed to its bactericidal activity. Presumably, phloretin directly targeted lactate dehydrogenase (LDH) and other metabolic enzymes involved in stress tolerance, similarly to its effect on LDH, catalase and isocitrate dehydrogenase (IDH) of S. aureus [Citation57].
In a complex multispecies cariogenic biofilm, S. mutans employs its quorum sensing systems to modulate inter- and intraspecies communication and stress response. Thus, histidine kinase sensor protein ComD and cognate response regulator ComE, are critical for the density-dependent quorum sensing [Citation64], while LuxS-mediated signalling is essential for acid and oxidative stress tolerance in S. mutans biofilm [Citation15,Citation65]. The comD, comE and luxS genes were downregulated in biofilms treated with 200 μg/ml phloretin suggesting its interference with S. mutans quorum sensing ().
Although the exact mechanism of the antibacterial and antibiofilm effects of phloretin needs further investigation, the observation from the current study makes phloretin a promising candidate to be tested in conditions relevant to clinical practice. This study only explores the antibacterial and antibiofilm effects of phloretin on 24 h monospecies biofilm of S. mutans, in vitro. However, in vivo, cariogenic biofilm development depends on multiple components of oral microbiome. Hence, further studies of phloretin effect on multispecies dental biofilms and, in particular, on commensal oral bacteria are required to elucidate the pharmacological potential of phloretin for oral health maintenance. It is worth noting that flavonoid effects could be species-specific [Citation42]. Thus, the reciprocal impact of phloretin on the biofilm formation of pathogenic and commensal E. coli strains has been previously described [Citation44]. Furthermore, to be a successful adjunct to oral hygiene, phloretin can be tested in a dynamic environment with shorter exposure time (a few minutes) as well as in combination with other agents, e.g. fluoride, other flavonoids or probiotics, to enhance their cariostatic properties without increasing exposure parameter.
Despite a long history of flavonoid investigation in dental research, their introduction into the therapeutic products is subject to several limitations and challenges such as low compound solubility in aqueous medium or reduced substantivity due to a very dynamic environment in oral cavity [Citation66]. However, recent progress in molecular chemistry and material science has provided attractive solutions to increase flavonoid solubility and to improve controlled drug delivery systems. Thus, microparticles, films and oral tablets with natural flavonol morin have been recently tested to increase its water solubility and improve drug release kinetics [Citation67,Citation68]. Different types of nanoparticles are currently being tested to improve the bioavailability of quercetin against oral diseases [Citation69]. Finally, flavonoid incorporation, e.g. apigenin, into dental composites, is tested to modulate cariogenic bacteria virulence [Citation70].
Conclusions
In conclusion, natural flavonoids retain a large potential as effective antimicrobial and antibiofilm agents for oral science. Phloretin, tested in this study, showed a prominent inhibitory effect against S. mutans virulence, mainly by compromising its viability, acidogenicity and acid tolerance. These observations make phloretin a promising candidate to test in more clinically relevant systems including specific oral healthcare products ranging from mouth rinses to toothpastes for caries preventive therapies.
Author contributions
Conception and design, V.S.; data curation, analysis and interpretation, LR and VS; original draft preparation, review and editing, V.S., L.R. and M.M.B., project administration and supervision, V.S. and M.M.B. All authors have read and agreed to the published version of the manuscript.
Supplemental Material
Download MS Word (1.3 MB)Acknowledgments
We thank the Nano Imaging Lab, SNI, University of Basel, and the Imaging Core Facility of the Biozentrum, University of Basel, for their support with SEM and CLSM analysis. We also thank Hedwig Wariwoda for excellent technical support and all members of the Department of Research, University Center for Dental Medicine Basel UZB, for insightful discussions.
Disclosure statement
No potential conflict of interest was reported by the author(s).
Supplementary material
Supplemental data for this article can be accessed online at https://doi.org/10.1080/20002297.2023.2230711
Additional information
Funding
References
- Theo Vos CA, Arora M, Barber RM, et al. Global, regional, and national incidence, prevalence, and years lived with disability for 310 diseases and injuries, 1990-2015: a systematic analysis for the Global Burden of Disease Study 2015. Lancet. 2016;388(10053):1545–13. doi: 10.1016/S0140-6736(16)31678-6
- Pitts NB, Zero DT, Marsh PD, et al. Dental caries. Nat Rev Dis Primers. 2017;3(1):17030. doi: 10.1038/nrdp.2017.30
- Marsh PD, Zaura E. Dental biofilm: ecological interactions in health and disease. J Clin Periodontol. 2017;44(Suppl 18):S12–S22. doi: 10.1111/jcpe.12679
- Bowen WH, Burne RA, Wu H, et al. Oral biofilms: pathogens, matrix, and polymicrobial interactions in microenvironments. Trends Microbiol. 2018;26(3):229–242. doi: 10.1016/j.tim.2017.09.008
- Zeng L, Burne RA. Comprehensive mutational analysis of sucrose-metabolizing pathways in Streptococcus mutans reveals novel roles for the sucrose phosphotransferase system permease. J Bacteriol. 2013;195(4):833–843. doi: 10.1128/JB.02042-12
- Jakubovics NS, Goodman SD, Mashburn‐Warren L, et al. The dental plaque biofilm matrix. Periodontol. 2000;86(1):32–56. 2021. doi: 10.1111/prd.12361
- Karygianni L, Ren Z, Koo H, et al. Biofilm matrixome: extracellular components in structured microbial communities. Trends Microbiol. 2020;28(8):668–681. doi: 10.1016/j.tim.2020.03.016
- Banas JA, Vickerman MM. Glucan-binding proteins of the oral streptococci. Crit Rev Oral Biol Med. 2003;14(2):89–99. doi: 10.1177/154411130301400203
- Jenkinson HF, Demuth DR. Structure, function and immunogenicity of streptococcal antigen I/II polypeptides. Mol Microbiol. 1997;23(2):183–190. doi: 10.1046/j.1365-2958.1997.2021577.x
- Lemos JA, Palmer SR, Zeng L, et al. The biology of Streptococcus mutans. Microbiol Spectr. 2019;7(1): doi: 10.1128/microbiolspec.GPP3-0051-2018
- Matsui R, Cvitkovitch D. Acid tolerance mechanisms utilized by Streptococcus mutans. Future Microbiol. 2010;5(3):403–417. doi: 10.2217/fmb.09.129
- Quivey RG Jr., Kuhnert WL, Hahn K. Adaptation of oral streptococci to low pH. Adv Microb Physiol. 2000;42:239–274.
- Li YH, Hanna MN, Svensäter G, et al. Cell density modulates acid adaptation in Streptococcus mutans: implications for survival in biofilms. J Bacteriol. 2001;183(23):6875–6884. doi: 10.1128/JB.183.23.6875-6884.2001
- Merritt J, Qi F, Goodman SD, et al. Mutation of luxS affects biofilm formation in Streptococcus mutans. Infect Immun. 2003;71(4):1972–1979. doi: 10.1128/IAI.71.4.1972-1979.2003
- Wen ZT, Burne RA. LuxS-mediated signaling in Streptococcus mutans is involved in regulation of acid and oxidative stress tolerance and biofilm formation. J Bacteriol. 2004;186(9):2682–2691. doi: 10.1128/JB.186.9.2682-2691.2004
- Jones CG. Chlorhexidine: is it still the gold standard? Periodontol. 2000;15(1):55–62. doi: 10.1111/j.1600-0757.1997.tb00105.x 1997.
- Song W, Ge S. Application of Antimicrobial Nanoparticles in Dentistry. Molecules. 2019;24(6):1033. doi: 10.3390/molecules24061033
- Besinis A, De Peralta T, Handy RD. The antibacterial effects of silver, titanium dioxide and silica dioxide nanoparticles compared to the dental disinfectant chlorhexidine on Streptococcus mutans using a suite of bioassays. Nanotoxicology. 2014;8(1):1–16. doi: 10.3109/17435390.2012.742935
- Mai J, Tian X-L, Gallant JW, et al. A novel target-specific, salt-resistant antimicrobial peptide against the cariogenic pathogen Streptococcus mutans. Antimicrob Agents Chemother. 2011;55(11):5205–5213. doi: 10.1128/AAC.05175-11
- Fruh R, Anderson A, Cieplik F, et al. Antibiotic resistance of selected bacteria after treatment of the supragingival biofilm with subinhibitory chlorhexidine concentrations. Antibiotics (Basel). 2022;11(10):1420. doi: 10.3390/antibiotics11101420
- Di Giulio M, Di Bartolomeo S, Di Campli E, et al. The effect of a silver nanoparticle polysaccharide system on streptococcal and saliva-derived biofilms. Int J Mol Sci. 2013;14(7):13615–13625. doi: 10.3390/ijms140713615
- Coppo E, Marchese A. Antibacterial activity of polyphenols. Curr Pharm Biotechnol. 2014;15(4):380–390. doi: 10.2174/138920101504140825121142
- Silva LN, Zimmer KR, Macedo AJ, et al. Plant natural products targeting bacterial virulence factors. Chem Rev. 2016;116(16):9162–9236. doi: 10.1021/acs.chemrev.6b00184
- Harborne JB, Williams CA. Advances in flavonoid research since 1992. Phytochemistry. 2000;55(6):481–504. doi: 10.1016/S0031-9422(00)00235-1
- Linke HA, LeGeros RZ. Black tea extract and dental caries formation in hamsters. Int J Food Sci Nutr. 2003;54(1):89–95. doi: 10.1080/0963748031000062029
- Touyz LZ, Amsel R. Anticariogenic effects of black tea (Camellia sinensis) in caries prone-rats. Quintessence Int.2001;32(8):647–650.
- Hirasawa M, Takada K, Otake S. Inhibition of acid production in dental plaque bacteria by green tea catechins. Caries Res. 2006;40(3):265–270. doi: 10.1159/000092236
- Koo H, Seils J, Abranches J, et al. Influence of apigenin on gtf gene expression in Streptococcus mutans UA159. Antimicrob Agents Chemother. 2006;50(2):542–546. doi: 10.1128/AAC.50.2.542-546.2006
- Koo H, Hayacibara MF, Schobel BD, et al. Inhibition of Streptococcus mutans biofilm accumulation and polysaccharide production by apigenin and tt-farnesol. J Antimicrob Chemother. 2003;52(5):782–789. doi: 10.1093/jac/dkg449
- Koo H, Pearson SK, Scott-Anne K, et al. Effects of apigenin and tt-farnesol on glucosyltransferase activity, biofilm viability and caries development in rats. Oral Microbiol Immunol. 2002;17(6):337–343. doi: 10.1034/j.1399-302X.2002.170602.x
- Koo H, Schobel B, Scott-Anne K, et al. Apigenin and tt-farnesol with fluoride effects on S. mutans biofilms and dental caries. J Dent Res. 2005;84(11):1016–1020. doi: 10.1177/154405910508401109
- Liu Y, HAN L, YANG H, et al. Effect of apigenin on surface-associated characteristics and adherence of Streptococcus mutans. Dent Mater J. 2020;39(6):933–940. doi: 10.4012/dmj.2019-255
- Zeng Y, Nikitkova A, Abdelsalam H, et al. Activity of quercetin and kaemferol against Streptococcus mutans biofilm. Arch Oral Biol. 2019;98:9–16. doi:10.1016/j.archoralbio.2018.11.005
- Yue J, Yang H, Liu S, et al. Influence of naringenin on the biofilm formation of Streptococcus mutans. J Dent. 2018;76:24–31. doi:10.1016/j.jdent.2018.04.013
- Falsetta ML, Klein MI, Lemos JA, et al. Novel antibiofilm chemotherapy targets exopolysaccharide synthesis and stress tolerance in Streptococcus mutans to modulate virulence expression in vivo. Antimicrob Agents Chemother. 2012;56(12):6201–6211. doi: 10.1128/AAC.01381-12
- Jeon JG, Klein MI, Xiao J, et al. Influences of naturally occurring agents in combination with fluoride on gene expression and structural organization of Streptococcus mutans in biofilms. BMC Microbiol. 2009;9(1):228. doi: 10.1186/1471-2180-9-228
- Hu P, Lv B, Yang K, et al. Discovery of myricetin as an inhibitor against Streptococcus mutans and an anti-adhesion approach to biofilm formation. Int J Med Microbiol. 2021;311(4):151512. doi: 10.1016/j.ijmm.2021.151512
- Kim D, Hwang G, Liu Y, et al. Cranberry flavonoids modulate cariogenic properties of mixed-species biofilm through exopolysaccharides-matrix disruption. PLoS One. 2015;10(12):e0145844. doi: 10.1371/journal.pone.0145844
- Shafiei Z, Rahim ZHA, Philip K, et al. Potential effects of Psidium sp., Mangifera sp., Mentha sp. and its mixture (PEM) in reducing bacterial populations in biofilms, adherence and acid production of S. sanguinis and S. mutans. Arch Oral Biol. 2020;109:104554. doi:10.1016/j.archoralbio.2019.104554
- de Farias AL, Arbeláez MIA, Meneguin AB, et al. Mucoadhesive controlled-release formulations containing morin for the control of oral biofilms. Biofouling. 2022;38(1):71–83. doi: 10.1080/08927014.2021.2015580
- Gutierrez-Venegas G, Gómez-Mora JA, Meraz-Rodríguez MA, et al. Effect of flavonoids on antimicrobial activity of microorganisms present in dental plaque. Heliyon. 2019;5(12):e03013. doi: 10.1016/j.heliyon.2019.e03013
- Pruteanu M, Hernández Lobato JI, Stach T, et al. Common plant flavonoids prevent the assembly of amyloid curli fibres and can interfere with bacterial biofilm formation. Environ Microbiol. 2020;22(12):5280–5299. doi: 10.1111/1462-2920.15216
- Paczkowski JE, Mukherjee S, McCready AR, et al. Flavonoids suppress Pseudomonas aeruginosa virulence through allosteric inhibition of quorum-sensing receptors. J Biol Chem. 2017;292(10):4064–4076. doi: 10.1074/jbc.M116.770552
- Lee JH, Regmi SC, Kim J-A, et al. Apple flavonoid phloretin inhibits Escherichia coli O157: h7 biofilm formation and ameliorates colon inflammation in rats. Infect Immun. 2011;79(12):4819–4827. doi: 10.1128/IAI.05580-11
- Adil M, Baig MH, Rupasinghe HPV. Impact of citral and phloretin, alone and in combination, on major virulence traits of Streptococcus pyogenes. Molecules. 2019;24(23):4237. doi: 10.3390/molecules24234237
- Wu J, Jiang X, Yang Q, et al. Inhibition of Streptococcus mutans biofilm formation by the joint action of oxyresveratrol and Lactobacillus casei. Appl Environ Microbiol. 2022;88(9):e0243621. doi: 10.1128/aem.02436-21
- Mandava K, Batchu UR, Kakulavaram S, et al. Design and study of anticaries effect of different medicinal plants against S. mutans glucosyltransferase. BMC Complement Altern Med. 2019;19(1):197. doi: 10.1186/s12906-019-2608-3
- Matsumoto M, Minami T, Sasaki H, et al. Inhibitory effects of oolong tea extract on caries–inducing properties of mutans Streptococci. Caries Res. 1999;33(6):441–445. doi: 10.1159/000016549
- Smullen J, Koutsou GA, Foster HA, et al. The antibacterial activity of plant extracts containing polyphenols against Streptococcus mutans. Caries Res. 2007;41(5):342–349. doi: 10.1159/000104791
- Sato M, Tanaka H, Fujiwara S, et al. Antibacterial property of isoflavonoids isolated from Erythrina variegata against cariogenic oral bacteria. Phytomedicine. 2003;10(5):427–433. doi: 10.1078/0944-7113-00225
- Wood N. The effects of selected dietary bioflavonoid supplementation on dental caries in young rats fed a high-sucrose diet. J Med Food. 2007;10(4):694–701. doi: 10.1089/jmf.2007.412
- Koo H, Duarte S, Murata RM, et al. Influence of cranberry proanthocyanidins on formation of biofilms by Streptococcus mutans on Saliva-coated apatitic surface and on dental caries development in vivo. Caries Res. 2010;44(2):116–126. doi: 10.1159/000296306
- Janakiram C, Venkitachalam R, Fontelo P, et al. Effectiveness of herbal oral care products in reducing dental plaque & gingivitis – a systematic review and meta-analysis. BMC Complement Med Ther. 2020;20(1):43. doi: 10.1186/s12906-020-2812-1
- Koo H, Cury JA, Rosalen PL, et al. Effect of a mouthrinse containing selected propolis on 3-day dental plaque accumulation and polysaccharide formation. Caries Res. 2002;36(6):445–448. doi: 10.1159/000066535
- Liu N, Zhang N, Zhang S, et al. Phloretin inhibited the pathogenicity and virulence factors against Candida albicans. Bioengineered. 2021;12(1):2420–2431. doi: 10.1080/21655979.2021.1933824
- Kroliczewsk IGRBJ, Bartoszewski R, Króliczewski J. Comprehensive review of antimicrobial activities of plant flavonoids. Phytochem Rev. 2019;18(1):241–272. doi: 10.1007/s11101-018-9591-z
- Barreca D, Bellocco E, Laganà G, et al. Biochemical and antimicrobial activity of phloretin and its glycosilated derivatives present in apple and kumquat. Food Chem. 2014;160:292–297. doi:10.1016/j.foodchem.2014.03.118
- Huang P, Hu P, Zhou SY, et al. Morin inhibits sortase a and subsequent biofilm formation in Streptococcus mutans. Curr Microbiol. 2014;68(1):47–52. doi: 10.1007/s00284-013-0439-x
- Kang SS, Kim J-G, Lee T-H, et al. Flavonols inhibit sortases and sortase-mediated Staphylococcus aureus clumping to fibrinogen. Biol Pharm Bull. 2006;29(8):1751–1755. doi: 10.1248/bpb.29.1751
- Pei ZJ, Li C, Dai W, et al. The anti-biofilm activity and mechanism of apigenin-7-o-glucoside against Staphylococcus aureus and Escherichia coli. Infect Drug Resist. 2023;16:2129–2140. doi:10.2147/IDR.S387157
- Wang Z, Ding Z, Li Z, et al. Antioxidant and antibacterial study of 10 flavonoids revealed rutin as a potential antibiofilm agent in Klebsiella pneumoniae strains isolated from hospitalized patients. Microb Pathog. 2021;159:105121. doi:10.1016/j.micpath.2021.105121
- Vazquez-Armenta FJ, Hernandez-Oñate MA, Martinez-Tellez MA, et al. Quercetin repressed the stress response factor (sigB) and virulence genes (prfA, actA, inlA, and inlC), lower the adhesion, and biofilm development of L. monocytogenes. Food Microbiol. 2020;87:103377. doi:10.1016/j.fm.2019.103377
- Raorane CJ, Lee J-H, Kim Y-G, et al. Antibiofilm and antivirulence efficacies of flavonoids and curcumin against Acinetobacter baumannii. Front Microbiol. 2019;10:990. doi:10.3389/fmicb.2019.00990
- Senadheera D, Cvitkovitch DG. Quorum sensing and biofilm formation by Streptococcus mutans. Adv Exp Med Biol. 2008;631:178–188.
- Huang Z, Meric, G, Liu, Z, et al. luxS-based quorum-sensing signaling affects biofilm formation in Streptococcus mutans. J Mol Microbiol Biotechnol. 2009;17(1):12–19. doi: 10.1159/000159193
- Jeon JG, Rosalen PL, Falsetta ML, et al. Natural products in caries research: current (limited) knowledge, challenges and future perspective. Caries Res. 2011;45(3):243–263. doi: 10.1159/000327250
- Sales LS, Gimenes MDS, Meneguin AB, et al. Development of multiparticulate systems based on natural polymers for morin controlled release. Int j biol macromol. 2023;228:1–12. doi:10.1016/j.ijbiomac.2022.12.146
- de Farias AL, Meneguin AB, da Silva Barud H, et al. The role of sodium alginate and gellan gum in the design of new drug delivery systems intended for antibiofilm activity of morin. Int j biol macromol. 2020;162:1944–1958. doi:10.1016/j.ijbiomac.2020.08.078
- Pourhajibagher M, Alaeddini M, Etemad-Moghadam S, et al. Quorum quenching of Streptococcus mutans via the nano-quercetin-based antimicrobial photodynamic therapy as a potential target for cariogenic biofilm. BMC Microbiol. 2022;22(1):125. doi: 10.1186/s12866-022-02544-8
- Andre CB, Rosalen PL, Giannini M, et al. Incorporation of apigenin and tt-farnesol into dental composites to modulate the Streptococcus mutans virulence. Dent Mater. 2021;37(4):e201–e212. doi: 10.1016/j.dental.2020.12.005