ABSTRACT
Type 2 diabetes mellitus (T2DM), a chronic disease, is widely prevalent all over the world. In recent years, the roles of some extracellular vesicles (EVs) in T2DM have attracted much attention. EVs are bilayer membrane vesicles secreted from most cells and can participate in regulating various physiological and pathological processes in vivo by being transported between cells. Recently, it was discovered that some abnormal EVs can contribute to the occurrence of T2DM by inducing insulin resistance and can also participate in the complications of T2DM. In addition, some stem/progenitor cells-derived EVs have a potential application in the therapy of T2DM. This review introduces basic concepts of EVs and summarizes the roles of EVs in the pathogenesis, complications, and therapy of T2DM.
Introduction
Diabetes mellitus is a group of metabolic disorders characterized by high blood sugar levels over a prolonged period [Citation1]. It occurs either when the pancreas does not produce sufficient insulin or when the body cannot effectively use the insulin produced. Generally, the symptoms of patients with diabetes mellitus include polyuria, polydipsia, constant hunger, and weight loss. Diabetes mellitus, if not well controlled, will cause serious complications including heart attack, kidney failure, unhealed wounds, vision loss, and nerve damage [Citation2–Citation6]. In addition, diabetes is also a risk factor for the prevalence of cancer [Citation7]. Currently, the number of patients with diabetes mellitus has reached more than 422 million worldwide and has been increasing rapidly [Citation8]. This disease has become an important public health problem. Notably, this figure was predicted to increase to 693 million by 2045 [Citation9].
There are three major types of diabetes mellitus: type 1 diabetes mellitus, type 2 diabetes mellitus, and gestational diabetes mellitus. Among these types of diabetes mellitus, T2DM has the greatest impact and accounts for approximately 90–95% of all diabetes types [Citation10]. T2DM results from insulin resistance, defects in insulin secretion, or both [Citation11]. However, the pathogenesis of T2DM is not yet fully elucidated and might involve multiple factors including family history, obesity, poor diet, as well as lack of exercise. Although the current therapeutic methods for this disease mainly include hypoglycaemic drugs, insulin, and other symptomatic treatment, these methods are still not effective in improving the patient’s condition. Therefore, more treatment alternatives are urgently needed for the clinical treatment of patients.
Notably, some studies have reported that extracellular vesicles (EVs) could play important roles in the pathogenesis, complications, and therapy of T2DM. The earliest study found that platelet-derived EVs levels were significantly increased in diabetes mellitus, and showed that platelet-derived EVs might participate in the development or progression of atherosclerosis in diabetes mellitus [Citation12]. Later, reports indicated different patterns of expression and procoagulant activity in the plasma EVs from patients with type 1 and type 2 diabetes with vasculopathies [Citation13]. Recently, a study showed that angiogenesis of diabetic mice was promoted by miR-126 in the EVs secreted by CD34+ peripheral blood mononuclear cells from healthy human volunteers [Citation14]. Despite the multiple studies indicating the significance of EVs in T2DM, a systematic examination of the relationship between EVs and T2DM has not been reported. In this review, we summarize the latest advances concerning the roles of EVs in T2DM.
Description of EVs
Classification and origin
The term “EVs” describes a class of small vesicles (30–2000 nm) that contain a variety of biomolecules, such as lipids, proteins, and nucleic acids. These vesicles are released by most types of cells including stem cells [Citation15], progenitor cells [Citation16], endothelial cells [Citation17], adipocytes [Citation18], and cancer cells [Citation19]. Based on their biogenesis, EVs are classified into three groups: exosomes, microvesicles, and apoptotic bodies (see ) [Citation20].
Table 1. Types of EVs.
Exosomes are the smallest EVs, with size ranging between 40 and 200 nm. They were initially isolated from sheep reticulocytes in 1983 [Citation21]. Generally, exosomes are generated within the cells through the endosomal pathway (see ). Firstly, the plasma membrane of the donor cell is internalized to produce endosomes. Then, proteins and RNAs (including lncRNA, mRNA, miRNA, and circRNA) are selectively packed into multivesicular bodies (MVBs) through endosomal sorting complex required for transport (ESCRT)-dependent pathway (, red-dotted line) or ESCRT-independent pathway (, black-dotted line). Subsequently, some of the MVBs are degraded by fusion with lysosomes, whereas others undergo Rab27-mediated fusion with the plasma membrane and are released into the extracellular space to become exosomes [Citation22–Citation24]. Microvesicles, ranging in size from 200 nm to 2,000 nm in diameter, are generated by the outward budding and fission of the plasma membrane of the donor cells. They were initially described as subcellular material originating from platelets in serum and plasma [Citation25]. The apoptotic bodies, with diameters ranging from 500 to 2000 nm, are relatively large vesicles derived from the apoptotic cells. These vesicles contain a few nuclei and proteins from apoptotic cells [Citation26].
Figure 1. Biogenesis of EVs.
Exosomes are formed via the endosomal pathway and are released upon fusion of MVBs with the plasma membrane. Microvesicles are generated by the outward budding and fission of the plasma membrane of the donor cells. The apoptotic bodies are large vesicle derived from the apoptotic cells.
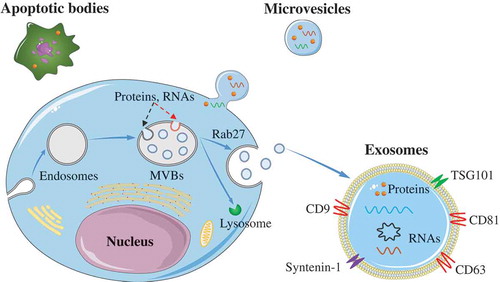
Isolation and identification
EVs exist in various body fluids, such as blood, urine, saliva, and spinal fluid [Citation27]. The commonly used methods for EVs isolation include ultracentrifugation, density gradients, precipitation, immuno-affinity capture, size exclusion chromatography, and ultrafiltration [Citation28–Citation34]. (a) Currently, the “gold standard” for purification of EVs is ultracentrifugation, which typically consists of low-speed centrifugation to remove cells and large vesicles and high-speed ultracentrifugation to pellet EVs. (b) Density gradients, a more stringent form of ultracentrifugation, further fractionate the vesicles based on density, with different vesicles undergoing sedimentation across the gradient at different rates. (c) Precipitation using chemicals, such as poly (ethylene glycol) (PEG), reduce the solubility of EVs to cause precipitation. The precipitated EVs could be easily and reproducibly isolated with low centrifugal forces. (d) Immuno-capture isolates vesicles using beads coated with antibodies to which the protein of interest can bind. (e) Size exclusion chromatography enables size-based separation of EVs on the column, with EVs eluting before soluble components such as proteins. (f) Ultrafiltration allows effective separation of EVs from the sample. To pass the impurity through the filter, a pressure is applied, or the filter is placed in an ultracentrifuge. However, the current isolation methods remain challenging and all of these methods have advantages as well as disadvantages (see ). They can’t effectively remove soluble impurities from the sample, such as lipoproteins in the blood which co-precipitate with EVs [Citation35]. Therefore, novel effective methods are highly desirable to isolate subpopulations of vesicles based on their size, density, or composition. Recently, microfluidic isolation was described as a promising method for selective concentration of EVs [Citation36–Citation38]. This technology may be ideal since it enables fast and precise isolation of EVs from small volumes of liquid samples.
Table 2. Isolation methods for EVs.
Western blot analysis, nanoparticle tracking, and transmission electron microscopy are the most commonly used approaches for analysing composition and morphology of EVs populations. Western blot analysis is a method to identify marker proteins of EVs, nanoparticle tracking analysis is an optical particle tracking method developed to determine concentration and size distribution of particles, and transmission electron microscopy is widely used to measure the physical features of EVs, such as vesicle size and distribution, concentration, and morphologies. Generally, the three approaches are used in combination with the identification and characterization of EVs. Recently, the application of flow cytometry in EVs population analysis has attracted great attention. A high-sensitivity flow cytometer allowed the quantitative multiparameter analysis of single EVs down to 40 nm, with an analysis rate up to 10,000 particles per minute. The apparatus was able to provide a sensitive and rapid platform for sizing and surface protein profiling of individual EVs [Citation39]. Furthermore, single-EV flow cytometry could realize single EV counting and phenotyping by target-initiated engineering of DNA nanostructures on each EV. By identifying markers on single EVs, single-EVs flow cytometry could efficiently recognize cancer cell-derived EVs among heterogeneous EVs populations [Citation40]. Therefore, flow cytometry may be an effective tool for the analysis of EVs.
In the field of EVs, most studies have focused on exosomes. Due to the overlapping range of size and density (see ), the purification of exosomes, microvesicles, and apoptotic bodies is technically difficult. A purified exosomes fraction was not used in most studies, and the presence of large/medium EVs and other contaminants had been confirmed [Citation41]. In addition, there is still a lack of specific markers that effectively distinguish between exosomes and microvesicles. In the past, tetraspanins (CD9, CD63, and CD81), HSP70, MHC, and TSG101 were considered as specific markers for exosomes. However, recent studies showed that these proteins might not be as specific as previously considered [Citation42]. A recent study used a rigorous approach (differential ultracentrifugation + iodixanol gradient fractionation) to isolate EVs and defined some novel markers for EVs subtypes [Citation43]. Several proteins often used as “exosomes markers,” such as CD9, CD63, MHC, Flotillin, and HSP70 were found to be present not only in the small EVs (sEVs, often called exosomes) but also in the larger EVs (i.e., microvesicles). The results further indicated that syntenin-1 and TSG101 might be specific for the sEVs enriched in CD63, CD9, and CD81 tetraspanins, representing bona fide “exosomes”. Recently, Jeppesen et al. [Citation44] reassessed exosomes composition via a combination of methods using high-resolution iodixanol density gradient fractionation and direct immunoaffinity capture. They showed that non-exosomes sEVs could be characterized by annexins A1 and A2, and identified annexin A1 as a specific marker for microvesicles that are shed directly from the plasma membrane. At present, there is no consensus yet on specific markers of EV subtypes. So, the term “exosomes” should be used with caution. Here, we have used the general term “EVs” while describing such studies throughout this review.
Pathophysiological functions
For a long time, EVs were recognized as cell debris with no significant functions and were thus under-appreciated. However, after the discovery of RNAs within EVs in 2007, EVs have been increasingly considered an important way of intercellular communication [Citation45]. Subsequently, they had garnered much attention in the past decade as novel and important delivery vehicles for signal molecule in vivo. These vesicles can carry a range of nucleic acids and proteins and can protect these molecules from degradation in the extracellular space [Citation46,Citation47]. After transport into target cells, they have an admittedly significant impact on the phenotype of these recipient cells.
Recently, some studies revealed the functions of EVs in physiological processes. Truman-Rosentsvit et al. [Citation48] found that ferritin could be secreted via EVs. The ability of these EVs to transfer iron-carrier molecules between cells might play an important role in the maintenance of iron homeostasis under physiological conditions and intercellular communication. Notably, EVs have also been implicated in presenting antigens between immune cells, thereby acting as an important mode of communication for initiating and maintaining an adaptive immune response [Citation49]. Interestingly, endothelial cells and adipocytes can exchange protein and lipid signals via EVs, and participate in conveying information about nutrient state changes from the blood and adipose tissues [Citation50]. Furthermore, Nocera et al. [Citation51] discovered novel innate immunosurveillance and defence mechanism of the upper airway mediated by EVs. In vivo, human nasal mucosa cells could eliminate airway pathogens and provide passive epithelial immune-protection both via the release of EVs.
Additionally, some EVs play a key role in a different pathological condition, including neurological diseases, cancer, as well as metabolic disease. Sardar et al. [Citation52] showed that Alzheimer brain EVs were enriched with toxic amyloid-β and played an important role in promoting disease progression in Alzheimer brain via spreading this toxic protein between nerve cells. Pavlyukov et al. [Citation53] showed that apoptotic glioblastoma cells could promote proliferation and therapy resistance of surviving tumour cells by secreting EVs enriched with splicing factors. Interestingly, EVs-mediated crosstalk between stromal cells and breast cancer cells was found to affect the progression of breast cancer. Unshielded RNA RN7SL1 carried by EVs from active stromal cells could enhance tumour growth, metastasis, and therapy resistance of recipient breast cancer cells [Citation54]. Recently, Chen et al. [Citation55] reported that metastatic melanoma cells suppressed the immune system and evaded immune surveillance by releasing EVs that carry PD-L1 on their surface, thus revealing a novel mechanism by which tumour cells could systemically contribute to immunosuppression and anti-PD-1 response. Freeman et al. [Citation56] indicate that levels of EVs from the blood of diabetic patients were significantly increased and might be important signalling mediators in diabetes. These EVs were preferentially internalized by monocytes and altered monocyte function including increasing expression of genes associated with immune function and inflammation, as well as decreasing the apoptosis and oxidative stress-related genes.
Abnormal EVs induce insulin resistance
Insulin resistance in T2DM arises from the inability of insulin to regulate glucose metabolism in peripheral tissues, including liver, adipose, and skeletal muscle. Recent studies reported that some abnormal EVs from different cells might directly or indirectly induce insulin resistance through activating inflammation, down-regulating glucose transporter type 4 (GLUT4), and affecting the insulin receptor (IR). Below, we summarize the potential mechanisms of insulin resistance induced by EVs.
Activating inflammation via M1 macrophages
Inflammation plays an important part in obesity-associated insulin resistance. Generally, pro-inflammatory adipose tissue macrophages (also called M1 macrophages) can induce insulin resistance in target cells through releasing pro-inflammatory cytokines, including tumour necrosis factor α (TNF-α), interleukin 6 (IL-6), interleukin 1β (IL-1β), and monocyte chemoattractant protein 1 (MCP-1) [Citation57,Citation58]. However, the mechanisms associated with the activation of M1 macrophages have not been fully elucidated. Recent data suggested that EVs from adipocyte could promote the differentiation of macrophages into M1 macrophages. These EVs might play a key role in signal crosstalk between adipocytes and macrophages, with the potential to aggravate insulin resistance in myocytes, adipocytes, and hepatocytes.
Previously, Deng et al. [Citation59] found that the retinal binding protein 4 (RBP4)-containing EVs derived from adipose tissue of obese mice could stimulate the differentiation of monocytes into M1 macrophages through the TLR4/TRIF pathway. These M1 macrophages could increase the secretion of both TNF-α and IL-6, which might induce insulin resistance in myocytes. Subsequently, it was confirmed that the EVs differentiating monocytes into M1 macrophages came from adipocytes [Citation60]. These adipocyte-derived EVs was found to contain adiponectin, an adipocyte-specific protein, and show clear enrichment of some pro-inflammatory cytokines such as TNF-α and RBP-4. Recently, Zhang et al. [Citation61] observed that adipocyte-derived EVs from obese mice could induce M1 macrophage phenotype and cause insulin resistance in the adipocyte. The EVs secreted by adipocytes were delivered to bone marrow macrophages (BMMs), and the miR-155 in these EVs could effectively induce BMMs polarization toward M1 phenotypes by targeting SOCS1 and regulating the JAK/STAT pathway. Similarly, Song et al. [Citation62] revealed that the sonic hedgehog protein carried by mice adipocyte-EVs could mediate BMMs to M1 macrophage polarization through the Ptch/PI3K pathway, possibly contributing to insulin resistance in adipocytes. Furthermore, high concentration of pro-inflammatory cytokines (including IL-6, MCP-1, RBP-4, and adiponectin) contained in the EVs of adipose tissue from obese patients was found to induce insulin resistance in hepatocytes and myocytes [Citation63].
Together, these findings indicate a potential mechanism whereby abnormal EVs derived from adipocyte may induce insulin resistance via the transmission of inflammatory signals inside the target cells (see ).
Figure 2. Activating inflammation via M1 macrophages.
Adipose tissue derived-EVs or adipocyte derived-EVs could induce insulin resistance in insulin target cells (including adipocyte, hepatocytes, and myocytes) through activating inflammation. The cargos (light blue box) in these EVs were able to promote monocytes or BMMs polarization toward M1 phenotypes. Then, M1 macrophages may induce insulin resistance in target cells through releasing pro-inflammatory cytokines, such as TNF-α, IL-6, IL-1β, and MCP-1. In addition, adipokines carried by the adipose tissue derived-EVs were able to induce insulin resistance.
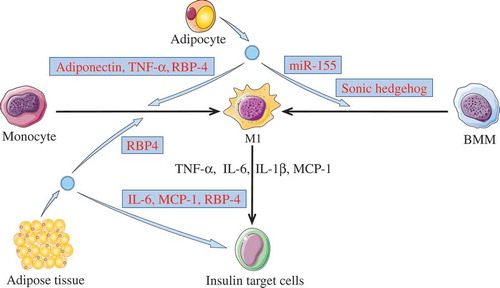
Down-regulation of GLUT4 in adipocytes and skeletal muscle cells
Glucose transporter type 4 (GLUT4) is an important glucose transport protein in the insulin signalling pathway. Notably, GLUT4 expression might also be affected by abnormal EVs, and down-regulated GLUT4 could decrease glucose uptake in cells and further impair insulin signalling. Zhang et al. [Citation64] discovered that human M1 macrophages-released EVs could be effectively taken up by adipocytes, consequently decreasing the translocation of GLUT4 from the cytoplasm to the cell surface in adipocyte through NF-κB activation. However, the related molecules in these EVs that affected the expression of GLUT4 on cell membranes were not revealed in this study. Recently, it was shown that M1 macrophage-derived EVs of obese mice were also taken up by adipocytes and promoted insulin resistance [Citation65]. The over-expressed miR-155 in these EVs could decrease the expression of GLUT4 via downregulation of peroxisome proliferator-activated receptor γ (PPAR-γ). Similarly, Yu et al. [Citation66] recently found that the miR-27a from the adipocyte-derived EVs of obese mice could decrease the expression of PPAR-γ in skeletal muscle, further leading to a decrease in the expression of GLUT4. In addition, Wang et al. [Citation67] indicated that EVs from pancreatic cancer cells (PCs) could induce insulin resistance in mice skeletal muscle cells through the PI3K/Akt/FoxO1 pathway. They also revealed that miR-883b-5p and miR-666-3p in these EVs might play essential roles in down-regulation of GLUT4 expression. Collectively, these studies show that some abnormal EVs might be involved in the induction of insulin resistance by affecting GLUT4 expression (see ).
Figure 3. Down-regulating GLUT4 via EVs.
Some EVs can induce insulin resistance by down-regulating GLUT4. miR-155 in M1 macrophage-derived EVs, or miR-27a from the adipocyte-derived EVs can inhibit the expression of GLUT4 via decreasing PPAR-γ. Both miR-883 and miR-450 in EVs from PCs may also affect the expression of GLUT4. In addition, M1 macrophage-released EVs could decrease the GLUT4 translocation from the cytoplasm to the cell surface.
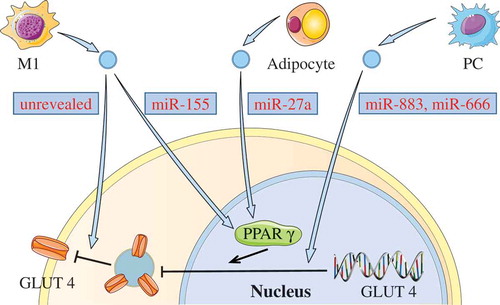
Affecting insulin receptor in hepatocytes
Generally, insulin binds to insulin receptor (IR) on the plasma membrane and activates the intracellular insulin signalling pathway via insulin receptor substrates (IRS). If the IR is damaged, it may lead to abnormal insulin signalling. Recently, it was found that under hyperglycaemic conditions, the insulin receptor β-subunit in hepatocytes was sequentially cleaved by both the calpain 2 and γ-secretase of hepatocyte-EVs (see ) [Citation68]. These cleaved IRs lead to impaired insulin signalling and caused insulin resistance in the long term. This suggests that abnormal EVs could induce insulin resistance via affecting the IRs, although this needs further thorough investigation.
Abnormal EVs participate in diabetic complications
Diabetic nephropathy (DN), diabetic cardiomyopathy (DCM), and diabetic retinopathy (DR) are the most common and most serious complications of T2DM. Emerging data show that abnormal EVs might be an important factor contributing to the pathogenesis of diabetic complication. Here, we focus on the potential role of EVs in these three major complications.
Diabetic nephropathy
Diabetic nephropathy (DN), the most common cause of chronic kidney disease, is a microvascular complication characterized by glomerular fibrosis, and the transforming growth factor-β1 (TGF-β1) is the predominant agent mediating these fibrotic changes [Citation69]. Some studies implicated that abnormal EVs might be involved in the development of DN via the TGF-β1 pathway. Recently, miR-320c in urinary EVs from DN patients was found to be strongly up-regulated [Citation70]. The over-expressed miR-320c could affect the TGF-β1 pathway by down-regulating thrombospondin 1 (TSP1), thereby accelerating the development of DN. Interestingly, it was reported that the over-expressed miR-192 from urinary EVs in patients with early DN was positively correlated with the expression of TGF-β1, which may be related to the development of DN [Citation71]. Recently, Wu et al. [Citation72] indicated that the glomerular mesangial cells (GMCs) could be abnormally activated by TGF-β1 mRNA of EVs derived from high glucose-treated glomerular endothelial cells (GECs). The TGF-β1 mRNA in these EVs promoted α-smooth muscle actin expression, proliferation and extracellular matrix protein overproduction in GMCs through the TGF-β1/Smad3 pathway, which might promote renal fibrosis. Additionally, Wu et al. [Citation73] also found that the TGF-β1 mRNA in these EVs could cause the epithelial-mesenchymal transition and the dysfunction of the glomerular barrier in glomerular podocytes through the Wnt/β-catenin pathway, further leading to glomerular fibrosis. In summary, these results suggest that abnormal EVs have a potential role in DN.
Diabetic cardiomyopathy
Diabetic cardiomyopathy (DCM) is a ventricular dysfunction initiated by disorders of glucose metabolism. Recently, some EVs were found to be a vital precursor for the damage to the myocardium in DCM. Wang et al. [Citation74] showed that EVs derived from cardiomyocytes of diabetic rats contained higher levels of miR-320 and lower levels of miR-126 compared with EVs from healthy controls. The over-expressed miR-320 in these EVs could be transferred to cardiac endothelial cells of rats, and inhibit the proliferation, migration, and tube formation of cardiac endothelial cells by down-regulating the expression of IGF-1, Hsp20, and Ets-2. Additionally, Davidson et al. [Citation75] indicated that cardio-protection mediated by the EVs containing HSP70 was impaired in the background of T2DM. The HSP70 in platelet EVs from diabetic rats no longer activated the ERK1/2 and HSP27 cardioprotective pathway in injured cardiomyocytes, which would inhibit the proliferation and angiogenesis of cardiomyocytes. Recently, Hu et al. [Citation76] indicated that abnormal crosstalk between cardiac endothelial cells and cardiomyocyte mediated by abnormal EVs could deteriorate DCM. Mst1 protein in EVs from cardiac endothelial cells of diabetic mice was found to transfer to cardiomyocytes, and induce cardiac dysfunction by inhibiting autophagy, promoting apoptosis, and suppressing the glucose metabolism.
Diabetic retinopathy
Diabetic retinopathy (DR) is a common complication of advanced T2DM, characterized by loss of retinal pericytes and abnormal angiogenesis. It was reported that some abnormal EVs played a key in the development of vascular damage and progression of DR. Mazzeo et al. [Citation77] found that plasma EVs from DR patients were able to induce features of retinopathy models of retinal microvasculature. The expression of miR-150-5p, miR-21-3p, and miR-30b-5p were significantly increased in these EVs compared with healthy controls. These increased miRNAs might be associated with retinal pericyte detachment and abnormal angiogenesis. Interestingly, IgG were found in plasma EVs of diabetic mice, and these IgG could lead to microvascular damage in DR via activation of the classical complement pathway [Citation78]. Additionally, Kamalden et al. [Citation79] showed that miR-15a was significantly increased in the plasma EVs of diabetic patients, correlating with retinal injury. miR-15a in these plasma EVs could transfer to retinal Müller cells, where they induced oxidative stress by targeting Akt3, leading to retinal cell injury. Recently, Liu et al. [Citation80] revealed the crosstalk between vascular pericytes and endothelial cells regulated by EVs in DR. Diabetes-related stress up-regulated circRNA cPWWP2A expression in pericytes; the cPWWP2A could then be transferred from pericytes to endothelial cells through EVs. These circRNAs could increase expression of angiopoietin 1, occludin, and sirtuin 1 via inhibition of miR-579 activity, thus inducing retinal vascular dysfunction.
Therapy for T2DM and its complications via EVs
EVs not only participate in the pathogenesis of T2DM but can also apply T2DM therapy. Generally, EVs enhance or inhibit the functions of target cells via the delivery of specific molecules, improving the disease effectively. Recently, some studies successively reported animal trials with stem/progenitor cell-derived EVs to treat T2DM and its complications. Notably, these EVs have low immunogenicity and high targeting ability in vivo. These recent updates on the application of EVs in the therapy of T2DM and associated complications are summarized in .
Table 3. the application of EVs in the therapy of T2DM and its complications.
Type 2 diabetes mellitus
In the past, the treatment of diabetes was heavily dependent on hypoglycaemic agents and insulin. Interestingly, some EVs may have potential applications in alleviating T2DM. Zhao et al. [Citation81] used EVs from adipose stem cells to treat obese mice and found that the STAT3 protein carried by these EVs could induce macrophages to form anti-inflammatory M2 phenotypes through the transactivation of arginase, thereby improving both metabolic balance and insulin resistance in mice. Subsequently, Tsukita et al. [Citation82] indicated that hyperglycaemia in diabetic mice was ameliorated after bone marrow transplantation. They showed that the miR-106b-5p and miR-222-3p in EVs secreted by the bone marrow cells of mice were able to promote the proliferation of pancreatic β-cells by down-regulating the Cip/Kip pathway. Another study reported that EVs from human mesenchymal stem cell (MSCs) could alleviate T2DM in rats by reversing peripheral insulin resistance and relieving β-cell destruction, providing an alternative approach for T2DM treatment [Citation83]. Thus, EVs might be helpful in the treatment of T2DM, although further investigation is required for its clinical application.
Diabetic wounds
The wounds of diabetic patients either heal slowly or fail to heal, and open wounds increase the risk of infection. Accelerated diabetic-wound healing is therefore an urgent need. Geiger et al. [Citation84] reported that human fibroblast-derived EVs might play a positive role in accelerating the wound healing of diabetic mice. These EVs carried HSP90α, pro-angiogenic miRNAs (miR-126, miR-130a, and miR-132), and anti-inflammatory miRNAs (miR124a, miR-125b), which could promote angiogenesis, activate fibroblasts, and induce the migration and proliferation of keratinocytes. Interestingly, Chen et al. [Citation85] found that proteins involved in the regulation of wound healing, particularly DMBT1 protein (a pro-angiogenic protein), were enriched in EVs released by human urinary-derived stem cells. Further functional assays showed that DMBT1 protein could improve angiogenesis capacity and accelerate wound repairing in diabetic mice.
Recently, Li et al. [Citation86] found that EVs from adipose-derived stem cells (ADSCs) overexpressing Nrf2 protein (a transcription factor) were able to accelerate cutaneous wound healing in diabetic rats by promoting vascularization. Functionally, these EVs could inhibit the cellular expression of both ROS and inflammatory cytokines (IL-1β, IL-6, and TNF-α), thereby contributing to the survival of endothelial progenitor cells (EPCs) in a high glucose environment and wound repairing process. Tao et al. [Citation87] showed that the impairment of angiogenesis in diabetes was significantly associated with the decrease of lncRNA-H19. To improve angiogenesis, they further used engineered EVs delivering lncRNA-H19. Interestingly, these EVs were internalized by endothelial cells, and then showed a strong ability to neutralize the regeneration-inhibiting effect of hyperglycaemia, remarkably accelerating the healing of chronic wounds in diabetic rats.
A study found that the EVs released from EPCs could significantly enhance proliferation, migration, and angiogenesis of endothelial cells (ECs) by activating the Erk1/2 pathway in diabetic rats, finally aiding both cutaneous wound healing and regeneration [Citation88]. Similarly, Li et al. [Citation89] also confirmed that EPC-derived EVs could stimulate ECs and enhance wound healing in diabetic rats by promoting the expression of angiogenesis-related molecules, including endothelial fibroblast growth factor 1 (FGF-1), vascular endothelial growth factor A (VEGF-A), and vascular endothelial growth receptor 2 (VEGFR-2). In addition, the EVs derived from platelet-rich plasma could effectively shorten the wound-healing time in diabetic rats. Functionally, these EVs were found to effectively induce proliferation and migration of endothelial cells and fibroblasts via activation of the Rho-YAP signal pathway in diabetic rat models, thereby promoting angiogenesis and re-epithelialisation of cutaneous wounds [Citation90]. However, the active molecules associated with diabetic wound healing were not revealed in EVs by three studies. Further research may be needed to determine the exact components before these EVs are used as therapeutic tools for patients with diabetes.
Taken together, these animal studies demonstrate that EVs may serve as a powerful instrument to effectively accelerate the healing of diabetic wounds and can be an extremely promising treatment system in the immediate future.
Erectile dysfunction related to T2DM
Recent studies stated that some EVs from stem cells could be used to improve erectile dysfunction in diabetic rats. It was reported that EVs from adipose-derived stem cells (ADSCs) could ameliorate the erectile dysfunction in diabetic rats. These vesicles were found to carry pro-angiogenic miRNAs (miR-126, miR-130a, and miR-132) and antifibrotic miRNAs (miR-let7b, miR-let7c), which were able to promote the angiogenesis of corpus cavernosum and decreased the fibrosis of cavernosum [Citation91]. Similarly, ADSC-derived EVs were also found to decrease apoptosis of endothelial and smooth muscle cells in the corpus cavernosum of diabetic rats. This decreased apoptosis could effectively ameliorate the erectile function in rat model of T2DM [Citation92]. Additionally, Ouyang et al. [Citation93] found that the erectile dysfunction of diabetic rats was effectively ameliorated after the EVs of urine-derived stem cells were transplanted into the corpora cavernosa of rats. RNA sequencing revealed that different miRNAs (miR-21-5p, let-7, and miR-10) that promote angiogenesis of corpora cavernosa were enriched in these EVs. Therefore, stem cell-derived EVs could be potential tools in the recovery of T2DM-related erectile dysfunction.
Cognitive impairment related with T2DM
EVs also play an important role in the treatment of cognitive impairment in T2DM. Nakano et al. [Citation94] found that rat bone marrow MSCs can improve the cognitive impairments of diabetic mice by repairing damaged neurons and astrocytes. Interestingly, cognitive impairments of mice were also significantly reversed by intracerebroventricular injection of MSCs-derived EVs. Additionally, the EVs derived from mice brain endothelial cells could be loaded into the brain’s ventricle of T2DM mice, leading to partial restoration of short-term memory function. The miR-146a in these EVs inhibited the gene expression of prion protein (PrPc) and alleviated the mice’s cognitive impairment induced by PrPc accumulation in brain cells [Citation95]. In summary, EVs have a positive role in improving T2DM-related cognitive impairment and may be applicable to humans in the future.
Diabetic nephropathy
Haemodialysis and kidney transplantation are common methods for treating DN, but they have limitations including high costs and low organ availability. It was reported that some MSC-derived EVs may be a promising therapy for DN. Nagaishi et al. [Citation96] found that MSCs contributed to the improvement of DN through the release of renal trophic factors including EVs. These vesicles from MSCs exerted an anti-apoptotic effect and protect tight junction structure in tubular epithelial cells. However, it was found that hyperglycaemia might induce abnormalities in MSCs resulting in loss of its therapeutic effects in diabetic mice. Interestingly, using of umbilical cord extracts containing EVs could circumvent the abnormalities of MSCs induced by hyperglycaemia, and increase the MSCs therapeutic effects on DN [Citation97]. Similarly, another study also confirmed that MSC-derived EVs could effectively improve DN, and showed that these EVs could enhance autophagy activity via inhibition of the mTOR pathway, contributing to the nephroprotective effects in DN rats [Citation98]. Recently, the renal function of diabetic mice was found to be significantly ameliorated by administration of EVs derived from human bone marrow MSCs and human liver stem-like cells (HLSCs). Further analysis showed that many miRNAs enriched in these EVs could inhibit renal fibrosis significantly via downregulation of pro-fibrotic genes [Citation99]. Therefore, MSC-derived EVs may be used as an important therapeutic strategy for DN in the future.
Diabetic cardiomyopathy and diabetic retinopathy
DCM and DR are common complications of T2DM. However, only few studies have focused on EVs as therapy for DCM and DR currently. Wang et al. [Citation100] showed that the HSP20-engineered EVs might be a novel therapeutic agent for DCM. The over-expressed HSP20-containing EVs from cardiomyocytes of HSP20-transgenic mice might be able to attenuate cardiac dysfunction, apoptosis, and fibrosis significantly, improving cardiovascular function in diabetic mice. Notably, some EVs have been applied in the therapy of other heart disease, including acute myocardial ischemic injury [Citation101], myocardial infarction [Citation102], and myocardial contractility [Citation103]. Using EVs in the treatment of DCM or DR may be a promising research direction in both animal models and humans.
Clinical application of EVs in other disease
EVs have gained significant attention as important mediators of disease biomarkers and therapeutic targets due to their close association with the development of diseases. In recent years, some studies reported that EVs might be potentially valuable biomarkers for non-invasive molecular diagnostics. Melo et al. [Citation104] showed that glypican-1 (+) EVs in the serum of patients with pancreatic cancer showed absolute specificity and sensitivity in distinguishing healthy subjects and patients with a benign pancreatic disease from patients with early- and late-stage pancreatic cancer. Hoshino et al. [Citation105] indicated that distinct integrins of tumour EVs could be used to predict organ-specific metastasis. Integrins α6β4 and α6β1 were associated with lung metastasis, while integrin αvβ5 was linked to liver metastasis. Furthermore, it was found that soluble E-cadherin was highly expressed in the EVs surface of ovarian cancer patients. These soluble E-cadherin+ EVs were closely related to malignant ascites formation and widespread peritoneal dissemination, which could potentially be a biomarker for diagnosis and prognosis of ovarian cancer patients [Citation106].
The EVs has raised great interest in the drug delivery field as carriers of functional RNA and proteins. Recently, some EVs showed broad prospects in macromolecular drug delivery and have been suggested in novel therapeutic approaches [Citation107]. A study demonstrated that EVs derived from mesenchymal cells could be engineered to carry siRNA that specifically target oncogenic KRASG12D, a common mutation in pancreatic cancer. These EVs could protect themselves from phagocytosis by monocytes due to the presence of CD47, and then inhibit the growth and metastasis of pancreatic cancer cells via specific targeting of KRASG12D [Citation108]. Interestingly, another study reported a method for targeted therapy of Duchenne muscular dystrophy based on modified EVs. A linker peptide CP05 was loaded onto EVs via binding to CD63, and then PMO (a targeted drug for treating Duchenne muscular dystrophy) and M12 (a muscle-targeting peptide) could bind to these CP05, respectively. Upon injecting into the mice with Duchenne muscular dystrophy, these EVs were highly enriched in the muscles and improved the function of muscle of mice [Citation109]. In order to fully exploit EVs therapeutic potential, Fuhrmann et al. [Citation110] engineered EVs with the tools of enzyme prodrug therapy (EPT). They embedded EVs into implantable biomaterials to achieve local delivery of therapeutics taking advantage of EPT. These vesicles were used as carriers for stabilizing enzymes in a hydrogel for local-controlled conversion of benign prodrugs to active anti-inflammatory compounds. Collectively, these results demonstrate the promise of using modularized EVs and engineered EVs as potential drug delivery.
Discussion
As an important means of cell-to-cell communication in vivo, EVs play critical roles in both physiological and pathological conditions. Accumulating evidence confirms that EVs are a potential cause in the pathogenesis of T2DM as well as an important factor for inducing diabetic complications. Some EVs can induce insulin resistance via activating inflammation, down-regulating GLUT4, and affecting insulin receptors. These vesicles can also participate in the development of diabetic complications including diabetic nephropathy, diabetic cardiomyopathy, and diabetic retinopathy through a series of molecular pathways. Generally, the development and progression of T2DM is related to some abnormal molecules carried by EVs, including proteins and miRNAs. It is still not clear what are the more essential ones carried by EVs in T2DM. This question may be more controversial.
Recent studies have shown that stem/progenitor cell-derived EVs have potential applications in T2DM rat/mouse models via the transfer of bioactive molecules. Particularly, miRNAs transferred by these EVs played important roles in the pathological angiogenesis of T2DM, including diabetic wounds, diabetic sexual dysfunction, diabetic nephropathy, and diabetic cardiomyopathy. Notably, these miRNAs could significantly improve the functions of related organs by promoting the proliferation of endothelial cells to affect angiogenesis. As a surrogate for stem/progenitor cell–based therapy, EVs have significant advantages when compared with these cells, including their relatively low immunological rejection, low probability of neoplastic transformation, and low abnormal differentiation. EVs have demonstrated great promise as natural drug delivery systems. Some therapeutic cargos, including small molecule drugs, RNA, proteins, and oligonucleotides, can be pre-loaded into chemically or biologically modified EVs. Even though liposomes are a relatively mature means of RNA delivery, unlike EVs, liposome treatment is limited by certain factors: high immunogenicity, low delivery efficiency, lack of specific targeting, and inability to cross the blood-brain barrier. Additionally, the bilayer membrane of the EV protects its cargo from clearance from the body, thereby extending their circulation half-life. Hence, EVs can be better exploited as drug carriers for therapeutic interventions in T2DM.
However, there are still some challenges in the treatment of T2DM with EVs. These challenges include large-scale production of desired EVs in a short time and improvement in the drug-loading efficiency in EVs. It is worth noting that most EV-based therapy have been performed in diabetic animal models. Clinical therapy for human requires high-purity EVs, which is limited by the current separation methods of EVs. In fact, the current separation methods have many shortcomings: inability to prepare a large number of high-purity EVs, inability to isolate the three subgroups of EVs, and the inability to separate specific EVs released by different cells. At this stage, there is still a reasonable doubt about the efficacy of EV in diabetic models, since the efficacy may be affected by contaminating proteins and RNAs in “EV fractions”. Certainly, these challenges in studying the role of EVs in T2DM are also present in other disease. Developing more efficient separation methods may be the key to solving these problems.
In summary, EVs play important roles in the pathological process and therapy of T2DM. Using EVs for the clinical treatment of T2DM is still challenging. It is necessary to further investigate the interplay between EVs and T2DM and provide a clear rationale for the application of EVs as a potential therapy for T2DM and its complications, thereby paving the way for curing diabetic patients in the future.
Geolocation information: Ganzhou, Jiangxi Province, China
Acknowledgments
We thank the members of our lab for providing some discussions. Yan He and Eric Handberg provided great help for polishing the manuscript.
Disclosure statement
The authors report no conflicts of interest in this work.
Additional information
Funding
References
- Punthakee Z, Goldenberg R, Katz P. Definition, classification and diagnosis of diabetes, prediabetes and metabolic syndrome. Can J Diabetes. 2018;42:10–14.
- Jia G, Whaley-Connell A, Sowers JR. Diabetic cardiomyopathy: A hyperglycaemia- and insulin-resistance-induced heart disease. Diabetologia. 2018;61:21–28.
- Schena FP, Gesualdo L. Pathogenetic mechanisms of diabetic nephropathy. J Am Soc Nephrol. 2005;16:30–33.
- Tang Y, Zhang MJ, Hellmann J, et al. Proresolution therapy for the treatment of delayed healing of diabetic wounds. Diabetes. 2013;62:618–627.
- Stitt AW, Curtis TM, Chen M, et al. The progress in understanding and treatment of diabetic retinopathy. Prog Retin Eye Res. 2016;51:156–186.
- Saedi E, Gheini MR, Faiz F, et al. Diabetes mellitus and cognitive impairments. World J Diabetes. 2016;7:412–422.
- Park Y, Colditz GA. Diabetes and adiposity: a heavy load for cancer. Lancet Diabetes Endocrinol. 2018;6:82–83.
- World Health Organization. Global report on diabetes. Working Papers; 2016.
- Cho NH, Shaw JE, Karuranga S, et al. Idf diabetes atlas: global estimates of diabetes prevalence for 2017 and projections for 2045. Diabetes Res Clin Pract. 2018;138:271–281.
- American Diabetes Association. (2) classification and diagnosis of diabetes: standards of medical care in diabetes-2018. Diabetes Care. 2018;41:13–27.
- Weyer C, Bogardus C, Mott DM, et al. The natural history of insulin secretory dysfunction and insulin resistance in the pathogenesis of type 2 diabetes mellitus. J Clin Invest. 1999;104:787–794.
- Nomura S, Suzuki M, Katsura K, et al. Platelet-derived microparticles may influence the development of atherosclerosis in diabetes mellitus. Atherosclerosis. 1995;116:235–240.
- Sabatier F, Darmon P, Hugel B, et al. Type 1 and type 2 diabetic patients display different patterns of cellular microparticles. Diabetes. 2002;51:2840–2845.
- Mocharla P, Briand S, Giannotti G, et al. Angiomir-126 expression and secretion from circulating cd34(+) and cd14(+) pbmcs: role for proangiogenic effects and alterations in type 2 diabetics. Blood. 2013;121:226–236.
- Mathiyalagan P, Liang Y, Kim D, et al. Angiogenic mechanisms of human cd34(+) stem cell exosomes in the repair of ischemic hindlimb. Circ Res. 2017;120:1466–1476.
- Fry CS, Kirby TJ, Kosmac K, et al. Myogenic progenitor cells control extracellular matrix production by fibroblasts during skeletal muscle hypertrophy. Cell Stem Cell. 2017;20:56–69.
- Song H, Li X, Zhao Z, et al. Reversal of osteoporotic activity by endothelial cell-secreted bone targeting and biocompatible exosomes. Nano Lett. 2019;19:3040–3048.
- Flaherty SE 3rd, Grijalva A, Xu X, et al. A lipase-independent pathway of lipid release and immune modulation by adipocytes. Science. 2019;363:989–993.
- Tkach M, Thery C. Communication by extracellular vesicles: where we are and where we need to go. Cell. 2016;164:1226–1232.
- Shao H, Im H, Castro CM, et al. New technologies for analysis of extracellular vesicles. Chem Rev. 2018;118:1917–1950.
- Pan BT, Johnstone RM. Fate of the transferrin receptor during maturation of sheep reticulocytes in vitro: selective externalization of the receptor. Cell. 1983;33:967–978.
- Kowal J, Tkach M, Thery C. Biogenesis and secretion of exosomes. Curr Opin Cell Biol. 2014;29:116–125.
- Van Niel G, D’angelo G, Raposo G. Shedding light on the cell biology of extracellular vesicles. Nat Rev Mol Cell Biol. 2018;19:213–228.
- Ostrowski M, Carmo NB, Krumeich S, et al. Rab27a and rab27b control different steps of the exosome secretion pathway. Nat Cell Biol. 2010;12:19–30.
- Wolf P. The nature and significance of platelet products in human plasma. Br J Haematol. 1967;13:269–288.
- Akers JC, Gonda D, Kim R, et al. Biogenesis of extracellular vesicles (ev): exosomes, microvesicles, retrovirus-like vesicles, and apoptotic bodies. J Neurooncol. 2013;113:1–11.
- Colombo M, Raposo G, Thery C. Biogenesis, secretion, and intercellular interactions of exosomes and other extracellular vesicles. Annu Rev Cell Dev Biol. 2014;30:255–289.
- FaW C, Brisson AR, Buzas EI, et al. Methodological guidelines to study extracellular vesicles. Circ Res. 2017;120:1632–1648.
- Gardiner C, Di Vizio D, Sahoo S, et al. Techniques used for the isolation and characterization of extracellular vesicles: results of a worldwide survey. J Extracell Vesicles. 2016;5:32945.
- Mateescu B, Kowal EJ, Van Balkom BW, et al. Obstacles and opportunities in the functional analysis of extracellular vesicle rna - an isev position paper. J Extracell Vesicles. 2017;6:1286095.
- Yamamoto CM, Murakami T, Oakes ML, et al. Uromodulin mrna from urinary extracellular vesicles correlate to kidney function decline in type 2 diabetes mellitus. Am J Nephrol. 2018;47:283–291.
- Musante L, Tataruch D, Gu D, et al. Proteases and protease inhibitors of urinary extracellular vesicles in diabetic nephropathy. J Diabetes Res. 2015;2015:1–14.
- Ravida A, Musante L, Kreivi M, et al. Glycosylation patterns of kidney proteins differ in rat diabetic nephropathy. Kidney Int. 2015;87:963–974.
- Musante L, Tataruch DE, Holthofer H. Use and isolation of urinary exosomes as biomarkers for diabetic nephropathy. Front Endocrinol (Lausanne). 2014;5:149.
- Karimi N, Cvjetkovic A, Jang SC, et al. Detailed analysis of the plasma extracellular vesicle proteome after separation from lipoproteins. Cell Mol Life Sci. 2018;75:2873–2886.
- Liga A, Vliegenthart AD, Oosthuyzen W, et al. Exosome isolation: A microfluidic road-map. Lab Chip. 2015;15:2388–2394.
- Reategui E, Van Der Vos KE, Lai CP, et al. Engineered nanointerfaces for microfluidic isolation and molecular profiling of tumor-specific extracellular vesicles. Nat Commun. 2018;9:175.
- Liu C, Xu X, Li B, et al. Single-exosome-counting immunoassays for cancer diagnostics. Nano Lett. 2018;18:4226–4232.
- Tian Y, Ma L, Gong M, et al. Protein profiling and sizing of extracellular vesicles from colorectal cancer patients via flow cytometry. ACS Nano. 2018;12:671–680.
- Shen W, Guo K, Adkins GB, et al. A single extracellular vesicle (ev) flow cytometry approach to reveal ev heterogeneity. Angew Chem Int Ed Engl. 2018;57:15675–15680.
- Sodar BW, Kittel A, Paloczi K, et al. Low-density lipoprotein mimics blood plasma-derived exosomes and microvesicles during isolation and detection. Sci Rep. 2016;6:24316.
- Thery C, Witwer KW, Aikawa E, et al. Minimal information for studies of extracellular vesicles 2018 (misev2018): a position statement of the international society for extracellular vesicles and update of the misev2014 guidelines. J Extracell Vesicles. 2018;7:1535750.
- Kowal J, Arras G, Colombo M, et al. Proteomic comparison defines novel markers to characterize heterogeneous populations of extracellular vesicle subtypes. Proc Natl Acad Sci U S A. 2016;113:968–977.
- Jeppesen DK, Fenix AM, Franklin JL, et al. Reassessment of exosome composition. Cell. 2019;177:428–445.
- Valadi H, Ekstrom K, Bossios A, et al. Exosome-mediated transfer of mrnas and micrornas is a novel mechanism of genetic exchange between cells. Nat Cell Biol. 2007;9:654–659.
- Maas SLN, Breakefield XO, Weaver AM. Extracellular vesicles: unique intercellular delivery vehicles. Trends Cell Biol. 2017;27:172–188.
- Chen C, Zong S, Wang Z, et al. Visualization and intracellular dynamic tracking of exosomes and exosomal mirnas using single molecule localization microscopy. Nanoscale. 2018;10:5154–5162.
- Truman-Rosentsvit M, Berenbaum D, Spektor L, et al. Ferritin is secreted via 2 distinct nonclassical vesicular pathways. Blood. 2018;131:342–352.
- Lindenbergh MFS, Stoorvogel W. Antigen presentation by extracellular vesicles from professional antigen-presenting cells. Annu Rev Immunol. 2018;36:435–459.
- Crewe C, Joffin N, Rutkowski JM, et al. An endothelial-to-adipocyte extracellular vesicle axis governed by metabolic state. Cell. 2018;175:695–708.
- Nocera AL, Mueller SK, Stephan JR, et al. Exosome swarms eliminate airway pathogens and provide passive epithelial immunoprotection through nitric oxide. J Allergy Clin Immunol. 2019;143:1525–1535.
- Sardar Sinha M, Ansell-Schultz A, Civitelli L, et al. Alzheimer’s disease pathology propagation by exosomes containing toxic amyloid-beta oligomers. Acta Neuropathol. 2018;136:41–56.
- Pavlyukov MS, Yu H, Bastola S, et al. Apoptotic cell-derived extracellular vesicles promote malignancy of glioblastoma via intercellular transfer of splicing factors. Cancer Cell. 2018;34:119–135.
- Nabet BY, Qiu Y, Shabason JE, et al. Exosome rna unshielding couples stromal activation to pattern recognition receptor signaling in cancer. Cell. 2017;170:352–366.
- Chen G, Huang AC, Zhang W, et al. Exosomal pd-l1 contributes to immunosuppression and is associated with anti-pd-1 response. Nature. 2018;560:382–386.
- Freeman DW, Noren Hooten N, Eitan E, et al. Altered extracellular vesicle concentration, cargo, and function in diabetes. Diabetes. 2018;67:2377–2388.
- Olefsky JM, Glass CK. Macrophages, inflammation, and insulin resistance. Annu Rev Physiol. 2010;72:219–246.
- Tateya S, Kim F, Tamori Y. Recent advances in obesity-induced inflammation and insulin resistance. Front Endocrinol (Lausanne). 2013;4:93.
- Deng Z, Poliakov A, Hardy RW, et al. Adipose tissue exosome-like vesicles mediate activation of macrophage-induced insulin resistance. Diabetes. 2009;58:2498–2505.
- Kranendonk ME, Visseren FL, Van Balkom BW, et al. Human adipocyte extracellular vesicles in reciprocal signaling between adipocytes and macrophages. Obesity. 2014;22:1296–1308.
- Zhang Y, Mei H, Chang X, et al. Adipocyte-derived microvesicles from obese mice induce m1 macrophage phenotype through secreted mir-155. J Mol Cell Biol. 2016;8:505–517.
- Song M, Han L, Chen FF, et al. Adipocyte-derived exosomes carrying sonic hedgehog mediate m1 macrophage polarization-induced insulin resistance via ptch and pi3k pathways. Cell Physiol Biochem. 2018;48:1416–1432.
- Kranendonk ME, Visseren FL, Van Herwaarden JA, et al. Effect of extracellular vesicles of human adipose tissue on insulin signaling in liver and muscle cells. Obesity. 2015;22:2216–2223.
- Zhang Y, Shi L, Mei H, et al. Inflamed macrophage microvesicles induce insulin resistance in human adipocytes. Nutr Metab (Lond). 2015;12:1–14.
- Ying W, Riopel M, Bandyopadhyay G, et al. Adipose tissue macrophage-derived exosomal mirnas can modulate in vivo and in vitro insulin sensitivity. Cell. 2017;171:372–384.
- Yu Y, Du H, Wei S, et al. Adipocyte-derived exosomal mir-27a induces insulin resistance in skeletal muscle through repression of pparγ. Theranostics. 2018;8:2171–2188.
- Wang L, Zhang B, Zheng W, et al. Exosomes derived from pancreatic cancer cells induce insulin resistance in c2c12 myotube cells through the pi3k/akt/foxo1 pathway. Sci Rep. 2017;7:5384.
- Yuasa T, Amo-Shiinoki K, Ishikura S, et al. Sequential cleavage of insulin receptor by calpain 2 and γ-secretase impairs insulin signalling. Diabetologia. 2016;59:2711–2721.
- Ce H, Pe S. Tgf-beta1-induced epithelial-to-mesenchymal transition and therapeutic intervention in diabetic nephropathy. Am J Nephrol. 2010;31:68–74.
- Delić D, Eisele C, Schmid R, et al. Urinary exosomal mirna signature in type II diabetic nephropathy patients. Plos One. 2016;11:e0150154.
- Jia Y, Guan M, Zheng Z, et al. Mirnas in urine extracellular vesicles as predictors of early-stage diabetic nephropathy. J Diabetes Res. 2016;2016:1–10.
- Wu X, Gao Y, Cui F, et al. Exosomes from high glucose-treated glomerular endothelial cells activate mesangial cells to promote renal fibrosis. Biol Open. 2016;5:484–491.
- Wu X, Gao Y, Xu L, et al. Exosomes from high glucose-treated glomerular endothelial cells trigger the epithelial-mesenchymal transition and dysfunction of podocytes. Sci Rep. 2017;7:9371.
- Wang X, Huang W, Liu G, et al. Cardiomyocytes mediate anti-angiogenesis in type 2 diabetic rats through the exosomal transfer of mir-320 into endothelial cells. J Mol Cell Cardiol. 2014;74:139–150.
- Davidson SM, Riquelme JA, Takov K, et al. Cardioprotection mediated by exosomes is impaired in the setting of type II diabetes but can be rescued by the use of non-diabetic exosomes in vitro. J Cell Mol Med. 2018;22:141–151.
- Hu J, Wang S, Xiong Z, et al. Exosomal mst1 transfer from cardiac microvascular endothelial cells to cardiomyocytes deteriorates diabetic cardiomyopathy. Biochim Biophys Acta Mol Basis Dis. 2018;1864:3639–3649.
- Mazzeo A, Beltramo E, Lopatina T, et al. Molecular and functional characterization of circulating extracellular vesicles from diabetic patients with and without retinopathy and healthy subjects. Exp Eye Res. 2018;176:69–77.
- Huang C, Fisher KP, Hammer SS, et al. Plasma exosomes contribute to microvascular damage in diabetic retinopathy by activating the classical complement pathway. Diabetes. 2018;67:1639–1649.
- Kamalden TA, Macgregor-Das AM, Kannan SM, et al. Exosomal microrna-15a transfer from the pancreas augments diabetic complications by inducing oxidative stress. Antioxid Redox Signal. 2017;27:913–930.
- Liu C, Ge HM, Liu BH, et al. Targeting pericyte-endothelial cell crosstalk by circular rna-cpwwp2a inhibition aggravates diabetes-induced microvascular dysfunction. Proc Natl Acad Sci U S A. 2019;116:7455–7464.
- Zhao H, Shang Q, Pan Z, et al. Exosomes from adipose-derived stem cells attenuate adipose inflammation and obesity through polarizing m2 macrophages and beiging in white adipose tissues. Diabetes. 2017;67:235–247.
- Tsukita S, Yamada T, Takahashi K, et al. Micrornas 106b and 222 improve hyperglycemia in a mouse model of insulin-deficient diabetes via pancreatic β-cell proliferation. Ebiomedicine. 2017;15:163–172.
- Sun Y, Shi H, Yin S, et al. Human mesenchymal stem cell derived exosomes alleviate type 2 diabetes mellitus by reversing peripheral insulin resistance and relieving beta-cell destruction. ACS Nano. 2018;12:7613–7628.
- Geiger A, Walker A, Nissen E. Human fibrocyte-derived exosomes accelerate wound healing in genetically diabetic mice. Biochem Biophys Res Commun. 2015;467:303–309.
- Chen CY, Rao SS, Ren L, et al. Exosomal dmbt1 from human urine-derived stem cells facilitates diabetic wound repair by promoting angiogenesis. Theranostics. 2018;8:1607–1623.
- Li X, Xie X, Lian W, et al. Exosomes from adipose-derived stem cells overexpressing nrf2 accelerate cutaneous wound healing by promoting vascularization in a diabetic foot ulcer rat model. Exp Mol Med. 2018;50:29.
- Tao SC, Rui BY, Wang QY, et al. Extracellular vesicle-mimetic nanovesicles transport lncrna-h19 as competing endogenous rna for the treatment of diabetic wounds. Drug Deliv. 2018;25:241–255.
- Zhang J, Chen C, Hu B, et al. Exosomes derived from human endothelial progenitor cells accelerate cutaneous wound healing by promoting angiogenesis through erk1/2 signaling. Int J Biol Sci. 2016;12:1472–1487.
- Li X, Jiang C, Zhao J. Human endothelial progenitor cells-derived exosomes accelerate cutaneous wound healing in diabetic rats by promoting endothelial function. J Diabetes Complications. 2016;30:986–992.
- Guo SC, Tao SC, Yin WJ, et al. Exosomes derived from platelet-rich plasma promote the re-epithelization of chronic cutaneous wounds via activation of yap in a diabetic rat model. Theranostics. 2017;7:81–96.
- Zhu LL, Huang X, Yu W, et al. Transplantation of adipose tissue-derived stem cell-derived exosomes ameliorates erectile function in diabetic rats. Andrologia. 2018;50:1–9.
- Chen F, Zhang H, Wang Z, et al. Adipose-derived stem cell-derived exosomes ameliorate erectile dysfunction in a rat model of type 2 diabetes. J Sex Med. 2017;14:1084–1094.
- Ouyang B, Xie Y, Zhang C, et al. Extracellular vesicles from human urine-derived stem cells ameliorate erectile dysfunction in a diabetic rat model by delivering proangiogenic microrna. Sex Med. 2019;7(2):241–250.
- Nakano M, Nagaishi K, Konari N, et al. Bone marrow-derived mesenchymal stem cells improve diabetes-induced cognitive impairment by exosome transfer into damaged neurons and astrocytes. Sci Rep. 2016;6:24805.
- Kalani A, Chaturvedi P, Maldonado C, et al. Dementia-like pathology in type-2 diabetes: a novel microrna mechanism. Mol Cell Neurosci. 2017;80:58–65.
- Nagaishi K, Mizue Y, Chikenji T, et al. Mesenchymal stem cell therapy ameliorates diabetic nephropathy via the paracrine effect of renal trophic factors including exosomes. Sci Rep. 2016;6:34842.
- Nagaishi K, Mizue Y, Chikenji T, et al. Umbilical cord extracts improve diabetic abnormalities in bone marrow-derived mesenchymal stem cells and increase their therapeutic effects on diabetic nephropathy. Sci Rep. 2017;7:8484.
- Ebrahim N, Ahmed IA, Hussien NI, et al. Mesenchymal stem cell-derived exosomes ameliorated diabetic nephropathy by autophagy induction through the mtor signaling pathway. Cells. 2018;7:E226
- Grange C, Tritta S, Tapparo M, et al. Stem cell-derived extracellular vesicles inhibit and revert fibrosis progression in a mouse model of diabetic nephropathy. Sci Rep. 2019;9:4468.
- Wang X, Gu H, Wei H, et al. Hsp20-mediated activation of exosome biogenesis in cardiomyocytes improves cardiac function and angiogenesis in diabetic mice. Diabetes. 2016;65:3111–3128.
- Luo Q, Guo D, Liu G, et al. Exosomes from mir-126-overexpressing adscs are therapeutic in relieving acute myocardial ischaemic injury. Cell Physiol Biochem. 2017;44:2105–2116.
- Vandergriff A, Huang K, Shen D, et al. Targeting regenerative exosomes to myocardial infarction using cardiac homing peptide. Theranostics. 2018;8:1869–1878.
- Mayourian J, Ceholski DK, Gorski PA, et al. Exosomal microrna-21-5p mediates mesenchymal stem cell paracrine effects on human cardiac tissue contractility. Circ Res. 2018;122:933–944.
- Melo SA, Luecke LB, Christoph K, et al. Glypican-1 identifies cancer exosomes and detects early pancreatic cancer. Nature. 2015;523:177–182.
- Hoshino A, Costa-Silva B, Shen TL, et al. Tumour exosome integrins determine organotropic metastasis. Nature. 2015;527:329–335.
- Tang MKS, Yue PYK, Ip PP, et al. Soluble e-cadherin promotes tumor angiogenesis and localizes to exosome surface. Nat Commun. 2018;9:2270.
- Ela S, Mager I, Breakefield XO, et al. Extracellular vesicles: biology and emerging therapeutic opportunities. Nat Rev Drug Discov. 2013;12:347–357.
- Kamerkar S, Lebleu VS, Sugimoto H, et al. Exosomes facilitate therapeutic targeting of oncogenic kras in pancreatic cancer. Nature. 2017;546:498–503.
- Gao X, Ran N, Dong X, et al. Anchor peptide captures, targets, and loads exosomes of diverse origins for diagnostics and therapy. Sci Transl Med. 2018;10:1–14.
- Fuhrmann G, Chandrawati R, Parmar PA, et al. Engineering extracellular vesicles with the tools of enzyme prodrug therapy. Adv Mater. 2018;30:e1706616.