ABSTRACT
Cells naïve to stress can display the effects of stress, such as DNA damage and apoptosis, when they are exposed to signals from stressed cells; this phenomenon is known as the bystander effect. We previously showed that bystander effect induced by ionising radiation are mediated by extracellular vesicles (EVs). Bystander effect can also be induced by other types of stress, including heat shock, but it is unclear whether EVs are involved. Here we show that EVs released from heat shocked cells are also able to induce bystander damage in unstressed populations. Naïve cells treated with media conditioned by heat shocked cells showed higher levels of DNA damage and apoptosis than cells treated with media from control cells. Treating naïve cells with EVs derived from media conditioned by heat shocked cells also induced a bystander effect when compared to control, with DNA damage and apoptosis increasing whilst the level of cell viability was reduced. We demonstrate that treatment of naïve cells with heat shocked cell-derived EVs leads to greater invasiveness in a trans-well Matrigel assay. Finally, we show that naïve cells treated with EVs from heat-shocked cells are more likely to survive a subsequent heat shock, suggesting that these EVs mediate an adaptive response. We propose that EVs released following stress mediate an intercellular response that leads to apparent stress in neighbouring cells but also greater robustness in the face of a subsequent insult.
Responsible Editor Giovanni Camussi, Universita degli Studi di Torino, Italy
Introduction
Cells naïve to stress can exhibit DNA damage as a consequence of signal transmission from neighbouring stressed cells [Citation1–Citation3], a phenomenon referred to as a bystander effect (BE). BE was first observed when irradiated populations showed higher levels of damaged cells than were expected to have been traversed by alpha particles [Citation4–Citation6]. To date, most BE research has focused on post-irradiation effects, but there is growing evidence of other stress treatments inducing damage in bystander populations including chemical and heat stresses [Citation7,Citation8]. Thermal BE has been observed after both lethal and sub-lethal levels of heat [Citation8–Citation10]. Whilst inhibition of gap junction signalling has been shown to abrogate the levels of bystander damage [Citation11,Citation12] media extracted from irradiated cells is sufficient to drive bystander damage in naïve populations [Citation2,Citation13,Citation14], suggesting that a secreted factor released into the culture media is sufficient for BE induction. The BE is not limited to cell culture models; animal studies have shown that after localised irradiation, damage is found at distant sites [Citation15,Citation16], suggesting that the causal signal is stable, and can withstand distribution via the circulatory system. Recent evidence from in vitro experiments show that extracellular vesicles (EVs) released by irradiated cells can induce BE [Citation17–Citation19], possibly by RNA transfer [Citation18,Citation20].
EVs are lipid-encased compartments released by cells into their surrounding environment. There are three main classes of EVs: apoptotic bodies, formed during programmed cell death, microvesicles, formed by budding from the plasma membrane, and exosomes, small EVs of an endocytic origin [Citation21–Citation25]. They are known to act as signalling molecule complexes and are released from many different cell types [Citation26,Citation27]. EVs have previously been linked with BE [Citation17,Citation18,Citation20] and have been shown to traffic various different biologically active molecules, including proteins, lipids and RNA [Citation28]. However, it is not known whether EVs also mediate BE induced by stresses other than ionising radiation.
Here we demonstrate that EVs released by heat shocked cells are sufficient to induce thermal BE, with an increase in apoptosis and DNA damage, and a reduction in cell viability observed in recipient cells. Bystander cells were also shown to become more resistant to subsequent stress and more invasive after exposure to heat shock cell derived EVs. These results suggest an adaptive role for EVs in the stress response. Our experiments shed new light on the role of EVs in intercellular communication during stress.
Methods
Cell culture
Cells were cultured in medium (MCF7 and HeLa: DMEM, SLS; K562: RPMI, Fisher, 10759263) supplemented with 2mM l-glutamine (Fisher, 12319762) and 10% (v/v) foetal calf serum (Fisher, 10500064). All cells were propagated in humidified incubator maintained at 5% CO2 and 37°C. For experiments cells were seeded the day prior to treatment at 3.5 × 104 cells per cm3 of growth surface. For EV extractions cells were grown in medium supplemented with pre-cleared (by ultracentrifugation for 16 h at 100,000g) FCS following treatment.
Heat shock conditions
Heat shock was performed by moving the culture flasks from the 37°C incubator to an incubator pre-warmed to 45°C. The MCF7 cells were then left at this temperature for 1 h (MCF7 and HeLa cells) or 3 h (K562 cells) before being returned to the 37°C incubator. Control cells were maintained at 37°C.
EV isolation
Media was removed from cells 24 h after heat shocking or control treatment (maintenance at 37°C) and centrifuged at 300g for 5 min to remove suspended cells. The supernatant was cleared of cell debris and larger vesicles by centrifugation at 16,500g for 20 min, the pellet was discarded and the supernatant was filtered through 0.22 µm filters that had been blocked with 0.01% BSA. EVs were pelleted by ultracentrifugation at 100,000g for 90 min at 4°C. For volumes below 16 ml, the SW32Ti rotor (Beckman Coulter κ-factor 204) was used; for larger volumes the 70Ti rotor (Beckman Coulter κ-factor 44) was used. EVs were washed by re-suspending the pellet in PBS and repeating the ultracentrifugation procedure. Isolated EVs were re-suspended in PBS for further use.
BE experiments
Filtered media or EVs were extracted as above and added to cells seeded the day prior to treatment. These cells were then incubated at 37°C for 24 h before the cells were harvested for analysis.
Comet assay
Comet assays were performed as previously described [Citation29]. Briefly, 20,000 cells were embedded in low melting point agarose (fisher, BP165-25G) on slides pre-coated with normal melting point agarose (Sigma-Aldrich, A9539-25G). The cells were lysed in a pH10 lysis buffer overnight and DNA unwound in alkaline electrophoresis buffer for 40 min, before the slides were electrophoresed at 1 V/cm for 30 min. Slides were washed and stained with 1x Sybr Gold (Invitrogen: S11494). When dry, photos were taken using a ZEISS Axio Imager 2 and damage was analysed using the comet analysis software CASP [Citation30]. Two slides were prepared and analysed for each biological replicate.
MTT (3-(4,5-dimethylthiazol-2-yl)-2,5-diphenyltetrazolium bromide) assay
The MTT assay was performed by adding 100 μl MTT solution at 4 mg/ml in PBS (Sigma-Aldrich, M5655-1G) to 100 μl of media in each well for a final concentration of 2 mg/ml. The plates were then incubated for 3 h at 37°C. After incubation both the MTT solution and media were carefully removed from each well and 100 μl of MTT solvent (4mM HCl, 0.1% IGEPAL® CA-630 in isopropanol) was added. The absorbance at wavelength 595 nm of each well was then recorded using a plate reader.
Apoptosis assay
Cell suspensions were centrifuged at 300g for 5 min and the cells were fixed with 1:3 acetic acid: methanol and maintained at room temperature for 15 min. The fixed cells were dropped onto slides and allowed to dry before 30 μl of DAPI (Fisher, VXP36931) was added. At least 200 cells were counted under a microscope (Carl Zeiss Axioplan) at 63× magnification and the number of cells displaying nuclear fragmentation (indicative of late apoptosis) was recorded.
Transmission electron microscopy
EV samples were fixed with 4% paraformaldehyde (Sigma-Aldrich) on ice for 15 min. Carbon-formvar coated copper 300 mesh grids (Agar Scientific, Stanstead) were in contact with the sample for 30 min. Grids were then washed three times using filtered ultrapure water and finally stained with 2% uranyl acetate (Sigma-Aldrich) and then left to air dry for 60 min. Grids were visualised using Hitachi H7650 Transmission Electron Microscope at 100 kV at 40,000× magnification. EV analysis was carried out using Advanced Microscopy Techniques software (Advanced Microscopy Techniques, Massachusetts, USA).
Western blots
Cell pellets were lysed in 1x radioimmunoprecipitation assay (RIPA) buffer under agitation for 30 min at 4°C. Cell debris and larger vesicles were removed and discarded by centrifugation at 14,000g for 20 min at 4°C. EV pellets were re-suspended in 50 µl 1x RIPA buffer and sonicated three times for 5 min at 30% amplitude in ice cold water. To extract the protein, EV samples were centrifuged at 14,000g for 20 min at 4°C. Protein was quantified using bicinchoninic acid assay kit (Life Technologies). Ten micrograms of protein were run on 12% precast acrylamide gels (BioRad) and transferred onto polyvinylidene difluoride membrane (BioRad). Membranes were blocked using 5% marvel in Tris-buffered saline with 0.05% Tween 20 followed by overnight incubation at 4°C with rabbit or mouse antihuman primary antibodies (Abcam) specific to HSP70 (ab5439) (exosome marker), TSG101 (ab83) (EV marker), cytochrome C oxidase (ab150422) (apoptotic body/mitochondrial marker), GAPDH (ab128915) (cytoplasmic marker) and calnexin (ab22595) (endoplasmic reticulum marker). Secondary Cy3 or horseradish peroxidase (HRP) tagged antibody (Abcam) incubations were then performed for 60 min at room temperature. Membranes were read using ChemiDoc MP (BioRad)
Matrigel assay
Cells were treated with heat shocked cell derived EVs and starved of serum for 24 h prior to seeding in trans-well inserts. Cells need to be starved sufficiently so that the complete media in the lower chamber will act as a chemoattractant for the cells. The cells were harvested by centrifugation (300g for 5 min). These cells were then seeded in Matrigel-coated 8.0 µm pore membrane trans-well inserts (BD Biosciences) at 100,000 cells/well. EVs isolated from heat shocked cells were added to the inserts immediately after cell seeding. Complete media was loaded into receiver wells (24-well plate) and cells were incubated for 24 h. The upper surface of the Matrigel was swabbed to remove any cells that had not invaded. Inserts and receiver wells were washed with PBS, and cells that had invaded were stained with 1% crystal violet (Sigma-Aldrich) for 10 min. Membranes were washed and mounted onto glass slides using di-n-butyl phthalate in xylene. Membranes were visualised under Zeiss Axioplan microscope at 125× magnification.
Nanoparticle tracking analysis
EV size and concentration were measured by nanoparticle tracking analysis (NTA) using a NanoSight LM10 with a laser wavelength of 642 nm and the NTA 2.3 build 0033 analytical software (Malvern Instruments Ltd, Malvern). Five 30 s videos were recorded for each sample and the software was used to estimate concentration and size of the EVs. Samples were diluted in PBS. The recording took place at room temperature which was monitored manually. Camera gain was 350 and the shutter speed was 14.99 ms. For analysis the detection threshold was set to 10 and the type to multi. The blur, min track length and min expected particle size were all set to auto. Calibration was carried out using 100 nm silica beads diluted to a known concentration in PBS and then five 30 s videos were recorded.
Statistics
Statistical significance for the comet assay data was tested using the non-parametric Mann-Whitney U test [Citation31]. For apoptosis assays, Western blot, NTA and Matrigel invasion assay data, statistical significance was calculated using the Student’s t-test. Statistical significance for apoptosis assays was calculated using the χ2 test, with the numbers of normal and apoptotic control cells used to calculate the expected ratio.
Results
Heat shock induces DNA damage and apoptosis and reduces cell viability in MCF7 cells
In order to test the effects of direct stress treatment MCF7 cells were incubated for 1 h at 45°C or 37°C (cells maintained at 37°C were considered the control experiment). After 24 h the levels of HSP70 protein, DNA damage and cell viability were assayed. The level of HSP70 in these cells was significantly higher than in control cells, confirming that the heat shock response had been activated (–)). As expected, DNA damage was higher in the heat shocked cells compared with those maintained at 37°C (), whilst cell viability was reduced (). These results confirm that the heat stress conditions were able to induce a stress response in directly treated cells.
Figure 1. Heat treatment induces a stress response in directly treated cells. MCF7 cells were heat shocked at 45°C (HS) control treated at 37°C (Ctrl) for 1 h and then maintained at 37°C for 24 h. The levels of HSP70 and GAPDH were assayed after heat shock (a) via western blotting (see Supplementary figure 4 for full blots) and the ratio of HSP70 to GAPDH was calculated (b). Error bars represent standard error of the mean for three biological replicates. The levels of DNA damage (c), cell viability (d) and apoptosis (e) in treated cells were measured using the comet assay, the MTT assay and the apoptosis assay, respectively. For comet assays at least 500 cells were counted across two biological replicates. Box and whisker plots show percentage of DNA in the comet tail, median, upper and lower quartiles, error bars are 1.5× interquartile range. For MTTs absorbance at 595 nm in 30 wells containing treated cells was measured. Box and whisker plots show absorbance at 595 nm, median, upper and lower quartiles, error bars are 1.5× interquartile range. For apoptosis assays at least 1000 cells were counted across two biological replicates, error bars represent standard deviation. *p < 0.05, **p < 0.01 and ***p < 0.001.
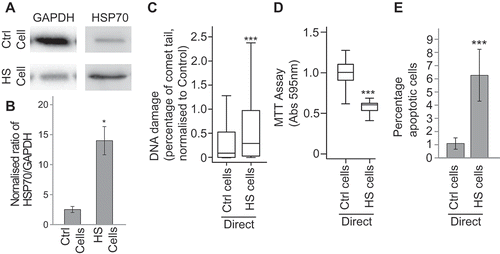
BE can BE induced using media extracted from heat shocked cells
To confirm whether BE could be induced by signals released from cells, we performed media transfer experiments. MCF7 cells were heat shocked at 45°C for 1 h and after 24 h the conditioned media (CM) was removed, filtered and then placed onto naïve MCF7 cells. The cells were then grown for 24 h before harvesting. The levels of DNA damage and apoptosis were measured using the comet and apoptosis assay, respectively (–). DNA damage (, p < 0.001) and apoptosis (, p < 0.001, χ2) increased in bystander cells treated with CM from heat shocked cells compared with those treated with media conditioned by control cells. Similar results were observed in HeLa and K562 cells (Supplementary figure 1). These data confirm prior work on active thermal BE [Citation9,Citation10], showing that media taken from heat shocked cells is able to induce BE in naïve cell populations.
Figure 2. Heat shock induces DNA damage and apoptosis which can be transferred to bystander cells MCF7 cells were heat shocked at 45°C (HS) or kept at the control temperature of 37°C (Ctrl) for one hour and then maintained at 37°C for 24 h. Conditioned media (CM) was removed from cells and placed onto naïve MCF7 cells. Twenty-four hours later, the level of DNA damage (a) and apoptosis (b) was measured in the bystander cells. For comet assays a total of at least 140 cells were counted across two biological replicates. Box and whisker plots show percentage of DNA in the comet tail, median, upper and lower quartiles, error bars are 1.5× interquartile range. For apoptosis assays at least 1000 cells were counted across two biological replicates, error bars represent standard deviation. *p < 0.05, **p < 0.01 and ***p < 0.001.
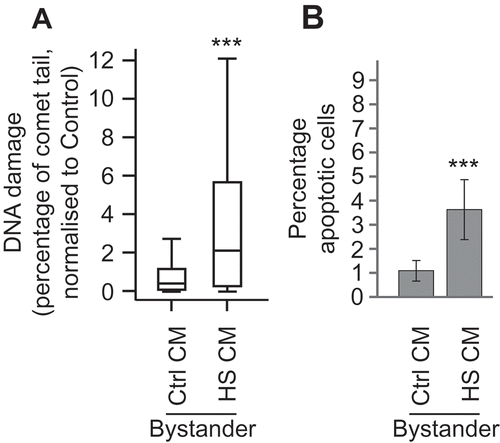
EVs released by stressed cells are able to induce BE
Having shown that heat-shock induces BE, we next wished to test whether EVs were involved. Our previous work has shown that EVs released from irradiated cells are capable of inducing BE in naïve populations [Citation17,Citation18]. In order to test whether the EV component of CM can induce BE in naïve cell populations, EVs were extracted from media conditioned for 24 h by heat shocked or control treated MCF7 cells. Transmission electron microscopy (TEM) images confirm the presence of EV-like structures (). Western blotting confirmed the presence of TSG101, HSP70 and GAPDH in EVs from heat shocked and control cells and absence of the endoplasmic reticulum protein calnexin and the mitochondrial marker cytochrome C, suggesting that the EVs are not contaminated by cellular debris and are not apoptotic bodies (). The electron microscopy and western blot images are therefore consistent with the successful isolation of EVs. Quantification of EV secretion concentrations after heat shocking or control treatment showed that there was no significant change in the quantity of EVs released following heat stress (). Measurement of the size of EVs using electron microscopy revealed that the vesicles released from cells after heat shocking are significantly smaller in diameter than those released at the control temperature (). To determine whether EVs isolated from heat shocked cells were able to induce BE in naïve cells, EVs were collected from the CM of heat shocked cells and control cells and transferred to naïve cells which were then maintained at 37°C for 24 h. After this culture period, the EV treated cells were tested for DNA damage, apoptosis and viability (using the comet assay, apoptosis assay and MTT assay, respectively). Bystander cells treated with EVs extracted from heat shocked cells showed significantly higher levels of DNA damage and apoptosis and significantly lower levels of overall cell survival than cells treated with EVs extracted from cells grown at 37°C (–)). Cells were also treated with the supernatant from the first 100,000g spin but there was no significant difference in the levels of apoptosis between cells treated with the supernatant from heat or mock shocked cells suggesting the supernatant is unable to induce BE (Supplementary figure 2(a)). Similar data demonstrating EV-induced BE were also shown in K562 and HeLa cells (Supplementary figure 2(b–c)). Taken together these data show that the EVs released by heat-shocked cells can induce BE in naïve cells.
Figure 3. EVs mediate heat shock-induced BE (a) Representative TEM images of EVs extracted from heat shocked or control MCF7 cells. (b) Western blot of cellular or EV protein (see Supplementary figure 4 for full membrane images). (c) NanoSight-derived counts for EVs extracted from control and heat shocked MCF7 cells. Error bars show standard error of the mean for three biological replicates. (d) Average EV size measured using TEM. Error bars show standard error of the mean for a total of 50 EVs counted. (e–g) MCF7 cells were heat shocked at 45°C (HS) or maintained at the control temperature of 37°C (Ctrl) for 1 h and maintained at 37°C for 24 h. The conditioned media (CM) was removed, EVs were isolated and placed onto naïve MCF7 cells, 24 h later the level of DNA damage (e), apoptosis (f) and viability (g) of the bystander cells was measured. For comet assays a total of at least 300 cells were counted across two biological replicates. Box and whisker plots show percentage of DNA in the comet tail, median, upper and lower quartiles, error bars are 1.5× interquartile range. For apoptosis assays at least 800 cells were counted across four biological replicates, error bars represent standard error of the mean. For MTTs absorbance at 595 nm in 30 wells containing treated bystander cells was measured. Box and whisker plots show absorbance at 595 nm, median, upper and lower quartiles, error bars are 1.5× interquartile range. *p < 0.05, **p < 0.01 and ***p < 0.001.
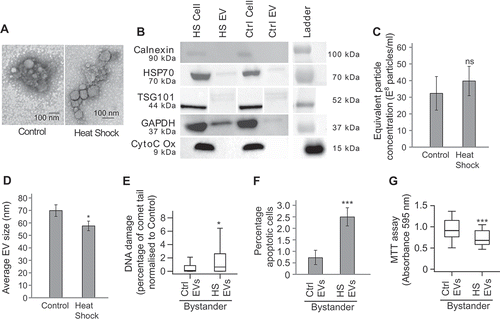
Heat shock induces an intercellular adaptive effect mediated by EVs
It has been previously shown that radiation-induced BE can also be associated with a protective effect in the recipient cells [Citation32]. The ability of heat shock EVs to protect naïve recipient cells from recurrent heat stress was assessed. MCF7 cells were treated with EVs collected from cells incubated at either 45°C or 37°C for 1 h and then grown for 24 h. These bystander cells were heat shocked at 45°C for 1 h and 24 h later the cells were harvested and levels of DNA damage and apoptosis were measured (–) respectively). Heat shocked cells that had previously received EVs from heat shocked cells had significantly lower levels of DNA damage and apoptosis than heat shocked cells that had been pre-treated with control-cell CM. Similar results were obtained from K562 cells treated the same way (Supplementary figure 3). These data suggest that the bystander cells have become adapted and are more robust in the face of a subsequent stressor.
Figure 4. Heat shock BE mediates an adaptive response in recipient cells. MCF7 cells were heat shocked after pre-treatment with heat shocked cell EVs (HS EV) or control-cell EVs (Ctrl EV); effects on DNA damage (a) and apoptosis (b) were measured using the comet assay and apoptosis assay, respectively. The data show a protective effect when cells are heat shocked after pre-treatment with heat shocked cell EVs. For comet assays a total of at least 250 cells were counted across two biological replicates. Box and whisker plots show percentage of DNA in the comet tail, median, upper and lower quartiles, error bars are 1.5× interquartile range. For apoptosis assays, at least 800 cells were counted across two biological replicates, error bars represent standard error of the mean. (c) MCF7 cells were incubated for 1 h at 45°C or 37°C whilst being treated with EV uptake inhibitors (amiloride or dynasore) or vehicle (control). Twenty-four hours later, viability was measured using the MTT assay. Readings at 45°C for each group were normalised to the average reading for that group at 37°C and then samples were normalised to the vehicle control. Error bars show standard error of the mean for twelve biological replicates. *p < 0.05, **p < 0.01 and ***p < 0.001.
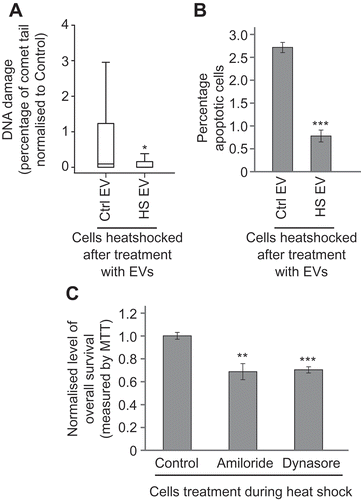
We reasoned that during heat shock treatment a cohort effect would take place, with heat shocked cells within the population releasing EVs that could potentially cause an adaptive response in other recipient cells within the cell flask. Inhibiting EV uptake should prevent this adaptive response and thus appear to sensitise the cohort to the effect of a stressor. To test this, we used two known EV uptake inhibitors, dynasore and amiloride. Both drugs increased the sensitivity of MCF7 cells to the effects of heat stress ()). Taken together, these data are consistent with a role for EVs in mediating an intercellular stress adaptation response.
Heat shock induces an intercellular invasive response mediated by EVs
There is a variety of evidence suggesting that EVs can play a role in metastatic processes [Citation33]. To test whether EVs secreted by heat shocked cells can induce invasive ability in bystander cells the Matrigel trans-well invasion assay was used (). EVs were collected from heat shocked or control cells and placed onto naïve MCF7 cells. Cells with the ability to invade through the Matrigel layer were stained and counted. There was a significant increase in invasive capacity when cells were treated with heat shock EVs (–). These results suggest that in addition to the effects on DNA damage and cell survival the EVs released from heat-shocked cells are able to induce invasion of cells in vitro.
Figure 5. Heat shock EVs can induce migration in bystander cells. (a) Schematic of the trans-well Matrigel invasion assay. (b) MCF7 cells were treated with either EVs from control cells (Ctrl EVs) or heat shocked cells (HS EVs) and the number of invasive cells in the trans-well Matrigel invasion assay was counted. Results are presented normalised to invasiveness of cells receiving control EVs. Error bars represent standard of the mean of nine biological replicates. Single star indicates p < 0.05. Representative images of the Matrigel assay are shown for cells treated with control (c) or heat shock (d) EVs. *p < 0.05.
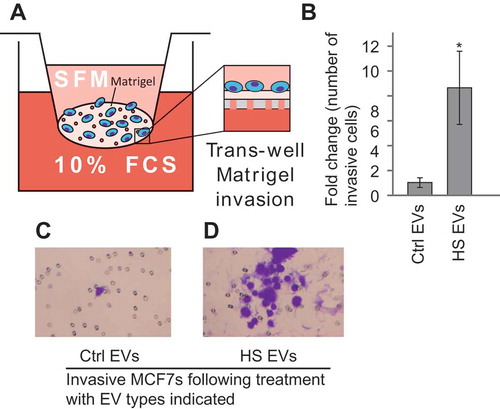
Discussion
EVs secreted into the media after heat shock are sufficient to induce damage in bystander cells
Previous work on thermal BE has shown that sub-lethal heat doses are sufficient to induce damage in bystander cells when grown in co-culture with directly stressed cells [Citation9,Citation10]. Studies with irradiated cells have also suggested that EVs released by cells are sufficient to induce bystander cell damage [Citation17–Citation19]. Here we examined whether EVs released from heat shocked cells were involved in thermal BE. EVs were extracted from heat shocked cells and then characterised using both western blot and electron microscopy. EV size, shape and protein cargo were all consistent with typical EV values [Citation34]. Interestingly EVs released from heat shocked cells are significantly smaller than those released by control treated cells when measured by TEM, a phenomenon we also observe in EVs released by cells treated with cisplatin and ionising radiation (manuscript in preparation). However, this was not seen when the EVs were measured via the NanoSight (data not shown). It could be that the observed differences in size seen with EM are an artefact of the fixation procedure as the NanoSight does not require fixed samples. There are some data suggesting that the NTA is not as good at determining the size of particles with a heterogeneous population compared with EM, which could also account for the differences [Citation35]. Nevertheless, the EM data results suggest a biophysical difference between EVs from normal and heat shocked cells.
The bystander cells showed increased levels of DNA damage and apoptosis and reduced cell viability. It is important to note that nuclear fragmentation is indicative of late apoptosis. Other assays, such as annexin V binding assays, could be used to see the cells that are in early apoptosis. These data support the hypothesis that EVs are important in the propagation of BE, at least in vitro. Further work is required to compare the ways in which EVs are secreted in response to different types of stress and their role in the induction of BE. Further, the method of EV extraction employed here primarily isolates the smaller EVs. Larger vesicles are pelleted in the 16,500g centrifugation step; in future it would be interesting to test whether these larger vesicles also contribute to the effect.
The mechanism by which EVs induce BE remains unclear; however, it could be due to changes in protein or RNA cargo within the vesicles that interact with the bystander cells. Future analysis of the content of both heat-treated and control EVs may implicate cargo molecules in the induction of BE. There is evidence that the cargo carried by EVs released from stressed cells is different than EVs released from unstressed populations. For example, differences in protein and RNA content have been demonstrated in vesicles from irradiated whole blood samples [Citation36]. Interestingly, one of the miRNAs found upregulated was miR-31-5p, a microRNA that we have previously shown to be involved in conferring cisplatin resistance [Citation37]. microRNAs are also known to be involved in stress responses and are often differentially regulated during stress [Citation38–Citation40], suggesting they may be responsible for mediating some or all aspects of EV-induced BE. Future work would seek to characterise the mechanism by which the heat shock EVs are able to induce these changes in bystander cells.
BE may exist as a way to increase the populations ability to resist stress
It has been shown that the BE is associated with a protective effect in recipient cells when subject to subsequent stress [Citation9,Citation10,Citation32]. Cells pre-treated with HS EVs showed lower levels of both DNA damage and apoptosis upon treatment with a second dose of thermal stress. These data suggest that pre-treatment with EVs from heat shocked cells are able to improve resistance to subsequent heat treatments. This may therefore represent an adaptation, whereby the cells in a population communicate through EVs in response to stress, to maximise the number of cells in the population that survive recurrent insults. To test this, we treated cells with inhibitors of EV uptake, amiloride and dynasore [Citation41,Citation42], and then heat shocked the cells. Cells treated with the inhibitors showed lower levels of survival following heat shock, suggesting that EVs released during stress do confer advantages for cell survival. Importantly these inhibitors do not specifically block EV uptake and these data could in fact be due to off-target effects of these inhibitors. However, when viewed in conjunction with the data above it does suggest some protective effect is conferred by the EVs. There is also some evidence that EVs released following oxidative stress or ionising radiation were able to confer resistance to repeat doses of stress in naïve cells [Citation43,Citation44].
EVs from heat stressed cells induce invasion in bystander cells
The release and uptake of EVs has been linked with invasion and metastasis of tumour cells [Citation33]. We investigated the impact of EVs released by heat shocked cells on the invasive behaviour of recipient cells. Using a trans-well assay, we showed that EVs from heat shocked cells induced a greater invasive capacity in bystander cells. This is consistent with previous work showing that exosomes released by glioblastomas following irradiation can induce higher levels of migration in recipient cells [Citation45]. These EVs were shown to increase the expression of numerous proteins related to migration including connective tissue growth factor (CTGF); it is possible that the same mechanism is causing the increase in migration following heat treatment. It also adds to the body of work demonstrating that EVs have pro-metastatic effects upon tumours [Citation46]. The ability of stress-induced EV secretion to cause increased metastatic behaviour in recipient cells appears not to be restricted to the effects of radiation, but may be a more general intercellular response to stress mediated by EVs. Further work is needed to establish the mechanisms by which this increased invasion is triggered as well as its in vivo significance.
Conclusions
Our data show that heat shocking cells induces the release of a subset of extracellular vesicles (EVs) that can induce a bystander effect (BE) in neighbouring untreated cells. The range of responses in bystander cells appears to encompass potentially positive and negative effects (), including increased DNA damage, apoptosis, resistance to recurrent thermal stress and invasiveness of recipient cells in vitro. These important results demonstrate that the EV-mediated BE observed following radiation is not just confined to one stress type. It also offers a new model for studying the BE and EV-mediated communication during stress response. Indeed, we speculate that this may represent a physiological response to stress, which is acting at the tissue level and is mediated by EVs. There are potential implications of this in the use of thermotherapy, for example, in its use to treat cancer. Hyperthermia is a form of cancer therapy currently undergoing clinical trials in which patients are subjected to high heat treatments usually in conjunction with chemo- or radiotherapy [Citation47]. As such it is important to understand what off target effects may be experienced, particularly those that induce an adaptive and invasive response in non-targeted tumour cells. Future research should focus on establishing the impact of EVs in the stress response in an in vivo context, and the molecular mechanisms by which this EV-mediated intercellular stress response operates.
Figure 6. EVs released during stress show both positive and negative effects on the surrounding population. When stressed cells release EVs they have a variety of effects on the nearby cells. Bystander cells show higher levels of DNA damage and apoptosis following treatment with heat shock derived vesicles, whilst the level of cell viability was reduced. These are all negative effects of the bystander effect; however, positive effects have also been demonstrated. Cells treated with HS EVs show greater invasiveness and show greater protection against heat treatment. These results both suggest that the bystander effect is not simply spreading damage but may in fact aid the survival of the bystander cells.
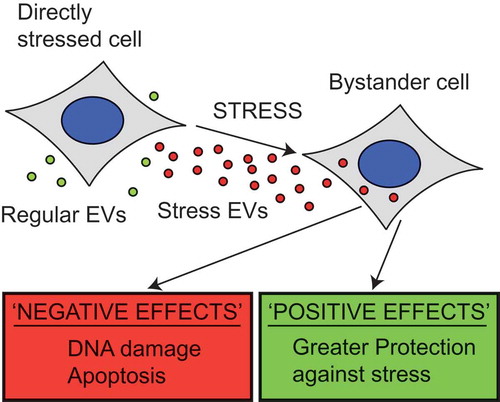
Disclaimer
The work below and any views expressed within are those of the authors alone
ZJEV_A_1340746_Supplementary_data.zip
Download Zip (3.8 MB)Acknowledgements
We acknowledge the Cancer and Polio Research Fund and Oxford Brookes University for funding and Travis Brooks, Adam McDermott-Rouse, Mel Webb and Philippa Murphy for technical help.
Disclosure statement
No potential conflict of interest was reported by the authors.
Additional information
Funding
References
- Hall EJ. The bystander effect. Health Phys. 2003;85(1):1–10.
- Chai Y, Hei TK. Radiation induced bystander effect in vivo. Acta Med Nagasaki. 2008;141(4):520–529.
- Kadhim M, Salomaa S, Wright E, et al. Non-targeted effects of ionising radiation-Implications for low dose risk. Mutation Res Rev Mutation Res. 2013;752(2):84–98.
- Hickman AW, Jaramillo RJ, Lechner JF, et al. Alpha-particle-induced p53 protein expression in a rat lung epithelial cell strain. Cancer Res. 1994;54(22):5797–5800.
- Nagasawa H, Little JB. Induction of sister chromatid exchanges by extremely low doses of α -particles. Cancer Res. 1992;2115(4):6394–6396.
- Kadhim MA, Macdonald DA, Goodhead DT, et al. Transmission of chromosomal instability after plutonium α-particle irradiation. Nature. 1992;355(6362):738–740.
- Asur RS, Thomas RA, Tucker JD. Chemical induction of the bystander effect in normal human lymphoblastoid cells. Mutat Res. 2009 May 31;676(1–2):11–16.
- Dabrowska A, Goś M, Janik P. “Bystander effect” induced by photodynamically or heat-injured ovarian carcinoma cells (OVP10) in vitro. Med Sci Monit. 2005;11(9):BR316–R324.
- Purschke M, Laubach H-J, Anderson RR, et al. Thermal injury causes DNA damage and lethality in unheated surrounding cells: active thermal bystander effect. J Invest Dermatol. Nature Publishing Group; 2010 Jan;130(1):86–92.
- Purschke M, Anderson RR, Zurakowski D, et al. Cell-cycle-dependent Active Thermal Bystander Effect (ATBE). Lasers Surg Med. 2011;43(3):230–235.
- Shao C, Furusawa Y, Aoki M, et al. Role of gap junctional intercellular communication in radiation-induced bystander effects in human fibroblasts. Radiat Res. 2003;160(3):318–323.
- Desai S, Kobayashi A, Konishi T, et al. Damaging and protective bystander cross-talk between human lung cancer and normal cells after proton microbeam irradiation. Mutat Res. 2014;763–764(January):39–44.
- Lyng FM, Seymour CB, Mothersill C. Production of a signal by irradiated cells which leads to a response in unirradiated cells characteristic of initiation of apoptosis. Br J Cancer. 2000;83(9):1223–1230.
- Mothersill C, Seymour C. Medium from irradiated human epithelial cells but not human fibroblasts reduces the clonogenic survival of unirradiated cells. Int J Radiat Biol. 1997;71(4):421–427.
- Mancuso M, Pasquali E, Leonardi S, et al. Oncogenic bystander radiation effects in Patched heterozygous mouse cerebellum. Proc Natl Acad Sci U S A. 2008;105(34):12445–12450.
- Ilnytskyy Y. Radiation‐induced bystander effects in vivo are epigenetically regulated in a tissue‐specific manner. Environ Mol Mutagen. 113(December2008). 2009;50:105–113.
- Ahj A-M, Bright S, Chapman K, et al. The non-targeted effects of radiation are perpetuated by exosomes. Mutat Res Mol Mech Mutagen. Elsevier B.V.; 2015;772:38–45.
- Ahj A-M, Irons SL, Pink RC, et al. Possible role of exosomes containing RNA in mediating nontargeted effect of ionizing radiation. Radiat Res. 2012;177(5):539–545.
- Jella KK, Rani S, O’Driscoll L, et al. Exosomes are involved in mediating radiation induced bystander signaling in human keratinocyte cells. Radiat Res. 2014;181(2):138–145.
- Xu S, Wang J, Ding N, et al. Exosome-mediated microRNA transfer plays a role in radiation-induced bystander effect. RNA Biol. 2015;6286(12):1355–1363.
- Théry C, Zitvogel L, Exosomes: AS. composition, biogenesis and function. Nat Rev Immunol. 2002 Aug;2(8):569–579.
- Bobrie A, Colombo M, Raposo G, et al. Exosome secretion: molecular mechanisms and roles in immune responses. Traffic. 2011 Dec;12(12):1659–1668.
- Urbanelli L, Magini A, Buratta S, et al. Signaling pathways in exosomes biogenesis, secretion and fate. Genes (Basel). 2013;4(2):152–170.
- Kowal J, Tkach M, Théry C. Biogenesis and secretion of exosomes. Curr Opin Cell Biol. 2014;29(1):116–125.
- Akers JC, Gonda D, Kim R, et al. Biogenesis of extracellular vesicles (EV): exosomes, microvesicles, retrovirus-like vesicles, and apoptotic bodies. J Neurooncol. 2013;113(1):1–11.
- Record M, Subra C, Silvente-Poirot S, et al. Exosomes as intercellular signalosomes and pharmacological effectors. Biochem Pharmacol. Elsevier Inc.; 2011 May 15;81(10):1171–1182.
- Yáñez-Mó M, Siljander PR-M, Andreu Z, et al. Biological properties of extracellular vesicles and their physiological functions. J Extracell Vesicles. 2015;4:1–60.
- Huang X, Yuan T, Tschannen M, et al. Characterization of human plasma-derived exosomal RNAs by deep sequencing. BMC Genomics. 2013;14:319.
- Hartmann A, Agurell E, Beevers C, et al. Recommendations for conducting the in vivo alkaline Comet assay. Mutagenesis. 2003;18(1):45–51.
- Końca K, Lankoff A, Banasik A, et al. A cross-platform public domain PC image-analysis program for the comet assay. Mutat Res - Genet Toxicol Environ Mutagen. 2003;534(1–2):15–20.
- Einaudi L, Courbiere B, Tassistro V, et al. In vivo exposure to benzo(a)pyrene induces significant DNA damage in mouse oocytes and cumulus cells. Hum Reprod. Oxford University Press; 2014 Mar 1;29(3):548–554.
- Shankar B, Pandey R, Sainis K. Radiation-induced bystander effects and adaptive response in murine lymphocytes. Int J Radiat Biol. 2006;82(8):537–548.
- Hoshino A, Costa-Silva B, Shen T-L, et al. Tumour exosome integrins determine organotropic metastasis. Nature. Nature Publishing Group; 2015;5277578:329–335.
- Witwer KW, Buzás EI, Bemis LT, et al. Standardization of sample collection, isolation and analysis methods in extracellular vesicle research. J Extracell Vesicles. 2013;2: 20360.
- Anderson W, Kozak D, Coleman VA, et al. A comparative study of submicron particle sizing platforms: accuracy, precision and resolution analysis of polydisperse particle size distributions. J Colloid Interface Sci. 2013;405:322–330.
- Yentrapalli R, Merl-Pham J, Azimzadeh O, et al. Quantitative changes in the protein and miRNA cargo of plasma exosome-like vesicles after exposure to ionizing radiation. Int J Radiat Biol. Taylor & Francis; 2017;00:1–37.
- Samuel P, Pink RC, Caley DP, et al. Over-expression of miR-31 or loss of KCNMA1 leads to increased cisplatin resistance in ovarian cancer cells. Tumor Biol. 2016;37(2):2565–2573.
- Leung AKL, Sharp PA. MicroRNA functions in stress responses. Molecular Cell. 2010;40:205–215.
- Mendell JT, Olson EN. MicroRNAs in stress signaling and human disease. Cell. Elsevier Inc.; 2012 Mar 16;148(6):1172–1187.
- Jacobs LA, Bewicke-Copley F, Poolman MG, et al. Meta-analysis using a novel database, miRStress, reveals miRNAs that are frequently associated with the radiation and hypoxia stress-responses. Pan X, editor. Plos One. 2013 Nov 14;8(11):e80844.
- Feng D, Zhao WL, Ye YY, et al. Cellular internalization of exosomes occurs through phagocytosis. Traffic. 2010 May;11(5):675–687.
- Newton AJ, Kirchhausen T, Murthy VN. Inhibition of dynamin completely blocks compensatory synaptic vesicle endocytosis. Proc Natl Acad Sci U S A. National Academy of Sciences; 2006 Nov 21;103(47):17955–17960.
- Eldh M, Ekström K, Valadi H, et al. Exosomes communicate protective messages during oxidative stress; possible role of exosomal shuttle RNA. Plos One. 2010 Jan;5(12):e15353.
- Mutschelknaus L, Peters C, Winkler K, et al. Exosomes derived from squamous head and neck cancer promote cell survival after ionizing radiation. Plos One. 2016.
- Arscott WT, Tandle AT, Zhao S, et al. Ionizing radiation and glioblastoma exosomes: implications in tumor biology and cell migration. Transl Oncol. 2013;6(6):638–648.
- Fujita Y, Yoshioka Y, Ochiya T. Extracellular vesicle transfer of cancer pathogenic components. Cancer Sci. 2016;107(4):385–390.
- Mallory M, Gogineni E, Jones GC, et al. Therapeutic hyperthermia: the old, the new, and the upcoming. Crit Rev Oncol Hematol. 2015;97:56–64.