ABSTRACT
Brain malignancies, including primary and metastatic brain tumours, are often associated with high mortality, reflecting a need for more effective diagnostics and therapeutics. Despite the different cells of origin, primary and metastatic brain tumours share the same microenvironment, which affects the survival mechanisms adopted by these tumours. Elucidating the mechanisms by which primary and metastatic brain tumours interact with the brain microenvironment can uncover potential targets for clinical applications. Extracellular vesicles have been recognized as intercellular communicators that can contribute to cancer progression and have shown promise as potential cancer biomarkers and therapeutics. Here, we outline the contribution of extracellular vesicles in the tumour–microenvironment interactions in primary and metastatic brain tumours with the goal of providing a guide for future translational research in this area.
Introduction
The survival and growth of cancerous cells in different organs highly depend on the ability of cells to adapt to and exploit the surrounding microenvironment. Cancer cells can develop a symbiotic relationship with different stromal compartments, such as endothelial cells, fibroblasts and immune cells to reverse tissue homeostasis toward a tumour-supporting microenvironment [Citation1]. This cooperation promotes an invasive phenotype in tumour cells and further supports tumour progression through enhancement of angiogenesis, alteration of the extracellular matrix (ECM) and facilitation of tumour immune evasion [Citation2]. The supporting role of the microenvironment in the brain is defined by specialized stromal cells such as astrocytes, microglia, and neurons, the distinct composition of the ECM and the presence of a blood–brain barrier. For detailed reviews of the brain tumour microenvironment, see Ref. [Citation3,Citation4].
Extracellular vesicles (EVs) have emerged as important means of intercellular communication that contribute to the interaction of tumour cells with the microenvironment and promote tumour progression [Citation5]. EVs include a broad range of vesicles including exosomes, microvesicles and oncosomes (collectively referred to as EVs in this review). The different subtypes of EVs vary in size and origin but are similar in that when secreted, they can be taken up by other cells, transfer their protein, RNA and DNA cargo, and change the behaviour of the recipient cells [Citation6]. Neoplasms in the brain include primary brain malignancies and secondary (metastatic) brain tumours, most commonly originated from lung cancer, breast cancer and melanoma. In primary brain tumours, transfer of EVs between tumour cells and stromal cells can facilitate tumour–microenvironment interactions and enhance processes such as immune modulation and angiogenesis [Citation7–Citation11]. Few studies have evaluated the role of EVs in the progression of metastatic brain tumours [Citation12–Citation15]. A better understanding of the EV-mediated interactions between metastatic tumour cells and the brain microenvironment can shed light on novel mechanisms driving brain metastasis.
Despite the notable differences between the cells of origin in primary and metastatic brain tumours, these two types of intracranial neoplasms share a microenvironment that while not identical, could be comparable to some extent. Through interactions with the brain microenvironment, metastatic tumour cells develop a number of growth and survival mechanisms, which can be similar to the mechanisms that are characteristically adopted by the transformed brain cells in a primary brain tumour. For instance, neurotrophin 3, a neural growth factor involved in the differentiation of neural progenitor cells during neurogenesis [Citation16], is highly expressed by primary brain tumours such as glioma to promote the survival and growth of brain tumour-initiating cells [Citation17]. Interestingly, it has been shown that breast cancer cells that metastasize to brain also hijack this mechanism to enhance their growth and survival [Citation18]. This and other comparable mechanisms prompted the question as to whether a parallel assessment of the EV literature with respect to tumour–microenvironmental interactions in primary and metastatic brain tumours could inform and enhance our understanding of the potential mechanisms of the EV contribution in brain cancer, in particular in less-studied metastatic brain tumours.
Here, we discuss the mechanisms by which bi-directional transfer of EVs derived from tumour cells and brain stromal cells modulates the different cellular and extracellular components of the tumour microenvironment to promote tumour development and progression. In this context, we compare the role of EVs in primary and metastatic brain tumours, with an aim to achieve novel insights into how a shared microenvironment can affect the EV-based mechanisms of adaptation and progression in primary and metastatic brain tumours of different origins. Understanding these EV-derived mechanisms can reveal novel diagnostic and therapeutic targets. We also discuss the current state of the clinical applications of EVs for diagnosis and treatment of primary and metastatic brain tumours. This review of literature is inclusive of EV studies on all types of primary and metastatic brain tumours that focus on the microenvironment component of the tumour.
Cellular components of the brain tumour microenvironment
Endothelial cells
One of the initial events associated with development of a malignancy is the establishment of a vascular network from the existing vasculature through the process of angiogenesis, which provides oxygen and nutrients critical for tumour cell survival and growth [Citation19]. Both primary and metastatic brain tumours depend on angiogenesis and are highly vascularized, making them potential targets for anti-angiogenic therapies, although their clinical efficacy has thus far been limited [Citation20,Citation21]. In addition to angiogenesis, mechanisms such as vessel co-option and vascular mimicry also contribute to providing a blood supply for brain tumours and have been described both for primary and metastatic brain tumours [Citation22,Citation23]. Angiogenic factors secreted from tumour cells such as vascular endothelial growth factor (VEGF) and fibroblast growth factor (FGF) along with different pro-inflammatory cytokines, proteases and miRNAs play pivotal roles in tumour angiogenesis [Citation19,Citation24,Citation25]. Tumour-derived EVs are also involved in the process of tumour angiogenesis. This function has most commonly been attributed to EV contents including pro-angiogenic proteins and miRNAs, which can directly trigger the activation of VEGF-dependent and -independent angiogenic pathways in endothelial cells [Citation26].
The angiogenic role of tumour-derived EVs in primary brain tumours has been investigated only in the context of glioma/glioblastoma, the most vascularized of all brain tumours (). Early studies demonstrated that EVs released from the U87 glioblastoma cell line contain angiogenic factors such as Interleukin-6 (IL-6), IL-8 and angiogenin and can induce tube formation in human microvascular endothelial cells [Citation27]. Other studies have demonstrated the presence of functional angiogenic proteins such as tissue factor, coagulation factor VIIa [Citation8], platelet-derived growth factor (PDGF) [Citation7], transforming growth factor beta (TGF-β) and C-X-C chemokine receptor type 4 (CXCR4) [Citation28] in glioblastoma-derived EVs. In addition, hypoxic glioblastoma EVs were demonstrated to be enriched in a number of angiogenic proteins and promoted stronger angiogenic phenotype in endothelial cells compared to normoxic glioblastoma EVs. Moreover, endothelial cells treated with hypoxic glioblastoma EVs were able to recruit more pericytes, in vitro and in vivo [Citation7]. Glioblastoma EVs contain a variety of proteases such as matrix metalloproteinase 2 (MMP-2), MMP-9, urokinase-type plasminogen activator (uPA) and tissue plasminogen activator (tPA) that increase the proliferation, migration and capillary tube formation of human brain endothelial cells [Citation28]. EVs isolated from the cerebrospinal fluid (CSF) of glioblastoma patients also induced angiogenesis in human umbilical vein endothelial cells [Citation29]. This effect was associated with the activation of the Akt/beta-catenin pathway; however, further investigation is required to evaluate the causal effect of EVs on the activation of this pathway. In accordance with these studies on primary brain tumours, breast cancer-derived-EVs were also shown to be involved in angiogenesis through the interaction of annexin AII on EVs with endothelial tPA [Citation30].
Figure 1. The effect of brain tumour-derived EVs on endothelial cells. EVs derived from primary and metastatic brain tumour cells can deliver angiogenic proteins and miRNAs and promote an angiogenic phenotype in endothelial cells.
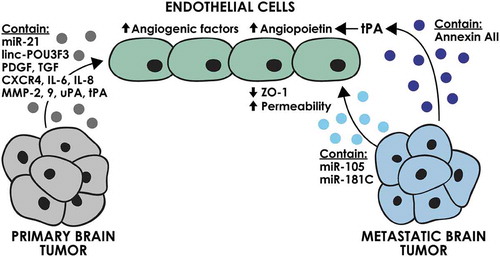
In addition to the pro-angiogenic protein cargo, a number of studies have indicated the importance of non-coding RNAs in the angiogenic function of glioblastoma-derived EVs (). For instance, EVs isolated from the CD133+ glioma stem cells derived from the U251 human glioblastoma cell line contain miR-21 that can trigger VEGF signalling in human brain endothelial cells in vitro [Citation31]. In contrast, miR-1 was demonstrated to be transferred between glioblastoma cells and tumour microenvironment through EVs and had a suppressive effect on angiogenesis, explaining its downregulation in glioblastoma patients [Citation11]. While the majority of studies on non-protein contents of EVs have focused on miRNAs, a variety of other non-coding small RNA species have been shown to be enriched in EVs derived from U251 cells. These EVs were shown to modulate the gene expression of human brain endothelial cells, which was considered to be a function of their small RNA content [Citation32]. It has also been reported that A172 glioma cell-derived EVs can transfer the long intergenic non-coding RNA, linc-POU3F3, to human brain endothelial cells, resulting in increased expression of FGF, FGFR and VEGF and increased angiogenesis both in vitro and in vivo [Citation33].
The potential contribution of non-coding RNAs in the angiogenic functions of EVs derived from metastatic brain tumours has yet to be evaluated. Comparative miRNA profile analysis of breast cancer cell lines demonstrated an overexpression of miR-210 in cells that specifically metastasize to brain versus the parental cell lines [Citation34]. miR-210 has been reported as a pro-angiogenic miRNA in normal adult mouse brain [Citation35]. The promising possibility of the involvement of EV miR-210 in breast cancer brain metastasis and angiogenesis requires further study.
In addition to tumour cells, several stromal cells in the tumour microenvironment such as bone marrow-derived tumour-associated macrophages and tissue-resident microglial cells play substantive roles in tumour angiogenesis [Citation36]. While the presence of angiogenic factors in stromal cell-derived EVs has been reported previously [Citation37], studies evaluating the importance of stromal cell-derived EVs in tumour angiogenesis are still lacking for both primary and metastatic brain tumours. Given the important role of the brain microenvironment in tumour angiogenesis, investigating the potential contribution of stromal cell-derived EVs in this process has the potential to uncover new opportunities for anti-angiogenic therapeutic approaches.
The interaction of EVs with the brain endothelium is not limited to increasing angiogenesis and vascularization. Breast cancer-derived EVs have been shown to facilitate brain metastasis through modulating the permeability of the brain endothelium (). EVs derived from MDA-MB-231 breast cancer cells were shown to decrease the expression of the tight junction molecule, ZO-1, via their cargo of miR-105 [Citation15]. Another study on brain-metastatic breast cancer cells also demonstrated an effect of EV miR-181c on the localization of actin filaments, resulting in increased permeability of the brain endothelium [Citation14]. The blood–brain barrier is a unique obstacle faced by the disseminated tumour cells metastasizing to the brain, making the potential effects of tumour-derived EVs on the integrity of this barrier of utmost importance.
Immune cells
A growing body of literature demonstrates that immune cells within the tumour microenvironment induce both stimulatory and inhibitory effects on tumour progression and resulting patient prognosis. The impact of these complicated tumour–immune cell interactions largely depends on the type and the location of the tumour [Citation38].
In the brain, resident immune cells including microglia and astrocytes along with infiltrating macrophages, neutrophils and lymphocytes form the immune component of the tumour microenvironment [Citation39]. In glioma, tumour-associated myeloid cells, including both recruited macrophages and resident microglia, constitute up to 40% of the cells in the tumour microenvironment [Citation40]. These cells facilitate the proliferation and invasion of glioma cells through secreting a variety of factors such as epidermal growth factor [Citation41]. In brain metastasis, recruitment of microglia/macrophages to the site of tumour has been shown to occur during early stages of brain metastasis [Citation42]. Immunohistochemistry analyses on human glioma samples have demonstrated significant infiltration of neutrophils in the majority of patients, particularly those with higher grade gliomas [Citation43]. While our understanding of the mechanisms by which tumour-infiltrating neutrophils contribute to tumour growth is incomplete, multiple studies have shown S100A proteins and elastase secreted by neutrophils can increase the proliferation and invasion of tumour cells, respectively [Citation44,Citation45]. Tumour-infiltrating lymphocytes have also been observed in patients with primary and metastatic brain tumours [Citation39]. The balance between the pro- and anti-tumour effects of tumour-infiltrating lymphocytes relies on the composition of this cell population in the tumour microenvironment. For instance, infiltration of CD4+ CD25+ FOXP3+ regulatory T cells in the tumour microenvironment has been reported for glioblastoma and brain metastasis in patient samples but not in benign brain tumours [Citation46].
The immunoregulatory potential of EVs was initially described in a fundamental study by Raposo and coworkers who demonstrated the secretion of major histocompatibility complex (MHC II)-containing EVs from B cells [Citation47]. This study along with many others [Citation48,Citation49] helped to establish the field of EV-based immunomodulation, which has grown to become an active area of investigation, particularly in cancer biology. Studies on EVs derived from immune cells have predominantly demonstrated anti-tumour effects. EVs derived from antigen presenting cells (APCs) contain MHC complexes and can directly or indirectly activate CD8+ and CD4+ T cells. Dendritic cell-derived EVs can also induce a pro-inflammatory cytokine profile. In contrast, EVs derived from tumour cells most commonly induce pro-tumourigenic immune modulations. For instance, populations of tumour-derived EVs were shown to suppress the activity of natural killer cells [Citation50] and to enhance the activity of myeloid-derived suppressor cells [Citation51], both leading to tumour immune surveillance and progression.
The immune modulating role of EVs derived from primary brain tumours has been evaluated for glioblastoma (). EVs derived from glioblastoma cell lines were reported to induce a tumour-supporting M2-like phenotype in microglia cells in vitro, as shown by upregulation of Arg-1. The expression of tumour-promoting cytokines such as IL-6 was increased, while immunogenic cytokines such as IL-16 were downregulated. Intravital microscopy showed that glioblastoma-derived EVs were taken up by microglial cells in vivo. This transfer of EVs led to the transfer of miR-21 and the suppression of its target, c-Myc, demonstrating the functionality of the EV transferred miRNA [Citation10]. This study supports the concept that the in vivo communication between glioblastoma and microglial cells can occur through tumour-derived EVs. The induction of the M2-like phenotype was also demonstrated in another study where patient-derived peripheral blood monocytes were treated with glioblastoma-derived EVs in vitro. The expression of M2 phenotype marker, CD163, as well as tumour-supporting cytokines such as IL-6, monocyte chemoattract protein-1 and VEGF was increased [Citation9]. In another study, transfer of cre recombinase mRNA through EVs derived from transplanted cre-expressing glioblastoma cells demonstrated the Gr1+ CD11b+ myeloid-derived suppressor cell population to be the prominent recipients of glioblastoma EVs in the tumour microenvironment [Citation52]. This transfer was suggested to potentially lead to an immunosuppressive microenvironment, but this was not directly demonstrated for glioblastoma EVs. T cells can also be affected by glioma-derived EVs.
Figure 2. The effect of brain tumour-derived EVs on immune cells. EVs derived from primary brain tumour cells can induce an M2 phenotype in microglia and decrease the cytotoxicity of the CD8 + T cells. Metastatic brain tumour EVs can activate microglia through the TLR receptor.
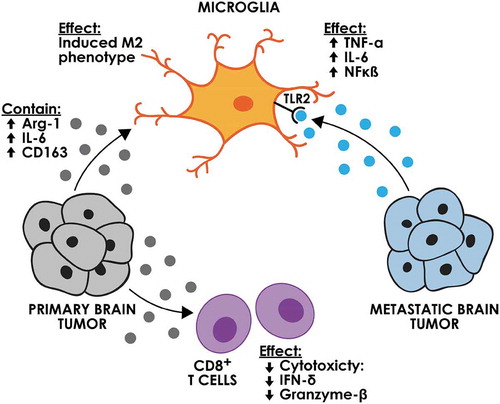
Interestingly, glioma EVs were shown to have a dose-dependent effect on the T cells isolated from peripheral blood mononuclear cells, inducing an immunosuppressive phenotype only in high concentrations [Citation53]. In another study, incubation of EVs isolated from plasma of glioblastoma patients with peripheral blood monocytes from healthy donors led to an inhibitory effect on the proliferation of CD4+ T cells [Citation54]. This inhibitory effect was further demonstrated to be mediated through EVs inducing a suppressive phenotype in CD14+ monocytes. More recently, it was shown that a subpopulation of EVs isolated from glioblastoma stem-like cells contain functional PDL-1 and were able to suppress T cell activation through direct interaction with PD1 [Citation55]. These authors also demonstrated that PDL-1 DNA was detected in plasma EVs from glioblastoma patients and correlated with tumour volume, suggestive of a potential biomarker.
Similar to primary brain tumours, metastatic brain tumours can communicate with macrophages/microglia in the brain through EVs (). EVs derived from breast tumours could interact with the Toll-like receptor 2 (TLR2) on macrophages and could stimulate the nuclear factor kappa-light-chain-enhancer of activated B cells (NF-κb) signalling pathway, leading to an increase in pro-inflammatory cytokines such as IL-6 and tumor necrosis factor alpha (TNF-α) [Citation56]. Consistent with this study, EVs released from drug-resistant MCF7 breast cancer cell line also stimulated the expression of IL-6 and decreased the chemotaxis ability of macrophages [Citation57]. The relationship between the EV-macrophage interactions and the development and progression of breast cancer brain metastasis was not evaluated in either of these studies and requires further investigation.
Overall, these studies demonstrate that EVs derived from both metastatic and primary tumours can induce a tumour-promoting phenotype in resident microglial cells and infiltrating macrophages. Given the prominent role of macrophages in brain tumour progression, these findings can potentially contribute to the development of actionable immunotherapeutic targets. The importance of the other components of the immune microenvironment in the brain cannot be neglected and studying the EV-based modulation of these components holds promise for novel discoveries regarding the role of the immune microenvironment in promoting primary and metastatic brain tumours.
Brain-specific cells
A variety of cell types including astrocytes, oligodendrocytes, neurons and microglia are specific to the brain and can play a role in the brain tumour microenvironment. In the brain microenvironment, astrocytes are one of the major host cells that establish interactions with primary and metastatic tumour cells and play major roles in promoting tumour cell survival and growth [Citation58,Citation59]. The current knowledge of the role of astrocyte-derived EVs in primary brain tumours is limited. EV transfer of alpha-crystallin B chain (CRYAB) from astrocytoma U373 cells was demonstrated to be involved in tumour cell resistance to apoptosis, a hallmark of cancer [Citation60]. Whether normal astrocytes in the tumour microenvironment can also promote tumour growth by transferring CRYAB-containing EVs to tumour cells is a potential mechanism that remains to be explored. Moreover, EVs released from astrocytes as well as the U87MG glioblastoma cell line contain mitochondrial DNA [Citation61]. A recent study demonstrated that cancer-associated fibroblasts could transfer their mitochondrial DNA to breast cancer cells via their EVs to induce an escape from metabolic dormancy in the cancer cells [Citation62]. Given these recent findings, it is of interest to determine whether the mitochondrial DNA found in astrocyte EVs can have any effect on the progression of primary and metastatic brain tumours.
In contrast to these potential promoting effects of astrocyte-derived EVs on tumour cells, tumour-derived EVs (oligodendroglioma G26/24 cell line) were shown to contain tumour necrosis factor-related apoptosis-inducing ligand (TRAIL) and could induce astrocyte apoptosis in vitro [Citation63] (). This process was suggested to be a mechanism by which tumour cells create space for expansion. The significance of this finding has not been evaluated in vivo.
Figure 3. The effect of brain tumour-derived EVs on brain-specific cells. Astrocytes can be affected by EVs from primary and metastatic brain tumours. Astrocyte-derived EVs can affect breast cancer brain metastasis through decreasing the PTEN expression.
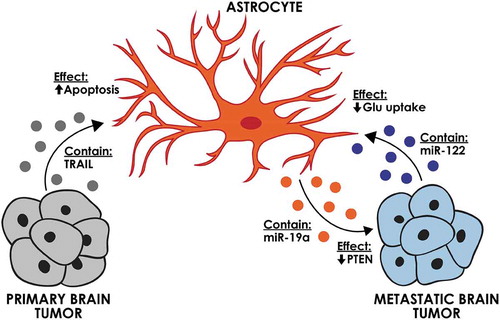
Studies on brain metastasis indicate that the EV-based interactions between astrocyte and metastatic tumour cells are mainly pro-tumourigenic (). Astrocyte EVs were shown to transfer miR-19a to metastatic breast cancer cells, which downregulated PTEN and enhanced the proliferation of the recipient tumour cells [Citation13]. A series of elaborate in vitro and in vivo experiments demonstrated that EV transfer of this miRNA is critical for the downregulation of PTEN, a common feature of breast cancer brain metastasis. In line with these studies, breast cancer derived-EVs were also shown to affect the metabolic status of brain metastases. It was demonstrated that tumour EVs can transfer miR-122 to astrocytes and reduce glucose uptake by these cells. This effect increased the availability of glucose in the brain for the metastatic tumour cells, enabling their initial expansion in the brain [Citation12].
Microglia and oligodendrocytes as well as neurons are among the other specialized cells in the brain that can contribute to tumour progression. The EV-based mechanisms of microglia–tumour interactions have been reported in multiple studies [Citation9,Citation10,Citation56,Citation57] as discussed in detail in the immune section of this review. The role of oligodendrocytes and neurons in these EV-based interactions is yet to be understood. Neurons have been reported to lack the ability to take up breast cancer-derived EVs within a 48-h incubation time [Citation12]. The possibility exists that the uptake of EVs by neurons occurs through fusion with the plasma membrane, which would be difficult to detect through tracing the EV fluorescent labels. Moreover, it must be noted that the effect of EVs on recipient cells does not merely rely on the uptake of EVs and can occur through surface interactions with the receptors on the cell membrane.
Extracellular components of the brain tumour microenvironment
The extracellular matrix, predominantly composed of proteins and glycosaminoglycans (GAGs), not only provides a scaffold for the growth and expansion of tumour cells but also functions in various ways to enhance tumour development. The ECM serves as a reservoir for different growth factors, which can be released and presented to their receptors upon modulation of the ECM. ECM components can also directly trigger signalling pathways in tumour cells promoting their survival, proliferation and invasion abilities [Citation64].
Brain ECM is unique both in terms of its components and structure. Similar to many other organs, the ECM that forms the basement membrane surrounding the brain vasculature, i.e. the perivascular ECM, is mainly comprised of fibrous proteins such as collagen, fibronectin and laminin, whereas the parenchymal ECM surrounding the neural and glial cells in the brain is abundant in hyaluronan and proteoglycans and has a very limited amount of fibrous proteins [Citation65]. Some of these components, such as brevicans, are specific to brain and nervous tissues and have been reported to enhance tumour progression and angiogenesis in high-grade primary brain tumours [Citation66]. ECM receptors such as CD44, the main receptor for hyaluronan, are also upregulated in glioma and meningioma cells, indicating the importance of tumour–ECM interactions for tumour progression [Citation67]. Unlike the other aspects of the microenvironment discussed in this review, the ECM composition surrounding the tumour differs between primary and metastatic brain tumours. Primary brain tumours often demonstrate an infiltrative pattern of growth while brain metastases, except for late-stage metastases, most frequently remain confined to the brain vasculature and grow along the vessels (i.e. vessel co-option). As a result, tumour–ECM interactions in primary tumours involve the parenchymal ECM, whereas in brain metastases, these interactions prominently occur within the fibrous perivascular ECM. This difference was indeed highlighted in a comparison of the ECM associated with glioblastoma and lung cancer brain metastasis, where a distinct pattern of ECM alterations was demonstrated for each type [Citation68]. Proteoglycans such as brevican and neurocans were more abundant in glioblastoma [Citation68]. These inherent differences should guide the design of studies on tumour ECM modulation in primary and metastatic brain tumours.
Potential involvement of tumour-derived EVs in ECM modulation is supported by proteomics studies that indicate the presence of a variety of adhesion molecules as well as proteases and glycosidases on tumour-derived EVs [Citation69]. Whether these EV proteins are functionally active and whether they play a role in cancer progression is a matter of active investigation. A comprehensive analysis of the interactions of pancreatic adenocarcinoma EVs with different ECM molecules in vitro demonstrated that EVs can bind to ECM through their integrins and CD44. Moreover, these EVs were shown to contain active proteases, such as MMPs and ADAMs, capable of degrading the ECM components and promoting tumour growth [Citation70].
The role of EVs derived from primary and metastatic brain tumours in ECM modulation has been studied to a limited extent (). In primary brain tumours, the direct role of tumour-derived EVs in modulation of ECM has yet to be elucidated. However, the current evidence suggests that EVs are capable of inducing ECM-modulating phenotypes in cells within the tumour microenvironment. EVs isolated from the glioblastoma U87 cell line increased the expression of MMP-14 in patient-derived microglia after 72 h of incubation, suggesting a potential indirect role for these EVs in modulation of the ECM [Citation9]. In metastatic brain tumours, evidence of both a direct and indirect role of EVs in modulation of ECM in the brain microenvironment has been reported. EVs derived from the breast cancer MDA-MB-231 cell line were shown to contain annexin AII, which induced an increase in plasmin activity and promoted angiogenesis [Citation30]. Annexin AII is a major co-receptor for plasminogen and tPA and is involved in the activation of plasmin that can degrade a variety of ECM component. Treatment of mice with Annexin AII+ EVs also led to an increase in the expression of MMP-9. The mechanism by which EVs induced this increase in expression has yet to be elucidated [Citation30].
Figure 4. The effect of brain tumour-derived EVs on extracellular matrix. EVs derived from primary and metastatic brain tumour cells can increase the degradation of extracellular matrix directly through activation of tPA and indirectly via an increase in expression of MMPs.
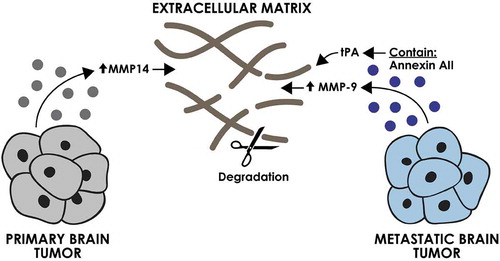
As evident by the limited number of studies on the role of EVs in ECM modulation in primary and metastatic brain tumours, many aspects of this process remain obscure. Further investigation of EV-based ECM modulation in the brain is necessary in order to understand the process involved in tumour progression. A comparison between the ability of EVs derived from primary and metastatic brain tumours to interact with and modulate the ECM can provide valuable insights into the mechanisms driving the different infiltrative behaviour of primary and metastatic brain tumours.
Clinical applications of EVs in primary and metastatic brain tumours
Along with their role as mediators of cancer progression, EVs have also shown promise in diagnostic and therapeutic applications. The potential of EVs as biomarkers for brain cancer detection was initially demonstrated when EVs isolated from the CSF of glioblastoma patients were shown to contain mutant EGFRvIII RNA [Citation27]. Interestingly, when compared to matched tissue samples, CSF EVs could identify two patients with EGFRvIII mutations that were previously identified to be negative for this mutation based on tissue analyses [Citation27]. Since then, EVs containing a variety of proteins and mRNAs/miRNAs have been correlated to the prognosis of patients with glioblastoma [Citation7,Citation27,Citation71,Citation72] suggesting their potential as biomarkers of disease progression. One such example is a study in which Annexin v-containing EVs derived from the sera of glioblastoma patients undergoing chemoradiation were demonstrated to have a positive association with earlier recurrence of the disease [Citation71]. EVs have also been introduced as potential companion diagnostics for monitoring treatment efficacy. O6-methylguanine DNA methyltransferase and alkylpurine-DNA-N-glycosylase are two enzymes that have been reported to inversely correlate with the efficacy of temozolomide treatment. Evaluation of the EVs isolated from the sera of seven glioblastoma patients demonstrated that alterations in the mRNA levels of these two enzymes can potentially be used as a predictive tool for treatment efficacy [Citation73]. Several studies have introduced novel techniques such as single EV analysis and microfluidic approaches to increase the sensitivity of EV detection for diagnostic purposes in glioblastoma and hold great promise for advancing this field [Citation74–Citation76].
EVs have also been used to transfer therapeutic mRNA [Citation77], miRNA [Citation78] and siRNA [Citation79] to glioma cells. For instance, the delivery of miR-9 and miR-146 in stem cell-derived EVs reduced resistance to temozolomide in vitro [Citation78] and decreased glioblastoma growth in a rat model [Citation80], respectively. A recent study demonstrated the benefits of combining miRNAs in EV-based cancer therapies to induce synergistic therapeutic function [Citation81]. The authors identified a combination of miRNAs downregulated in glioblastoma patients including miR-124, 128 and 137 that target mRNAs with interdependent functions in epigenetic regulation. Transfer of these miRNAs via EVs was demonstrated in vivo and were associated with prolonged survival when used in combination with chemotherapy in a murine glioblastoma model [Citation81]. The potential of EVs as cancer vaccines has also been tested. For instance, dendritic cells treated with tumour-derived EVs were shown to have the potential to be used as vaccines as they could induce anti-tumour T cell response in in vitro and in vivo glioma models [Citation82]. The absence of clinical studies on the application of EVs as diagnostic and therapeutic agents for metastatic brain tumours reflects the limited number of mechanistic EV studies of metastatic brain tumours. Advancing our understanding of EV-based tumour–microenvironment interactions in both primary and metastatic brain tumours has the potential to lead us to the development of effective EV-based clinical applications.
Conclusions
It is now widely acknowledged that extracellular vesicles can play a major role in tumour progression and invasion, by affecting both tumour cells and stromal cells in the tumour microenvironment and pre-metastatic niches. While many efforts have been made to determine the pathological role and the translational potential of EVs in primary brain tumours, the role of EVs in metastatic brain cancer has been far less studied and is yet equally significant. Moreover, the limited number of studies on the role of EVs in brain metastatic tumours has predominantly focused on breast cancer brain metastasis. Establishing animal models that can reliably recapitulate brain metastasis from other cancers that commonly metastasize to brain (e.g. lung cancer and melanoma) is a necessary step toward expanding the studies on the role of EVs in metastatic brain tumours. Similarly, the majority of studies on primary brain tumours involved high-grade tumours of glial origin such as glioblastoma, most likely due to the high incidence and aggressiveness of these tumours. As a result, there is a gap in our current knowledge of the behavior of EVs in low-grade glial primary brain tumours or primary tumours of other cellular origins such as neuronal tumours.
In this review, we have focused on the common microenvironment shared between primary and metastatic brain tumours to evaluate the effects of EVs on the development and progression of these types of cancer. The EV-based interactions of primary and metastatic tumour cells with the brain microenvironment support the prominent effects of tumour microenvironment on the mechanisms by which brain tumour cells, regardless of their origin, undertake to support their growth and progression. This perspective can direct the future EV research in metastatic and primary brain tumours toward actionable translational endpoints. It is important to note that most EV studies on primary and metastatic brain tumours predominantly rely on in vitro experiments and in vivo models that might not provide a representative recapitulation of the disease. Determining the physiologic relevance of the reported findings is a prerequisite to reach translation goals in the EV field.
Acknowledgements
The authors wish to express their gratitude to Dr. Xandra Breakefield (Massachusetts General Hospital) for providing valuable discussions and suggestions. The authors thank Kristin Johnson of the Vascular Biology Program at Boston Children’s Hospital for assistance with the illustrations.
Disclosure statement
No potential conflict of interest was reported by the authors.
Additional information
Funding
References
- Quail DF, Joyce JA. Microenvironmental regulation of tumor progression and metastasis. Nat Med. 2013;19(11):1423–11.
- Whiteside TL. The tumor microenvironment and its role in promoting tumor growth. Oncogene. 2008;27(45):5904–5912.
- Quail DF, Joyce JA. The microenvironmental landscape of brain tumors. Cancer Cell. 2017;31(3):326–341.
- Broekman ML, Maas SLN, Abels ER, et al. Multidimensional communication in the microenvirons of glioblastoma. Nat Rev Neurol. 2018;14(8):482–495.
- D’Asti E, Chennakrishnaiah S, Lee TH, et al. Extracellular vesicles in brain tumor progression. Cell Mol Neurobiol. 2016;36(3):383–407.
- Abels ER, Breakefield XO. Introduction to extracellular vesicles: biogenesis, RNA cargo selection, content, release, and uptake. Cell Mol Neurobiol. 2016;36(3):301–312.
- Kucharzewska P, Christianson HC, Welch JE, et al. Exosomes reflect the hypoxic status of glioma cells and mediate hypoxia-dependent activation of vascular cells during tumor development. Proc Natl Acad Sci U S A. 2013;110(18):7312–7317.
- Svensson KJ, Kucharzewska P, Christianson HC, et al. Hypoxia triggers a proangiogenic pathway involving cancer cell microvesicles and PAR-2-mediated heparin-binding EGF signaling in endothelial cells. Proc Natl Acad Sci U S A. 2011;108(32):13147–13152.
- de Vrij J, Maas SL, Kwappenberg KM, et al. Glioblastoma-derived extracellular vesicles modify the phenotype of monocytic cells. Int J Cancer. 2015;137(7):1630–1642.
- van der Vos KE, Abels ER, Zhang X, et al. Directly visualized glioblastoma-derived extracellular vesicles transfer RNA to microglia/macrophages in the brain. Neuro Oncol. 2016;18(1):58–69.
- Bronisz A, Wang Y, Nowicki MO, et al. Extracellular vesicles modulate the glioblastoma microenvironment via a tumor suppression signaling network directed by miR-1. Cancer Res. 2014;74(3):738–750.
- Fong MY, Zhou W, Liu L, et al. Breast-cancer-secreted miR-122 reprograms glucose metabolism in premetastatic niche to promote metastasis. Nat Cell Biol. 2015;17(2):183–194.
- Zhang L, Zhang S, Yao J, et al. Microenvironment-induced PTEN loss by exosomal microRNA primes brain metastasis outgrowth. Nature. 2015;527(7576):100–104.
- Tominaga N, Kosaka N, Ono M, et al. Brain metastatic cancer cells release microRNA-181c-containing extracellular vesicles capable of destructing blood-brain barrier. Nat Commun. 2015;6:6716.
- Zhou W, Fong MY, Min Y, et al. Cancer-secreted miR-105 destroys vascular endothelial barriers to promote metastasis. Cancer Cell. 2014;25(4):501–515.
- Zhu G, Sun C, Liu W. Effects of neurotrophin-3 on the differentiation of neural stem cells into neurons and oligodendrocytes. Neural Regen Res. 2012;7(19):1483–1487.
- Lawn S, Krishna N, Pisklakova A, et al. Neurotrophin signaling via TrkB and TrkC receptors promotes the growth of brain tumor-initiating cells. J Biol Chem. 2015;290(6):3814–3824.
- Louie E, Chen XF, Coomes A, et al. Neurotrophin-3 modulates breast cancer cells and the microenvironment to promote the growth of breast cancer brain metastasis. Oncogene. 2013;32(35):4064–4077.
- Harper J, Moses MA. Molecular regulation of tumor angiogenesis: mechanisms and therapeutic implications. Exs. 2006;96:223–268.
- Liu Y, Carson-Walter EB, Cooper A, et al. Vascular gene expression patterns are conserved in primary and metastatic brain tumors. J Neurooncol. 2010;99(1):13–24.
- Smith ER, Zurakowski D, Saad A, et al. Urinary biomarkers predict brain tumor presence and response to therapy. Clin Cancer Res. 2008;14(8):2378–2386.
- Soda Y, Marumoto T, Friedmann-Morvinski D, et al. Transdifferentiation of glioblastoma cells into vascular endothelial cells. Proc Natl Acad Sci U S A. 2011;108(11):4274–4280.
- Dey N, Barwick BG, Moreno CS, et al. Wnt signaling in triple negative breast cancer is associated with metastasis. BMC Cancer. 2013;13:537.
- Fang J, Shing Y, Wiederschain D, et al. Matrix metalloproteinase-2 is required for the switch to the angiogenic phenotype in a tumor model. Proc Natl Acad Sci U S A. 2000;97(8):3884–3889.
- Yang J, McNeish B, Butterfield C, et al. Lipocalin 2 is a novel regulator of angiogenesis in human breast cancer. Faseb J. 2013;27(1):45–50.
- Kholia S, Ranghino A, Garnieri P, et al. Extracellular vesicles as new players in angiogenesis. Vascul Pharmacol. 2016;86:64–70.
- Skog J, Wurdinger T, van Rijn S, et al. Glioblastoma microvesicles transport RNA and proteins that promote tumour growth and provide diagnostic biomarkers. Nat Cell Biol. 2008;10(12):1470–1476.
- Giusti I, Delle Monache S, Di Francesco M, et al. From glioblastoma to endothelial cells through extracellular vesicles: messages for angiogenesis. Tumour Biol. 2016;37(9):12743–12753.
- Liu S, Sun J, Lan Q. Glioblastoma microvesicles promote endothelial cell proliferation through Akt/beta-catenin pathway. Int J Clin Exp Pathol. 2014;7(8):4857–4866.
- Maji S, Chaudhary P, Akopova I, et al. Exosomal annexin II promotes angiogenesis and breast cancer metastasis. Mol Cancer Res. 2017;15(1):93–105.
- Sun X, Ma X, Wang J, et al. Glioma stem cells-derived exosomes promote the angiogenic ability of endothelial cells through miR-21/VEGF signal. Oncotarget. 2017;8(22):36137–36148.
- Li CC, Eaton SA, Young PE, et al. Glioma microvesicles carry selectively packaged coding and non-coding RNAs which alter gene expression in recipient cells. RNA Biol. 2013;10(8):1333–1344.
- Lang HL, Hu GW, Chen Y, et al. Glioma cells promote angiogenesis through the release of exosomes containing long non-coding RNA POU3F3. Eur Rev Med Pharmacol Sci. 2017;21(5):959–972.
- Camacho L, Guerrero P, Marchetti D. MicroRNA and protein profiling of brain metastasis competent cell-derived exosomes. PLoS One. 2013;8(9):e73790.
- Zeng L, He X, Wang Y, et al. MicroRNA-210 overexpression induces angiogenesis and neurogenesis in the normal adult mouse brain. Gene Ther. 2014;21(1):37–43.
- Zhu C, Kros JM, Cheng C, et al. The contribution of tumor-associated macrophages in glioma neo-angiogenesis and implications for anti-angiogenic strategies. Neuro Oncol. 2017;19:1435–1446.
- Taraboletti G, D’Ascenzo S, Borsotti P, et al. Shedding of the matrix metalloproteinases MMP-2, MMP-9, and MT1-MMP as membrane vesicle-associated components by endothelial cells. Am J Pathol. 2002;160(2):673–680.
- Fridman WH, Zitvogel L, Sautes-Fridman C, et al. The immune contexture in cancer prognosis and treatment. Nat Rev Clin Oncol. 2017;14(12):717–734.
- Hamilton A, Sibson NR. Role of the systemic immune system in brain metastasis. Mol Cell Neurosci. 2013;53:42–51.
- Hambardzumyan D, Gutmann DH, Kettenmann H. The role of microglia and macrophages in glioma maintenance and progression. Nat Neurosci. 2016;19(1):20–27.
- Coniglio SJ, Eugenin E, Dobrenis K, et al. Microglial stimulation of glioblastoma invasion involves epidermal growth factor receptor (EGFR) and colony stimulating factor 1 receptor (CSF-1R) signaling. Mol Med. 2012;18:519–527.
- Andreou KE, Soto MS, Allen D, et al. Anti-inflammatory microglia/macrophages as a potential therapeutic target in brain metastasis. Front Oncol. 2017;7:251.
- Fossati G, Ricevuti G, Edwards SW, et al. Neutrophil infiltration into human gliomas. Acta Neuropathol. 1999;98(4):349–354.
- Liang J, Piao Y, Holmes L, et al. Neutrophils promote the malignant glioma phenotype through S100A4. Clin Cancer Res. 2014;20(1):187–198.
- Iwatsuki K, Kumara E, Yoshimine T, et al. Elastase expression by infiltrating neutrophils in gliomas. Neurol Res. 2000;22(5):465–468.
- Jacobs JF, Idema AJ, Bol KF, et al. Regulatory T cells and the PD-L1/PD-1 pathway mediate immune suppression in malignant human brain tumors. Neuro Oncol. 2009;11(4):394–402.
- Raposo G, Nijman HW, Stoorvogel W, et al. B lymphocytes secrete antigen-presenting vesicles. J Exp Med. 1996;183(3):1161–1172.
- Denzer K, van Eijk M, Kleijmeer MJ, et al. Follicular dendritic cells carry MHC class II-expressing microvesicles at their surface. J Immunol. 2000;165(3):1259–1265.
- Thery C, Regnault A, Garin J, et al. Molecular characterization of dendritic cell-derived exosomes. Selective accumulation of the heat shock protein hsc73. J Cell Biol. 1999;147(3):599–610.
- Clayton A, Mitchell JP, Court J, et al. Human tumor-derived exosomes down-modulate NKG2D expression. J Immunol. 2008;180(11):7249–7258.
- Chalmin F, Ladoire S, Mignot G, et al. Membrane-associated Hsp72 from tumor-derived exosomes mediates STAT3-dependent immunosuppressive function of mouse and human myeloid-derived suppressor cells. J Clin Invest. 2010;120(2):457–471.
- Ridder K, Sevko A, Heide J, et al. Extracellular vesicle-mediated transfer of functional RNA in the tumor microenvironment. Oncoimmunology. 2015;4(6):e1008371.
- Hellwinkel JE, Redzic JS, Harland TA, et al. Glioma-derived extracellular vesicles selectively suppress immune responses. Neuro Oncol. 2016;18(4):497–506.
- Domenis R, Cesselli D, Toffoletto B, et al. Systemic T cells immunosuppression of glioma stem cell-derived exosomes is mediated by monocytic myeloid-derived suppressor cells. PLoS One. 2017;12(1):e0169932.
- Ricklefs FL, Alayo Q, Krenzlin H, et al. Immune evasion mediated by PD-L1 on glioblastoma-derived extracellular vesicles. Sci Adv. 2018;4(3):eaar2766.
- Chow A, Zhou W, Liu L, et al. Macrophage immunomodulation by breast cancer-derived exosomes requires Toll-like receptor 2-mediated activation of NF-kappaB. Sci Rep. 2014;4:5750.
- Jaiswal R, Johnson MS, Pokharel D, et al. Microparticles shed from multidrug resistant breast cancer cells provide a parallel survival pathway through immune evasion. BMC Cancer. 2017;17(1):104.
- Wasilewski D, Priego N, Fustero-Torre C, et al. Reactive astrocytes in brain metastasis. Front Oncol. 2017;7:298.
- Obenauf AC, Massague J. Surviving at a distance: organ specific metastasis. Trends Cancer. 2015;1(1):76–91.
- Kore RA, Abraham EC. Inflammatory cytokines, interleukin-1 beta and tumor necrosis factor-alpha, upregulated in glioblastoma multiforme, raise the levels of CRYAB in exosomes secreted by U373 glioma cells. Biochem Biophys Res Commun. 2014;453(3):326–331.
- Guescini M, Genedani S, Stocchi V, et al. Astrocytes and Glioblastoma cells release exosomes carrying mtDNA. J Neural Transm (Vienna). 2010;117(1):1–4.
- Sansone P, Savini C, Kurelac I, et al. Packaging and transfer of mitochondrial DNA via exosomes regulate escape from dormancy in hormonal therapy-resistant breast cancer. Proc Natl Acad Sci U S A. 2017;114(43):E9066–E75.
- Lo Cicero A, Schiera G, Proia P, et al. Oligodendroglioma cells shed microvesicles which contain TRAIL as well as molecular chaperones and induce cell death in astrocytes. Int J Oncol. 2011;39(6):1353–1357.
- Pickup MW, Mouw JK, Weaver VM. The extracellular matrix modulates the hallmarks of cancer. EMBO Rep. 2014;15(12):1243–1253.
- Lau LW, Cua R, Keough MB, et al. Pathophysiology of the brain extracellular matrix: a new target for remyelination. Nat Rev Neurosci. 2013;14(10):722–729.
- Varga I, Hutoczki G, Szemcsak CD, et al. Brevican, neurocan, tenascin-C and versican are mainly responsible for the invasiveness of low-grade astrocytoma. Pathol Oncol Res. 2012;18(2):413–420.
- Novak U, Kaye AH. Extracellular matrix and the brain: components and function. J Clin Neurosci. 2000;7(4):280–290.
- Virga J, Szemcsak CD, Remenyi-Puskar J, et al. Differences in extracellular matrix composition and its role in invasion in primary and secondary intracerebral malignancies. Anticancer Res. 2017;37(8):4119–4126.
- Sanderson RD, Bandari SK, Vlodavsky I. Proteases and glycosidases on the surface of exosomes: newly discovered mechanisms for extracellular remodeling. Matrix Biol. 2019 Jan;75–76:160–169.
- Mu W, Rana S, Zoller M. Host matrix modulation by tumor exosomes promotes motility and invasiveness. Neoplasia. 2013;15(8):875–887.
- Evans SM, Putt M, Yang XY, et al. Initial evidence that blood-borne microvesicles are biomarkers for recurrence and survival in newly diagnosed glioblastoma patients. J Neurooncol. 2016;127(2):391–400.
- Manterola L, Guruceaga E, Gallego Perez-Larraya J, et al. A small noncoding RNA signature found in exosomes of GBM patient serum as a diagnostic tool. Neuro Oncol. 2014;16(4):520–527.
- Shao H, Chung J, Lee K, et al. Chip-based analysis of exosomal mRNA mediating drug resistance in glioblastoma. Nat Commun. 2015;6:6999.
- Fraser K, Jo A, Giedt J, et al. Characterization of single microvesicles in plasma from glioblastoma patients. Neuro Oncol. 2019 May;21(5):606–615.
- Lee K, Fraser K, Ghaddar B, et al. Multiplexed profiling of single extracellular vesicles. ACS Nano. 2018;12(1):494–503.
- Shao H, Chung J, Balaj L, et al. Protein typing of circulating microvesicles allows real-time monitoring of glioblastoma therapy. Nat Med. 2012;18(12):1835–1840.
- Erkan EP, Senfter D, Madlener S, et al. Extracellular vesicle-mediated suicide mRNA/protein delivery inhibits glioblastoma tumor growth in vivo. Cancer Gene Ther. 2017;24(1):38–44.
- Munoz JL, Bliss SA, Greco SJ, et al. Delivery of functional anti-miR-9 by mesenchymal stem cell-derived exosomes to glioblastoma multiforme cells conferred chemosensitivity. Mol Ther Nucleic Acids. 2013;2:e126.
- Yang T, Fogarty B, LaForge B, et al. Delivery of small interfering RNA to inhibit vascular endothelial growth factor in zebrafish using natural brain endothelia cell-secreted exosome nanovesicles for the treatment of brain cancer. Aaps J. 2017;19(2):475–486.
- Katakowski M, Buller B, Zheng X, et al. Exosomes from marrow stromal cells expressing miR-146b inhibit glioma growth. Cancer Lett. 2013;335(1):201–204.
- Bhaskaran V, Nowicki MO, Idriss M, et al. The functional synergism of microRNA clustering provides therapeutically relevant epigenetic interference in glioblastoma. Nat Commun. 2019;10(1):442.
- Bu N, Wu H, Sun B, et al. Exosome-loaded dendritic cells elicit tumor-specific CD8+ cytotoxic T cells in patients with glioma. J Neurooncol. 2011;104(3):659–667.