ABSTRACT
Nutrients are essential resources for food production but are used inefficiently, and thereby they pollute inland and coastal waters and are lost into the oceans. Nutrient conservation by retention and consecutive reuse would prevent nutrient losses to the atmosphere and downstream ecosystems. We present Smart Nutrient Retention Networks (SNRNs) as a novel management approach to achieve nutrient conservation across networks of connected waterbodies through strategic water quality management. To present the key features of SNRNs, we review existing knowledge of nutrient retention processes in inland waters, water quality management options for nutrient conservation, and nutrient retention models to develop SNRNs. We argue that successful nutrient conservation, even at a local level, through SNRN management strategies requires clearly formulated goals and catchment-wide system understanding. Waterbody characteristics, such as hydraulic residence time and the presence of macrophytes, shape local nutrient retention with potential network-wide cascading effects of improved water quality and are therefore key targets of SNRN management strategies. Nutrient retention models that include the self-reinforcing feedback loop of ecological water quality, nutrient retention, and nutrient loading in networks of inland waters in relation to management options can support the development of SNRNs. We conclude that SNRNs can contribute to sustainable use of nutrients in human food production.
Introduction
Societal challenge
Fleets of vessels are dispatched, at great expense, to collect the dung of petrels and penguins at the South Pole, and the incalculable element of opulence which we have on hand, we send to the sea. All the human and animal manure which the world wastes, restored to the land instead of being cast into the water, would suffice to nourish the world. (Victor Hugo in “Les Misérables”: Volume V – Jean Valjean, Second Book, 1862.)
Figure 1. Global nitrogen (N; gray numbers) and phosphorus (P; blue numbers) flows in Tg per year (Smil Citation2000, Green et al. Citation2004, Van Drecht et al. Citation2005, Tysmans et al. Citation2013, Beusen et al. Citation2016). Nutrient inputs on land include natural and anthropogenic sources. Considerable amounts of these nutrients end up in inland waters. After entering inland waters, nutrients are retained within waterbodies or transported into the oceans. Note that these numbers represent estimates of global totals. Ratios between the different flows may differ strongly between individual river catchments (Tysmans et al. Citation2013). The question mark indicates an unknown fraction of retained nutrients that can be recovered in Smart Nutrient Retention Networks (SNRNs) to be reused on land for nutrient conservation. Color version available online.
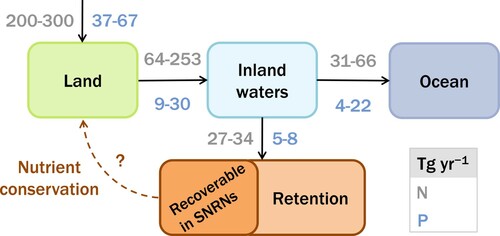
Networks of inland waters
Individual waterbodies (e.g., reservoirs, lakes, rivers, and wetlands) can form a network of inland waters, exchanging nutrients and other substances through hydrological connections (Teurlincx et al. Citation2019) and influencing each other’s chemical and ecological water quality (Tundisi et al. Citation1998, Carpenter and Lathrop Citation2014, Teurlincx et al. Citation2019). One waterbody can act as a net nutrient source or sink to downstream waterbodies (Zhang et al. Citation2012). Higher nutrient retention is associated with higher water quality (i.e., clear, submerged macrophyte-dominated vs. turbid, phytoplankton-dominated waters). Water flows through the network influence hydraulic residence times, which also determine nutrient retention (Van Gerven et al. Citation2017). When nutrient load reduction leads to an ecological regime shift from phytoplankton dominance to submerged macrophyte dominance in one waterbody, nutrient retention could increase locally, resulting in lower nutrient loading to connected waterbodies (discussed later; also see Supplemental Material A). This feedback could cause cascading effects of improved water quality and offer opportunities to benefit from local interventions on a network scale. However, despite the recognized importance of hydrological connections on nutrient flows and retention, water quality is generally assessed for individual waterbodies, and only a few model studies address the potential cascading effects of ecological water quality and nutrient retention in connected inland waters (Hilt et al. Citation2011, Van Gerven et al. Citation2017).
A novel approach for nutrient conservation through water quality management
Our aim was to fill this gap in the explicit use of inland water networks in water quality management by presenting what we named Smart Nutrient Retention Networks (SNRNs), a novel management approach for nutrient conservation through water quality management (). Here, we define nutrient conservation as the prevention of nutrient losses to the atmosphere and downstream ecosystems by nutrient retention and consecutive nutrient reuse. Nutrient retention comprises natural internal retention within and natural losses from waterbodies, as well as harvesting by humans. Retained nutrients are only conserved if reused, for example, as organic fertilizer to mitigate synthetic fertilizer production and application. Contrary to traditional water quality management that focuses on nutrient pollution reduction and local remediating interventions (Paerl et al. Citation2016, Strokal et al. Citation2020), SNRNs employ the biogeochemical nutrient retention potential of networks of inland waters to deal with nutrient pollution and promote nutrient conservation. Thus, we focus on a type of nutrient reuse that is often ignored.
Figure 2. Smart Nutrient Retention Networks (middle panel) have multiple dimensions, that should be considered to achieve the goal(s) set for the network of inland waters: minimized nutrient loss to the oceans, maximized nutrient retention in inland waters, good ecological water quality, or maximized reuse of nutrients retained within the network (bottom right panel). At the level of individual waterbodies, the ecological state and waterbody type influence the potential for nutrient retention (top left panel). Local interventions can influence this nutrient retention potential, for example, by adjusting the hydrology, changing the ecological state, or harvesting and reusing nutrient retaining ecosystem components (top right panel). At the catchment level, conditions apply which are beyond the scope of local water managers: external nutrient loading, configuration of hydrological connections, climate change, catchment-level legislation, and socioeconomic conditions (bottom left panel). Color version available online.
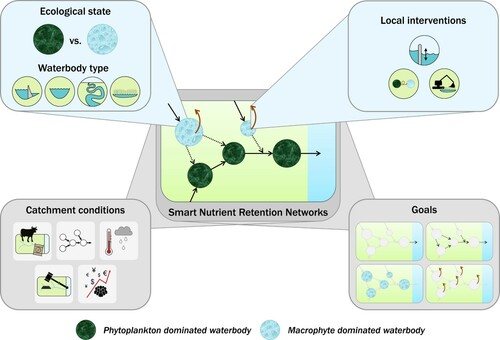
SNRNs aim to restore degraded inland waters, mitigate further ecological degradation, prevent nutrient losses into the ocean, and stimulate on-land reuse of nutrients harvested from inland waters (, bottom right panel) through smart combinations of catchment-specific interventions that account for cascading effects of improved water quality in connected waterbodies. Hence, in SNRNs the network of inland waters is managed in a smart way, where contextual adaptive management decisions are based on actual data and prior knowledge. This smart management includes manipulation of the system’s hydrology and ecological states to enhance nutrient retention. Moreover, nutrients in SNRNs are retained and reused by, for example, harvesting macrophytes, sediment, or fish (, top right panel). Overall, SNRNs could mitigate socioeconomic impacts related to nutrient pollution, which encompass chemical and ecological water quality degradation and unsustainable nutrient resource management.
In this paper, we explore current knowledge to develop SNRN management strategies. We specifically elaborate on (1) natural nutrient retention processes in individual waterbodies and networks of inland waters, (2) water quality management options for nutrient conservation, and (3) nutrient retention models. We focus on the northern temperate zone where strong human impacts on water systems (e.g., a legacy of intense nutrient enrichment) prevail, and regularly refer to examples from (sub)tropical regions where nutrient conservation is more common. Within the northern temperate zone, we expect that the principles of SNRNs can especially improve water quality and nutrient management in regions with highly modified and controlled water systems and many shallow lakes (with potential for macrophyte-dominated vs. phytoplankton-dominated states), such as lowland western Europe.
Nutrient retention processes in inland waters
In SNRNs, nutrient retention is maximized to benefit the whole catchment. The catchment covers the largest spatial scale of networks of inland waters, including connected waterbodies and the land draining into these waters. At the waterbody level, nutrients are either retained or flow freely with the water in dissolved or particulate forms such as detrital matter or phytoplankton (Teurlincx et al. Citation2019). Nutrient retention processes include (1) natural internal retention (e.g., long-term storage by sedimentation, burial of biomass, and P bound to mineral particles; Uhlmajnn and Horn Citation1992, Smolders et al. Citation2006, Finlay et al. Citation2013, Kong et al. Citation2019), (2) natural losses from the waterbody (e.g., denitrification and consumption by migrating waterfowl; Saunders and Kalff Citation2001, Doughty et al. Citation2016, Kong et al. Citation2019), or (3) harvesting by humans (e.g., in the form of macrophytes, sediment, or fish). Water management can influence nutrient retention pathways directly (e.g., harvesting by humans), and indirectly through ecosystem state management (e.g., increased denitrification by bank reshaping). In general, increases in nutrient retention processes could decrease the risk of harmful algal blooms. Some nutrient retention processes counteract nutrient conservation (e.g., N2 degases by denitrification), which enhances water quality but constitutes a loss process.
Waterbody characteristics
The hydraulic residence time of a waterbody promotes denitrification and sedimentation because it increases sediment–water contact (Ahlgren et al. Citation1988, Jansson et al. Citation1994, Saunders and Kalff Citation2001, Brett and Benjamin Citation2008, de Klein and Koelmans Citation2011; ). Waterbodies with a large volume and relatively low water discharge, such as large and dammed reservoirs, have long residence times (Maavara et al. Citation2015). In the meta-analysis by Saunders and Kalff (Citation2001), rivers had the largest average water discharge rate, followed by lakes and wetlands, whereas total N (TN) retention was largest for wetlands, followed by lakes and rivers. Although the average TN retention differed per waterbody type, it was similar in all waterbody types when correcting for discharge rates (Saunders and Kalff Citation2001). Higher water residence times in wetlands may partly be due to dense macrophyte stands that reduce flow velocity and increase sedimentation (Petticrew and Kalff Citation1992, Benoy and Kalff Citation1999, Saunders and Kalff Citation2001). In streams, macrophytes may lower water velocity and provide shelter, stimulating sedimentation (Svendsen and Kronvang Citation1993, Schulz et al. Citation2003). The highest nutrient storage potential in rivers was found within and downstream of areas with macrophytes (Svendsen and Kronvang Citation1993, Schulz et al. Citation2003). Especially in summer, areas with macrophytes tend to retain more nutrients, but weed cutting and autumn storm flows counteract this temporary storage through resuspension (Svendsen and Kronvang Citation1993).
Table 1. Illustrative examples of how waterbody type and system characteristics contribute to higher (+) or lower (−) N and P retention, focused on the northern temperate zone.
Additionally, stratification, relative nutrient processing rates, and the volume to surface area ratio of waterbodies influence the strength of nutrient retention processes (). Once a lake or reservoir stratifies and the hypolimnion becomes anoxic, it may act as a P source when the water column remixes or the outlet is at the bottom of a dam (Nürnberg Citation1984, Kõiv et al. Citation2011). The meta-analysis by Kõiv et al. (Citation2011) of 54 reservoirs and lakes (0–6.6 m deep) showed that stratifying waterbodies are generally deeper, and their P retention capacity decreases with relative depth. This redox-dependent P retention in lake sediment also depends on nitrate, sulfate, and particulate iron concentrations (Andersen Citation1982, Gächter and Müller Citation2003). Moreover, the balance, or even tradeoff, between biogeochemical nutrient processing rates and hydraulic residence time may determine net nutrient retention (Höhener and Gächter Citation1993, Powers et al. Citation2012, Schmadel et al. Citation2018), as described by the nutrient spiraling theory for individual streams (Newbold et al. Citation1981). For example, the combination of shorter hydraulic residence times, relatively invariant reaction times, and larger nutrient loadings during high-flow periods results in a lower N retention efficiency in rivers with higher streamflow variability (Ye et al. Citation2012). Additionally, direct P adsorption/desorption between water and sediment may be more important than sedimentation in shallow lakes with a relatively large sediment surface area to lake volume (Andersen Citation1997). Also, shallow wetlands with a large surface area likely retain N through denitrification, whereas those with a smaller surface area more likely retain P by sedimentation (Hansson et al. Citation2005).
Moreover, the amount of retained N and P tends to increase with nutrient loading (Prairie Citation1989, Saunders and Kalff Citation2001, Kõiv et al. Citation2011, Wang et al. Citation2020). Saunders and Kalff (Citation2001) found that N loading is an excellent statistical predictor for the magnitude of TN retention in wetlands and lakes. Thus for N retention, water discharge or hydraulic residence time and nutrient loading are important determining factors, although hydraulic residence time’s effect was strongest in the global lake dataset of Finlay et al. (Citation2013). Further, P sedimentation in 4 lakes worldwide was found to correlate with P loading and in-lake P concentrations (Prairie Citation1989). The relation between N and P retention and nutrient loading differs seasonally, however (Hansson et al. Citation2005). Moreover, increased total P concentrations can increase N retention by stimulating phytoplankton production, settling, and decomposition, which decreases dissolved oxygen concentrations and thereby increases denitrification rates (Finlay et al. Citation2013). For example, Finlay et al. (Citation2013) showed for a diverse and broadly representative set of lakes that N retention was >7 times higher in P-rich eutrophic lakes than in oligotrophic lakes, and similar trends were found by Donald et al. (Citation2015) for 12 reservoirs in Canada. This dependency of nutrient retention on nutrient loading and seasonal variation may partly be explained by the ecological configuration of the ecosystem in the growing season, with limited retention in oligotrophic systems with little macrophyte growth, increased retention in mesotrophic systems with strong macrophyte growth, and either high or low retention in eutrophic systems, depending on submerged macrophyte or phytoplankton dominance, respectively.
Especially when biological processes are dominant, nutrient retention shows seasonal patterns along with temperature dependencies of process rates (Kadlec and Reddy Citation2001, de Klein and Koelmans Citation2011, Wang et al. Citation2020). At higher temperatures, process rates (e.g., of denitrification) generally increase but oxygen levels tend to drop (Kadlec and Reddy Citation2001, Jeppesen et al. Citation2009). Low oxygen levels may result in lower nutrient retention by reducing nitrification and denitrification (Jeppesen et al. Citation2009, Özen et al. Citation2010) and enhancing sediment P release (Jensen and Andersen Citation1992). For example, in Lake Chaohu (China) low summer P retention is due to increased sediment P release and low winter N retention is due to low rates of denitrification (Wang et al. Citation2020). Reduced nutrient retention under winter conditions can also be explained by nitrate accumulation under ice cover and nutrient release by senescing vegetation (White and Bayley Citation2001). Moreover, snow changes hydrological conditions as it accumulates and causes long and intense runoff as it melts (German et al. Citation2003). During snowmelt, this process results in lower nutrient retention efficiencies (German et al. Citation2003), probably because of shorter hydraulic residence times.
Furthermore, climatic conditions such as precipitation, temperature, and degree of seasonality (Lewis Citation1996) are important for nutrient retention. Especially in tropical river lakes, seasonal precipitation may strongly affect hydraulic residence time (Lewis Citation1996) and thereby nutrient retention. In drier climates, less nutrient loading by runoff results in lower in-lake nutrient concentrations (Jeppesen et al. Citation2009, Citation2011, Özen et al. Citation2010). By contrast, in warmer climates more evaporation results in higher in-lake nutrient concentrations and higher chances of harmful algal blooms (Jeppesen et al. Citation2009, Citation2011, Özen et al. Citation2010). Similarly, macrophyte cover and critical nutrient loading levels at which lakes turn from clear to turbid are expected to decrease with warming (Jeppesen et al. Citation2009, Citation2011, Özen et al. Citation2010), and therefore lower nutrient retention is expected at higher temperatures. The balance between the effects of altered hydraulic residence time, nutrient loading, evaporation, and ecological state will determine the effect of different climates on nutrient retention.
The hydrological configuration of networks of inland waters influences local and network-wide nutrient retention. In systems with connected waterbodies, water quality, nutrient concentrations, and nutrient retention may differ depending on surrounding landscape and position in the catchment (Miranda et al. Citation2008, Schmadel et al. Citation2018, Teurlincx et al. Citation2019). For example, in river networks, large rivers retain more N than small rivers because they more effectively retain N per mean length of stream order, and they receive nutrients that are not retained in smaller rivers or that bypass them on land and directly enter the larger river (Wollheim et al. Citation2006). In general, network-wide nutrient retention is higher with abundant retaining waterbodies, such as lakes (Huttunen et al. Citation2016; see Supplemental Material B for an example of chains of lakes modeled with PCLake). Additionally, N removal increases with pond roundness and connectivity between ponds and streams (Schmadel et al. Citation2018). Also, when wetlands and floodplains temporarily connect to rivers by inundation, additional nutrient retention or release may occur (Noe and Hupp Citation2007). The multi-pond system above Chaohu Lake (China) exemplifies how connected waterbodies can influence nutrient retention (Yin et al. Citation1993). The ponds retain water, sediment, and nutrients and are used for rice field irrigation, which enhances nutrient recycling and retention on land. In 1993, Yin et al. (Citation1993) reported that from January to September overall, 99% and 98% of N and P loading was retained.
Macrophytes
An intricate balance of direct and indirect processes determines the net effect of macrophytes on nutrient retention (). Macrophytes directly contribute to nutrient retention by assimilation and consecutive burial of plant litter (Granéli and Solander Citation1988, Jansson et al. Citation1994, Clarke Citation2002, Kreiling et al. Citation2011), enhancing sedimentation and decreasing resuspension (Howard-Williams Citation1983, Clarke Citation2002, Zhu et al. Citation2015). Moreover, macrophytes indirectly contribute to nutrient retention by providing an attachment surface for nutrient consuming epiphytes and denitrifying bacteria (Howard-Williams and Allanson Citation1981, Weisner et al. Citation1994) and stimulating both P sorption and (coupled nitrification-)denitrification by sediment oxygenation (Risgaard-Petersen and Jensen Citation1997, Ottosen et al. Citation1999, Smolders et al. Citation2002, Kreiling et al. Citation2011, Vila-Costa et al. Citation2016). Macrophytes indirectly stimulate denitrification by (dissolved) organic carbon supply to the water or sediment and sediment nitrate penetration by root water uptake while inhibiting denitrification by competition for N and production and release of oxygen (Weisner et al. Citation1994). Moreover, shading by macrophytes may both stimulate and inhibit denitrification because it decreases oxygen production by photosynthesis and lowers the water temperature, respectively (Weisner et al. Citation1994). Furthermore, the net effect of macrophytes on nutrient retention depends on the time scale of measurement and the nutrient form (Carpenter and Lodge Citation1986). For example, macrophytes accumulate nutrients during spring and summer but release nutrients during their senescence (Landers Citation1982). And in general, macrophyte stands contribute to net particulate P retention and net dissolved P release (Carpenter and Lodge Citation1986).
Figure 3. Macrophytes directly and indirectly influence natural nutrient retention processes (white text in brown box) through macrophyte processes (light box). Nutrient uptake and assimilation followed by burial is the most direct route. Indirect influences are found through the effect of macrophytes on both chemical (e.g., dissolved oxygen [DO] and organic carbon [OC] concentration) and physical factors (dark boxes). Note the importance of seasonality, nutrient form (particulate/dissolved), macrophyte functional group, and species for macrophyte and nutrient retention processes. Color version available online.
![Figure 3. Macrophytes directly and indirectly influence natural nutrient retention processes (white text in brown box) through macrophyte processes (light box). Nutrient uptake and assimilation followed by burial is the most direct route. Indirect influences are found through the effect of macrophytes on both chemical (e.g., dissolved oxygen [DO] and organic carbon [OC] concentration) and physical factors (dark boxes). Note the importance of seasonality, nutrient form (particulate/dissolved), macrophyte functional group, and species for macrophyte and nutrient retention processes. Color version available online.](/cms/asset/21f8dc5c-f3f2-47e4-8fe9-518d56b83ed4/tinw_a_1870852_f0003_oc.jpg)
Macrophyte species and their functional groups may strongly influence their effect on nutrient retention. Larger vegetation types (e.g., helophytes) have more biomass and are therefore expected to contribute more to nutrient retention than low herbaceous vegetation (Sollie et al. Citation2008). Charophytes are more efficient nutrient sinks than vascular macrophytes because charophytes have lower decomposition rates and take up most of their nutrients from the water (vs. sediment) because of their larger shoot to root ratios (Kufel and Kufel Citation2002). Whether a macrophyte species is rooting and sessile or non-rooting and (similar to phytoplankton) flowing along with the water (Janssen et al. Citation2019b) is essential for their contribution to nutrient retention. Also, the functional group strongly influences denitrification rates. Especially, rooted macrophytes can increase denitrification by oxygenating the sediment, thereby enhancing coupled nitrification–denitrification (Risgaard-Petersen and Jensen Citation1997, Ottosen et al. Citation1999, Vila-Costa et al. Citation2016). Closed mats of floating macrophytes may increase denitrification and sediment P release through low dissolved oxygen concentrations (Veraart et al. Citation2011, Janssen et al. Citation2020). The net contribution to nutrient retention from other (e.g., submerged) macrophyte species is less evident (Søndergaard et al. Citation2001). In particular, dense macrophyte stands can seasonally cause a net sediment P release from low oxygen levels (e.g., during decomposition or by constrained water mixing) or increase pH due to high primary production (Søndergaard Citation1988, Frodge et al. Citation1991, Barko and James Citation1998).
Although outcomes among studies vary, they mostly show higher nutrient retention in macrophyte-dominated over phytoplankton-dominated shallow lakes (26 of 40 unique papers on nutrient retention in the review by Hilt et al. Citation2017). For example, from Veraart et al. (Citation2011) we expect about 10 times more N retention in a vegetated over an unvegetated state (with 12 h light). The relative contribution of assimilation in macrophytes to overall nutrient retention also varies, with 8–77% for N and 12–73% for P (Reddy and De Busk Citation1985, Kreiling et al. Citation2011, Veraart et al. Citation2011, Wang et al. Citation2013). Vegetation also enhances nutrient retention in streams (Balestrini et al. Citation2018). Moreover, the nutrient retention capacity of macrophytes explains one of the self-reinforcing (i.e., mathematically positive) feedback loops that self-maintain macrophyte-dominated versus phytoplankton-dominated states in shallow lakes (Scheffer et al. Citation1993), demonstrated by the ecosystem model PCLake (Supplemental Material A).
Feedback loops involving macrophytes as described by Scheffer et al. (Citation1993) for individual waterbodies can also emerge in hydrological networks. For example, Gillis et al. (Citation2014) showed that mangrove forests and seagrass beds retain nutrients, providing positive interactions with connected ecosystems (e.g., coral reefs) through reduced nutrient loadings. Such spatial effects of local nutrient retention by marine ecosystems could also be expected in networks of inland waters (Teurlincx et al. Citation2019) through a self-reinforcing feedback loop between nutrient loading, ecological water quality, and nutrient retention in networks of inland waters (). The underlying theory is that nutrient loading reduces water quality (i.e., increases the likelihood of phytoplankton dominance over macrophyte dominance and hence turbidity over clarity; Scheffer et al. Citation1993). Good water quality itself results in higher nutrient retention by the self-reinforcing feedback loop between macrophytes and water clarity within the waterbody. Finally, on the hydrological network level, the increased nutrient retention within the waterbody decreases nutrient loading to downstream waterbodies, where the feedback chain could repeat itself (i.e., has spatial cascading effects; Klose et al. Citation2020), resulting in a self-reinforcing feedback loop for the entire network of inland waters.
Figure 4. Schematic of the self-reinforcing (i.e., mathematically positive) feedback loop in networks of inland waters. Nutrient loading has a negative feedback on ecological water quality, water quality has a positive feedback on nutrient retention, and nutrient retention has a negative feedback on nutrient loading downstream, overall resulting in a self-reinforcing feedback loop that cascades down the network.
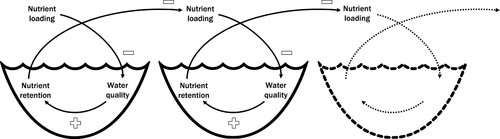
Water quality management options for nutrient conservation
Hydrological management
Hydrological management strategies to retain, harvest, and reuse more nutrients in and from inland waters can be applied with the ultimate goal to improve nutrient conservation in the entire catchment. Local changes in hydrology and nutrient retention affect downstream hydrology, ecology, and nutrient retention (Hilt et al. Citation2011, Jenny et al. Citation2014, Kondolf et al. Citation2014, Van Cappellen and Maavara Citation2016, Teurlincx et al. Citation2019, Maavara et al. Citation2020). Therefore, Hilt et al. (Citation2011) and Teurlincx et al. (Citation2019) argued for a hydrological network perspective and to tactically use local, upstream interventions. Local hydrological interventions may include water level and flow regulation by pumps, dams, and sluices, and dechannelization (Vörösmarty Citation1997, Stanley and Doyle Citation2002, Li et al. Citation2013, Kong et al. Citation2017, Fraaije et al. Citation2019, Maavara et al. Citation2020). These measures alter the hydraulic residence time and risk of hypoxia (Jenny et al. Citation2014), thereby influencing nutrient retention in the waterbody (Wang et al. Citation2020). Also, waterbody types may be altered; for example, dam construction can convert river sections into dammed reservoirs and strongly increase P retention (Tundisi et al. Citation1998, Vörösmarty et al. Citation2003). In addition to waterbody-level interventions, management of the hydrological network structure may stimulate net nutrient retention, for example, by increasing lateral connections between rivers and vegetated lakes or wetlands (Mitsch et al. Citation2008, Kreiling et al. Citation2011, Newcomer Johnson et al. Citation2016). More natural reconstruction of hydrological networks may have mutual benefits; for example, increased nutrient retention and flood protection are expected after (re)construction of waterways and flooding areas in the Dutch “Room for the River” and “Living with Water” projects (Van Gerven et al. Citation2009).
Macrophyte-focused management
Stimulation and preservation of macrophytes (Hilt et al. Citation2006) may enhance nutrient retention and ecological water quality (e.g., according to the European Water Framework Directive). However, macrophytes may cause problems for drinking water and hydropower production and block waterways for boating (Tundisi et al. Citation1998, Hilt et al. Citation2006). Yet the public perception on macrophyte establishment, in for example urban streams, is generally positive or neutral (Larned et al. Citation2006). Moreover, macrophyte-stimulating interventions may have multiple benefits, such as enhancing biodiversity, recreational value, fish spawning areas, and nutrient retention. At present, the benefits of paludiculture (i.e., wet agriculture/forestry on rewetted peatlands) are being explored (Vroom et al. Citation2018). Moreover, more vegetated water systems are being promoted or developed to support biodiversity, such as thousands of kilometers of nature-friendly banks in the Netherlands (ter Veld Citation2014). For lakes specifically, diverse management options to promote macrophyte dominance over phytoplankton dominance exist: flushing with cleaner water, nutrient load reductions beyond the lower critical nutrient load, and biomanipulation by fish removal (Janse et al. Citation2008, Bernes et al. Citation2015, Janssen et al. Citation2019b). Here, we highlight 2 more examples of macrophyte-focused management that can be applied for nutrient conservation: constructed wetlands and an engineering project for macrophyte harvesting and reuse.
Constructed wetlands
Constructed wetlands are wet systems created with macrophytes (and sediment), mostly used to treat wastewater. For example, reed filters in a stream bypass purify and store water at the estate of Lankheet (the Netherlands; Mulder and Querner Citation2008), and in (sub)tropical regions, common water hyacinth (Eichhornia crassipes) and water lettuce (Pistia stratiotes) are used to bioremediate multiple wastewater types (Reddy and D’angelo Citation1990, Kutty et al. Citation2009, Lu et al. Citation2010, Akinbile and Yusoff Citation2012). Howard-Williams (Citation1985) extensively reviewed N and P retention in wetlands and the early developments of constructed wetlands, and Wu et al. (Citation2015) comprehensively reviewed constructed wetland application and recent developments on their sustainable design. The nutrient retention effectiveness of constructed wetlands tends to decrease over time, especially for P (Mitsch et al. Citation2014), possibly explained by saturation of the soil and accumulation of detritus and plant biomass (Mitsch et al. Citation2012). To counteract saturation and accumulation effects and to maintain effectiveness of the constructed wetlands, these nutrient-retaining components should be harvested and used as a (nutrient) source elsewhere, thereby enhancing nutrient conservation (e.g., see Reddy and D’angelo Citation1990). For example, the new “bio-cascade water purification” approach applies knowledge of biogeochemical processes in soil, water, and macrophytes to prevent saturation effects in connected water basins and to conserve nutrients by harvesting helophytes and floating macrophytes (Kwakernaak et al. Citation2015). However, to meet increasingly strict water quality standards, research and development is still required for appropriate plant harvest and reuse strategies in constructed wetlands (Wu et al. Citation2015).
Harvesting
More nutrients could be reused by mowing macrophytes (Kuiper et al. Citation2017), dredging sediment, or fishing. Think of using reed as a building material (Köbbing et al. Citation2013), lake-dredged materials and decayed or processed water hyacinths as soil amendments (Sigua Citation2009, Aremu et al. Citation2012, Masto et al. Citation2013), or fish as a food source (Edwards et al. Citation1997, McIntyre et al. Citation2016, Kim et al. Citation2019). Such harvests are currently occurring but rarely considered for nutrient conservation or to combat eutrophication problems. For example, Tang and Xie (Citation2000) considered fish catches, like water outflow, a nutrient outflow. Nevertheless, fishing conserved 3–4% and 10% of the N and P loading, respectively. We noted one example of macrophyte harvesting to purposefully remove nutrients from a natural waterbody: an ecological engineering project in subtropical Lake Caohai (China; Wang et al. Citation2013). Here, seedlings of common water hyacinth were planted in constructed enclosures, harvested after growth, and processed into biogas and organic fertilizer. This process removed 76% of the inflowing TN, with 65% of the overall retained N in the form of macrophyte biomass. Wang et al. (Citation2013) concluded that “large scale utilization of E. crassipes for removal of N in the eutrophic lake [Caohai] is practicable,” thus the potential for nutrient conservation exists. However, harvesting should be applied with care to avoid drastic ecosystem disruptions (Van Zuidam and Peeters Citation2012, Kuiper et al. Citation2017) and the loss of indirect contributions of macrophytes to nutrient retention. Moreover, risks of contaminating food chains should be carefully assessed before reusing macrophytes and dredged materials (Beyer and Stafford Citation1993, Aremu et al. Citation2012).
Catchment-level nutrient management
Most water quality management measures are applied to individual waterbodies but would become more effective if embedded in catchment-wide management strategies, considering potentially cascading effects of improved water quality in networks of inland waters (). At the catchment level, additional measures can be taken by, for example, regional or (inter)national governing authorities (). These measures can target external nutrient loading (i.e., nutrients from diffuse and point sources in the catchment, which may eventually reach target waterbodies) and hydrological connections. These issues may be addressed by landscape-level legislation and enforcement (e.g., fertilizer application limits and wastewater treatment standards) or catchment-level management (e.g., changing hydrological network structure by (re)constructing waterways and adjusting macrophyte mowing schemes) but are beyond the scope of local water managers. Moreover, nutrient loading and retention are influenced by socioeconomic and climatic changes, for example land use change, population and economic growth, increasing temperatures, and changes in precipitation and runoff (Strokal et al. Citation2016). These (inter)national and global challenges require adjustments at even larger scales.
Nutrient retention models
SNRN management strategies can be designed with the help of nutrient retention models available at various spatial scales (Supplemental Material C). Nutrient retention models simulating individual waterbodies are often process-based (i.e., employing process rates and mechanistic insights to estimate nutrient retention; Van Gerven et al. Citation2009). For example, PCLake(+) (Janse Citation2005, Janssen et al. Citation2019a), PCDitch (Janse and Van Puijenbroek Citation1997, Janse Citation2005), and the GLOBIO-Wetlands model (under development; Janse et al. Citation2019) are process-based models from which nutrient retention processes and balances can be derived (Kong et al. Citation2019). These models include feedback loops between ecological states and nutrient retention and have been used to explore water quality management options (Janssen et al. Citation2019b). An example of a partly process-based model to analyze the effect of interventions on water quality is the Dutch KRW-Verkenner (Water Framework Directive Explorer). Users can themselves assign nutrient retention fractions of waterbodies, guided by meta-models for lowland streams, shallow lakes, and rivers that are statistically derived from the mechanistic process-based model AquaVenus (de Klein Citation2008, Van Gerven et al. Citation2009). Although some of the meta-models include the effect of macrophytes on nutrient retention, they do not include feedback loops between nutrient loading, ecological water quality, and nutrient retention (Van Gerven et al. Citation2009). Wetland models most explicitly cover the effect of macrophytes on nutrient retention and are usually more specific, for example, focusing on either N or P, or on a specific wetland type and location (Mitsch and Reeder Citation1991, Van Dam et al. Citation2007). Most of these and other waterbody-level nutrient retention models include macrophyte and/or phytoplankton presence (Supplemental Material C).
On the hydrological network level (i.e., global or catchment scale in Supplemental Material C), nutrient retention is often expressed as a fraction of the nutrient flow into the system that is retained and derived by statistical relationships to one or multiple waterbody characteristics. For example, the global model WorldQual derives P retention in waterbodies as a function for the whole catchment depending on hydraulic residence time (Fink et al. Citation2018). In other global models, the nutrient retention fraction is, in addition to hydraulic residence time, based on denitrification and sedimentation rates (Harrison et al. Citation2009, Beusen et al. Citation2016). GlobalNEWS includes denitrification in rivers as a function of water depth and travel time (Seitzinger et al. Citation2002), nutrient removal by water abstraction for irrigation and other human consumptive water use, and retention in dammed reservoirs as a function of hydraulic residence time and depth (Mayorga et al. Citation2010). The MARINA model builds on the latter global model but is specified for Chinese river catchments, with multiple subcatchments and channel section-specific nutrient retention (Strokal et al. Citation2016). In addition to the retention processes in GlobalNEWS, MARINA includes dissolved inorganic P retention in rivers by, for example, sedimentation and accumulation (Strokal et al. Citation2016). All these and other large-scale nutrient retention models exclude management options for individual waterbodies and generally disregard the effect of ecological state (Supplemental Material C).
To support the development of SNRNs, a model should include management options and the self-reinforcing feedback loop of ecological water quality, nutrient retention, and nutrient loading in networks of inland waters. Hence, existing nutrient retention models for individual waterbodies seem more promising than large-scale models. Especially, global-scale models strongly simplify nutrient retention, which may be justified because they are intended to identify global pollution hotspots and long-term water quality trends, whereas local-scale models are more often designed to assess management options (Tang et al. Citation2019). Large-scale models disregard alternative ecological states of lakes, which might be explained by their focus on rivers, and neither explicitly consider biogeochemical nutrient retention processes other than denitrification. Most local-scale models are more detailed and process-based but also have their limitations for modeling nutrient retention management at catchment-scale. For example, these models do not focus on nutrient retention, or they focus only on specific nutrients or waterbodies types (Supplemental Material C). The advantage of detailed, process-based models for SNRN management strategy development is that they more often include management options, primary producers, and potentially include feedback loops between ecological states and nutrient retention. By their mechanistic basis, these more process-based local-scale models can serve as a building block to consider cascading effects on a hydrological network scale; alternatively, their insights can be applied in large-scale models.
Toward Smart Nutrient Retention Network Management Strategies
The specific design of any SNRN management strategy depends on the configuration and state of the targeted network of inland waters, including its local waterbody types and their ecological states, and availability and efficacy of local intervention options (). Moreover, successful nutrient conservation through the implementation of SNRN management strategies requires an understanding of catchment conditions and clearly formulated goals (). Catchment conditions include biogeochemical and social system properties such as external nutrient loading, hydrological connections, climate change, catchment-level legislation, and socioeconomic conditions. Tailoring case-specific goals and strategies to such cross-sectoral catchment conditions is beyond the scope of water managers alone and requires partnerships of stakeholders and involvement of larger scale governing authorities (see catchment-level nutrient management discussion earlier).
Formulation of clear goals before implementation is a key task for responsible managers and stakeholders. It is crucial that the manager is aware of potential local and regional tradeoffs of interventions and between goals. For example, interventions may have contrary effects on N and P retention, denitrification can enhance water quality but counteract nutrient conservation, and increased flushing may locally improve water quality while deteriorating downstream ecosystems through increased nutrient loading. To avoid unforeseen tradeoffs, more complete and quantitative empirical studies must be conducted on the effect of macrophytes on nutrient retention at the level of networks of inland waters. Empirical examples and studies of effective, efficient, and safe nutrient harvesting and reuse can inform the further development of SNRNs, specifically to manage the risk of undesired changes in ecological state and nutrient retention potential. Ideally, the impact of SNRN management strategies on processes and goals aside from nutrient retention and conservation should be considered, as advocated by integrated water resource management approaches (Al-Jawad et al. Citation2019). For example, greenhouse gas emissions (Deemer et al. Citation2016, Chen et al. Citation2019), biodiversity loss (Dudgeon Citation2000, Hansson et al. Citation2005), and sediment starvation (Kondolf et al. Citation2014) should be considered.
SNRN management strategies can be designed conceptually, based on expert knowledge, or with the help of simulation models. Simulation models can provide outcomes of multiple scenarios for quantitative comparison, but integrated models must be developed to include (1) applications to networks of inland waters; (2) feedback between ecological states, nutrient retention, and nutrient loading; and (3) nutrient retention management options. Networks of inland waters could be modeled using a node-link schematization, building on existing waterbody models, as suggested by Teurlincx et al. (Citation2019). When a wide range of management options is available, such models could be run with optimization algorithms to determine the optimal solution (Al-Jawad et al. Citation2019, Strokal et al. Citation2020). While time-consuming and challenging, using an optimization approach could result in novel solutions to water management problems that might not be found intuitively.
Developing complex nutrient retention network models could drive significant improvements in network-level water management for nutrient conservation. Nonetheless, these developments are not necessary for water managers to start to include the core principles of SNRNs. SNRN principles could, for instance, be applied to the “River Basin Management Plans” required by the European Water Framework Directive (Griffiths Citation2002). Beyond Europe, considering nutrient retention in networks of inland waters may support sustainable water quality and nutrient management.
Human food production was long dependent on, and limited by, nutrient recycling by manure application on agricultural lands. With the onset of the industrial age, feeding a growing world population became increasingly dependent on unsustainable fertilization techniques that exploit mineral P deposits and marginally renewable geological stocks of fossil fuels for N fixation. Maintaining, and ensuring for all, the high living standards that result from such unsustainable agricultural practices, but within the means of the planet, is today’s greatest challenge (Raworth Citation2012). Smart Nutrient Retention Networks can be one component of a sustainable and just future as they are designed around biogeochemical nutrient cycling processes and contribute directly to at least 3 UN Sustainable Development Goals (SDGs): zero hunger (SDG 2), clean water and sanitation (SDG 6), and responsible consumption and production (SDG 12) (United Nations Citation2015).
Supplemental Material A
Download PDF (163.7 KB)Supplemental Material B
Download PDF (304.4 KB)Supplemental Material C
Download PDF (147.1 KB)Acknowledgements
We thank the Netherlands Organisation for Scientific Research (NWO) for their formulation of the project call Complexity – Programmable Self-organisation, which challenged and inspired us, and sparked the development of the concept of Smart Nutrient Retention Networks. We are grateful for the valuable suggestions of 2 reviewers and the editors to improve our manuscript, and Andrea Downing for proof-reading the revised version. This is publication 7176 of the Netherlands Institute of Ecology (NIOO-KNAW).
Disclosure statement
No potential conflict of interest was reported by the author(s).
Additional information
Funding
References
- Ahlgren I, Frisk T, Kamp-Nielsen L. 1988. Empirical and theoretical models of phosphorus loading, retention and concentration vs. lake trophic state. In: Persson G, Jansson M, editors. Phosphorus in freshwater ecosystems. New York (NY, USA): Springer; p. 285–303.
- Akinbile C, Yusoff MS. 2012. Assessing water hyacinth (Eichhornia crassopes) and lettuce (Pistia stratiotes) effectiveness in aquaculture wastewater treatment. Int J Phytoremediat. 14(3):201–211.
- Al-Jawad JY, Alsaffar HM, Bertram D, Kalin RM. 2019. A comprehensive optimum integrated water resources management approach for multidisciplinary water resources management problems. J Environ Manage. 239:211–224.
- Andersen JM. 1982. Effect of nitrate concentration in lake water on phosphate release from the sediment. Water Res. 16(7):1119–1126.
- Andersen T. 1997. The biogeochemical theatre – phosphorus cycling and phosphorus household in lakes. In: Andersen T, editor. Pelagic nutrient cycles. New York (NY, USA): Springer; p. 207–213.
- Aremu A, Ojoawo S, Alade G. 2012. Water hyacinth (Eichhornia crassipes) culture in sewage: nutrient removal and potential applications of bye-products. Transnat J Sci Technol. 2(7):103–114.
- Balestrini R, Delconte CA, Palumbo MT, Buffagni A. 2018. Biotic control of in-stream nutrient retention in nitrogen-rich springs (Po Valley, Northern Italy). Ecol Eng. 122:303–314.
- Barko JW, James WF. 1998. Effects of submerged aquatic macrophytes on nutrient dynamics, sedimentation, and resuspension. In: Jeppesen E, Sondergaard Martin, Sondergaard Morton, Christoffersen K, editors. The structuring role of submerged macrophytes in lakes. New York (NY, USA): Springer; p. 197–214.
- Beaulieu JJ, Smolenski RL, Nietch CT, Townsend-Small A, Elovitz MS, Schubauer-Berigan JP. 2014. Denitrification alternates between a source and sink of nitrous oxide in the hypolimnion of a thermally stratified reservoir. Limnol Oceanogr. 59(2):495–506.
- Benoy GA, Kalff J. 1999. Sediment accumulation and Pb burdens in submerged macrophyte beds. Limnol Oceanogr. 44(4):1081–1090.
- Bernes C, Carpenter SR, Gårdmark A, Larsson P, Persson L, Skov C, Speed JD, Van Donk E. 2015. What is the influence of a reduction of planktivorous and benthivorous fish on water quality in temperate eutrophic lakes? A systematic review. Env Evid. 4(1):7.
- Beusen AHW, Bouwman AF, Van Beek LPH, Mogollon JM, Middelburg JJ. 2016. Global riverine N and P transport to ocean increased during the 20th century despite increased retention along the aquatic continuum. Biogeosciences. 13(8):2441–2451.
- Beyer WN, Stafford C. 1993. Survey and evaluation of contaminants in earthworms and in soils derived from dredged material at confined disposal facilities in the great lakes region. Environ Monit Assess. 24(2):151–165.
- Brett MT, Benjamin MM. 2008. A review and reassessment of lake phosphorus retention and the nutrient loading concept. Freshwater Biol. 53(1):194–211.
- Carpenter SR, Lathrop RC. 2014. Phosphorus loading, transport and concentrations in a lake chain: a probabilistic model to compare management options. Aquat Sci. 76(1):145–154.
- Carpenter SR, Lodge DM. 1986. Effects of submersed macrophytes on ecosystem processes. Aquat Bot. 26:341–370.
- Chen Z, Huang P, Zhang Z. 2019. Interaction between carbon dioxide emissions and eutrophication in a drinking water reservoir: a three-dimensional ecological modeling approach. Sci Total Environ. 663:369–379.
- Chislock MF, Doster E, Zitomer RA, Wilson AE. 2013. Eutrophication: causes, consequences, and controls in aquatic ecosystems. Nature Educ Knowl. 4(4):10.
- Clarke SJ. 2002. Vegetation growth in rivers: influences upon sediment and nutrient dynamics. Prog Phys Geogr. 26(2):159–172.
- Deemer BR, Harrison JA, Li S, Beaulieu JJ, DelSontro T, Barros N, Bezerra-Neto JF, Powers SM, Dos Santos MA, Vonk JA. 2016. Greenhouse gas emissions from reservoir water surfaces: a new global synthesis. BioScience. 66(11):949–964.
- de Jonge VN, Elliott M, Orive E. 2002. Causes, historical development, effects and future challenges of a common environmental problem: eutrophication. In: Orive E, Elliott M, de Jonge VN, editors. Nutrients and eutrophication in estuaries and coastal waters. New York (NY, USA): Springer; p. 1–19.
- de Klein JJM. 2008. From ditch to delta: nutrient retention in running waters [dissertation]; Wageningen (the Netherlands): Wageningen University.
- de Klein JJM, Koelmans AA. 2011. Quantifying seasonal export and retention of nutrients in west European lowland rivers at catchment scale. Hydrol Process. 25(13):2102–2111.
- Donald DB, Parker BR, Davies J-M, Leavitt PR. 2015. Nutrient sequestration in the Lake Winnipeg watershed. J Great Lakes Res. 41(2):630–642.
- Doughty CE, Roman J, Faurby S, Wolf A, Haque A, Bakker ES, Malhi Y, Dunning JB, Svenning J-C. 2016. Global nutrient transport in a world of giants. P Natl Acad Sci USA. 113(4):868–873.
- Dudgeon D. 2000. The ecology of tropical Asian rivers and streams in relation to biodiversity conservation. Annu Rev Ecol Syst. 31(1):239–263.
- Edwards P, Little D, Yakupitiyage A. 1997. A comparison of traditional and modified inland artisanal aquaculture systems. Aquac Res. 28(10):777–788.
- Fink G, Alcamo J, Flörke M, Reder K. 2018. Phosphorus loadings to the world’s largest lakes: sources and trends. Global Biogeochem Cy. 32(4):617–634.
- Finlay JC, Small GE, Sterner RW. 2013. Human influences on nitrogen removal in lakes. Science. 342(6155):247–250.
- Fraaije RG, Poupin C, Verhoeven JT, Soons MB. 2019. Functional responses of aquatic and riparian vegetation to hydrogeomorphic restoration of channelized lowland streams and their valleys. J Appl Ecol. 56(4):1007–1018.
- Frodge JD, Thomas GL, Pauley GB. 1991. Sediment phosphorus loading beneath dense canopies of aquatic macrophytes. Lake Reserv Manage. 7(1):61–71.
- Gächter R, Müller B. 2003. Why the phosphorus retention of lakes does not necessarily depend on the oxygen supply to their sediment surface. Limnol Oceanogr. 48(2):929–933.
- German J, Svensson G, Gustafsson L-G, Vikström M. 2003. Modelling of temperature effects on removal efficiency and dissolved oxygen concentrations in stormwater ponds. Water Sci Technol. 48(9):145–154.
- Gillis LG, Bouma TJ, Jones CG, Van Katwijk MM, Nagelkerken I, Jeuken CJL, Herman PMJ, Ziegler AD. 2014. Potential for landscape-scale positive interactions among tropical marine ecosystems. Mar Ecol Prog Ser. 503:289–303.
- Granéli W, Solander D. 1988. Influence of aquatic macrophytes on phosphorus cycling in lakes. Hydrobiologia. 170(1):245–266.
- Green PA, Vörösmarty CJ, Meybeck M, Galloway JN, Peterson BJ, Boyer EW. 2004. Pre-industrial and contemporary fluxes of nitrogen through rivers: a global assessment based on typology. Biogeochemistry. 68(1):71–105.
- Griffiths M. 2002. The European water framework directive: an approach to integrated river basin management. European Water Association (EWA); European Water Manage Online. 5:1–14.
- Hansson LA, Brönmark C, Anders Nilsson P, Åbjörnsson K. 2005. Conflicting demands on wetland ecosystem services: nutrient retention, biodiversity or both? Freshwater Biol. 50(4):705–714.
- Harrison JA, Maranger RJ, Alexander RB, Giblin AE, Jacinthe P-A, Mayorga E, Seitzinger SP, Sobota DJ, Wollheim WM. 2009. The regional and global significance of nitrogen removal in lakes and reservoirs. Biogeochemistry. 93(1–2):143–157.
- Heisler J, Glibert PM, Burkholder JM, Anderson DM, Cochlan W, Dennison WC, Dortch Q, Gobler CJ, Heil CA, Humphries E. 2008. Eutrophication and harmful algal blooms: a scientific consensus. Harmful Algae. 8(1):3–13.
- Hilt S, Brothers S, Jeppesen E, Veraart AJ, Kosten S. 2017. Translating regime shifts in shallow lakes into changes in ecosystem functions and services. BioScience. 67(10):928–936.
- Hilt S, Gross EM, Hupfer M, Morscheid H, Mählmann J, Melzer A, Poltz J, Sandrock S, Scharf E-M, Schneider S. 2006. Restoration of submerged vegetation in shallow eutrophic lakes – a guideline and state of the art in Germany. Limnologica. 36(3):155–171.
- Hilt S, Köhler J, Kozerski HP, van Nes EH, Scheffer M. 2011. Abrupt regime shifts in space and time along rivers and connected lake systems. Oikos. 120(5):766–775.
- Höhener P, Gächter R. 1993. Prediction of dissolved inorganic nitrogen (DIN) concentrations in deep, seasonally stratified lakes based on rates of DIN input and N removal processes. Aquat Sci. 55(2):112–131.
- Howard-Williams C. 1983. Wetlands and watershed management: the role of aquatic vegetation. J Limnol Soc S Afr. 9(2):54–62.
- Howard-Williams C. 1985. Cycling and retention of nitrogen and phosphorus in wetlands: a theoretical and applied perspective. Freshwater Biol. 15(4):391–431.
- Howard-Williams C, Allanson BR. 1981. Phosphorus cycling in a dense Potamogeton pectinatus L. bed. Oecologia. 49(1):56–66.
- Hugo V. 1862. Les Misérables: volume V; Jean Valjean: Second book – The intestine of the leviathan; Chapter I - The land impoverished by the sea. New York (NY): Carleton.
- Huttunen I, Huttunen M, Piirainen V, Korppoo M, Lepistö A, Räike A, Tattari S, Vehviläinen B. 2016. A national-scale nutrient loading model for Finnish watersheds—VEMALA. Environ Model Assess. 21(1):83–109.
- Janse JH. 2005. Model studies on the eutrophication of shallow lakes and ditches [dissertation]. Wageningen (the Netherlands): Wageningen University.
- Janse JH, Domis LNDS, Scheffer M, Lijklema L, Van Liere L, Klinge M, Mooij WM. 2008. Critical phosphorus loading of different types of shallow lakes and the consequences for management estimated with the ecosystem model PCLake. Limnologica. 38(3–4):203–219.
- Janse JH, van Dam AA, Hes EMA, de Klein JJM, Finlayson CM, Janssen ABG, van Wijk D, Mooij WM, Verhoeven JTA. 2019. Towards a global model for wetlands ecosystem services. Curr Opin Env Sust. 36:11–19.
- Janse JH, Van Puijenbroek PJTM. 1997. PCDitch, a model for eutrophication and the development of vegetation in ditches. Report no 703715 004. RIVM Bilthoven; p. 121.
- Janssen ABG, Hilt S, Kosten S, de Klein JJM, Paerl HW, Van de Waal DB. 2020. Shifting states, shifting services: linking regime shifts to changes in ecosystem services of shallow lakes. Freshwater Biol. 66(1):1–12.
- Janssen ABG, Teurlincx S, Beusen AHW, Huijbregts MAJ, Rost J, Schipper AM, Seelen LMS, Mooij WM, Janse JH. 2019a. PCLake+: a process-based ecological model to assess the trophic state of stratified and non-stratified freshwater lakes worldwide. Ecol Modell. 396:23–32.
- Janssen ABG, van Wijk D, van Gerven LPA, Bakker ES, Brederveld RJ, DeAngelis DL, Janse JH, Mooij WM. 2019b. Success of lake restoration depends on spatial aspects of nutrient loading and hydrology. Sci Total Environ. 679:248–259.
- Jansson M, Andersson R, Berggren H, Leonardson L. 1994. Wetlands and lakes as nitrogen traps. Ambio. 23(6):320–325.
- Jenny JP, Arnaud F, Alric B, Dorioz JM, Sabatier P, Meybeck M, Perga ME. 2014. Inherited hypoxia: a new challenge for reoligotrophicated lakes under global warming. Global Biogeochem Cy. 28(12):1413–1423.
- Jensen HS, Andersen FO. 1992. Importance of temperature, nitrate, and pH for phosphate release from aerobic sediments of four shallow, eutrophic lakes. Limnol Oceanogr. 37(3):577–589.
- Jeppesen E, Kronvang B, Meerhoff M, Søndergaard M, Hansen KM, Andersen HE, Lauridsen TL, Liboriussen L, Beklioglu M, Özen A. 2009. Climate change effects on runoff, catchment phosphorus loading and lake ecological state, and potential adaptations. J Environ Qual. 38(5):1930–1941.
- Jeppesen E, Kronvang B, Olesen JE, Audet J, Søndergaard M, Hoffmann CC, Andersen HE, Lauridsen TL, Liboriussen L, Larsen SE. 2011. Climate change effects on nitrogen loading from cultivated catchments in Europe: implications for nitrogen retention, ecological state of lakes and adaptation. Hydrobiologia. 663(1):1–21.
- Kadlec RH, Reddy KR. 2001. Temperature effects in treatment wetlands. Water Environ Res. 73(5):543–557.
- Kim M, Mam K, Sean V, Try V, Brooks A, Thay S, Hav V, Gregory R. 2019. A manual for community fish refuge-rice field fisheries system management in Cambodia. Phnom Penh (Cambodia): Fisheries Administration.
- Klose AK, Karle V, Winkelmann R, Donges JF. 2020. Emergence of cascading dynamics in interacting tipping elements of ecology and climate. Roy Soc Open Sci. 7(6):200599.
- Köbbing JF, Thevs N, Zerbe S. 2013. The utilisation of reed (Phragmites australis): a review. Mires Peat. 13:1–14.
- Kõiv T, Nõges T, Laas A. 2011. Phosphorus retention as a function of external loading, hydraulic turnover time, area and relative depth in 54 lakes and reservoirs. Hydrobiologia. 660(1):105–115.
- Kondolf G, Rubin Z, Minear J. 2014. Dams on the Mekong: cumulative sediment starvation. Water Resour Res. 50(6):5158–5169.
- Kong X, He Q, Yang B, He W, Xu F, Janssen ABG, Kuiper JJ, Van Gerven LPA, Qin N, Jiang Y, et al. 2017. Hydrological regulation drives regime shifts: evidence from paleolimnology and ecosystem modeling of a large shallow Chinese lake. Global Change Biol. 23(2):737–754.
- Kong X, Zhan Q, Boehrer B, Rinke K. 2019. High frequency data provide new insights into evaluating and modeling nitrogen retention in reservoirs. Water Res. 166:115017.
- Kreiling RM, Richardson WB, Cavanaugh JC, Bartsch LA. 2011. Summer nitrate uptake and denitrification in an upper Mississippi River backwater lake: the role of rooted aquatic vegetation. Biogeochemistry. 104(1–3):309–324.
- Kufel L, Kufel I. 2002. Chara beds acting as nutrient sinks in shallow lakes – a review. Aquat Bot. 72(3–4):249–260.
- Kuiper JJ, Verhofstad MJJM, Louwers ELM, Bakker ES, Brederveld RJ, van Gerven LPA, Janssen ABG, de Klein JJM, Mooij WM. 2017. Mowing submerged macrophytes in shallow lakes with alternative stable states: battling the good guys? Environ Manage. 59(4):619–634.
- Kutty SRM, Ngatenah S, Isa MH, Malakahmad A. 2009. Nutrients removal from municipal wastewater treatment plant effluent using Eichhornia crassipes. World Acad Sci Eng Technol. 60:1115–1123.
- Kwakernaak C, Jansen PC, Kempen M, Smolders F, Rheenen H. 2015. A smart solution for flooding, drought and water pollution. Water Matters. 2:44–47.
- Landers DH. 1982. Effects of naturally senescing aquatic macrophytes on nutrient chemistry and chlorophyll a of surrounding waters. Limnol Oceanogr. 27(3):428–439.
- Larned ST, Suren AM, Flanagan M, Biggs BJF, Riis T. 2006. Macrophytes in urban stream rehabilitation: establishment, ecological effects, and public perception. Restor Ecol. 14(3):429–440.
- Lewis WMJ. 1996. Tropical lakes: how latitude makes a difference. In: Schiemer F, Boland KT, editors. Perspectives in tropical limnology. Amsterdam (the Netherlands): Academic Publishing; p. 43–64.
- Li Y, Tang C, Wang C, Anim DO, Yu Z, Acharya K. 2013. Improved Yangtze River diversions: are they helping to solve algal bloom problems in lake Taihu, China? Ecol Eng. 51:104–116.
- Lu Q, He ZL, Graetz DA, Stoffella PJ, Yang X. 2010. Phytoremediation to remove nutrients and improve eutrophic stormwaters using water lettuce (Pistia stratiotes L.). Environ Sci Pollut Res. 17(1):84–96.
- Maavara T, Chen Q, Van Meter K, Brown LE, Zhang J, Ni J, Zarfl C. 2020. River dam impacts on biogeochemical cycling. Nat Rev Earth Environ. 1(2):103–116.
- Maavara T, Parsons CT, Ridenour C, Stojanovic S, Dürr HH, Powley HR, Van Cappellen P. 2015. Global phosphorus retention by river damming. P Natl Acad Sci USA. 112(51):15603–15608.
- Masto RE, Kumar S, Rout T, Sarkar P, George J, Ram L. 2013. Biochar from water hyacinth (Eichornia crassipes) and its impact on soil biological activity. Catena. 111:64–71.
- Mayorga E, Seitzinger SP, Harrison JA, Dumont E, Beusen AHW, Bouwman A, Fekete BM, Kroeze C, Van Drecht G. 2010. Global nutrient export from WaterSheds 2 (NEWS 2): model development and implementation. Environ Model Softw. 25(7):837–853.
- McIntyre PB, Liermann CAR, Revenga C. 2016. Linking freshwater fishery management to global food security and biodiversity conservation. P Natl Acad Sci USA. 113(45):12880–12885.
- Miranda LE, Habrat MD, Miyazono S. 2008. Longitudinal gradients along a reservoir cascade. T Am Fish Soc. 137(6):1851–1865.
- Mitsch WJ, Reeder BC. 1991. Modelling nutrient retention of a freshwater coastal wetland: estimating the roles of primary productivity, sedimentation, resuspension and hydrology. Ecol Modell. 54(3–4):151–187.
- Mitsch WJ, Zhang L, Fink DF, Hernandez ME, Altor AE, Tuttle CL, Nahlik AM. 2008. Ecological engineering of floodplains. Ecohydrol Hydrobiol. 8(2–4):139–147.
- Mitsch WJ, Zhang L, Stefanik KC, Nahlik AM, Anderson CJ, Bernal B, Hernandez M, Song K. 2012. Creating wetlands: primary succession, water quality changes, and self-design over 15 years. Bioscience. 62(3):237–250.
- Mitsch WJ, Zhang L, Waletzko E, Bernal B. 2014. Validation of the ecosystem services of created wetlands: two decades of plant succession, nutrient retention, and carbon sequestration in experimental riverine marshes. Ecol Eng. 72:11–24.
- Mulder HM, Querner EP. 2008. Waterberging op het landgoed Lankheet: Mogelijkheden en consequenties voor het watersysteem [Water storage on the Lankheet estate: possibilities and consequences for the water system]. Wageningen (the Netherlands): Alterra Report 1674.
- Newbold JD, Elwood JW, O’Neill RV, Winkle WV. 1981. Measuring nutrient spiralling in streams. Can J Fish Aquat Sci. 38(7):860–863.
- Newcomer Johnson TA, Kaushal SS, Mayer PM, Smith RM, Sivirichi GM. 2016. Nutrient retention in restored streams and rivers: a global review and synthesis. Water. 8(4):116.
- Noe GB, Hupp CR. 2007. Seasonal variation in nutrient retention during inundation of a short-hydroperiod floodplain. River Res Appl. 23(10):1088–1101.
- Nürnberg GK. 1984. The prediction of internal phosphorus load in lakes with anoxic hypolimnia. Limnol Oceanogr. 29(1):111–124.
- Ottosen LDM, Risgaard-Petersen N, Nielsen LP. 1999. Direct and indirect measurements of nitrification and denitrification in the rhizosphere of aquatic macrophytes. Aquat Microb Ecol. 19(1):81–91.
- Özen A, Karapınar B, Kucuk I, Jeppesen E, Beklioglu M. 2010. Drought-induced changes in nutrient concentrations and retention in two shallow Mediterranean lakes subjected to different degrees of management. Hydrobiologia. 646(1):61–72.
- Paerl HW, Scott JT, McCarthy MJ, Newell SE, Gardner WS, Havens KE, Hoffman DK, Wilhelm SW, Wurtsbaugh WA. 2016. It takes two to tango: When and where dual nutrient (N and P) reductions are needed to protect lakes and downstream ecosystems. Environ Sci Technol. 50(20):10805–10813.
- Petticrew EL, Kalff J. 1992. Water flow and clay retention in submerged macrophyte beds. Can J Fish Aquat Sci. 49(12):2483–2489.
- Powers SM, Johnson RA, Stanley EH. 2012. Nutrient retention and the problem of hydrologic disconnection in streams and wetlands. Ecosystems. 15(3):435–449.
- Prairie YT. 1989. Statistical models for the estimation of net phosphorus sedimentation in lakes. Aquat Sci. 51(3):192–210.
- Raworth K. 2012. A safe and just space for humanity: can we live within the doughnut. Oxfam Policy and Practice: Climate Change and Resiliance. 8(1):1–26.
- Reddy K, D’angelo E. 1990. Biomass yield and nutrient removal by water hyacinth (Eichhornia crassipes) as influenced by harvesting frequency. Biomass. 21(1):27–42.
- Reddy KR, De Busk WF. 1985. Nutrient removal potential of selected aquatic macrophytes. J Environ Qual. 14(4):459–462.
- Risgaard-Petersen N, Jensen K. 1997. Nitrification and denitrification in the rhizosphere of the aquatic macrophyte Lobelia dortmanna L. Limnol Oceanogr. 42(3):529–537.
- Saunders DL, Kalff J. 2001. Nitrogen retention in wetlands, lakes and rivers. Hydrobiologia. 443(1–3):205–212.
- Scheffer M, Hosper SH, Meijer ML, Moss B, Jeppesen E. 1993. Alternative equilibria in shallow lakes. Trends Ecol Evol. 8(8):275–279.
- Schmadel NM, Harvey JW, Alexander RB, Schwarz GE, Moore RB, Eng K, Gomez-Velez JD, Boyer EW, Scott D. 2018. Thresholds of lake and reservoir connectivity in river networks control nitrogen removal. Nat Commun. 9(1):1–10.
- Schulz M, Kozerski H-P, Pluntke T, Rinke K. 2003. The influence of macrophytes on sedimentation and nutrient retention in the lower River Spree (Germany). Water Res. 37(3):569–578.
- Seitzinger SP, Styles RV, Boyer EW, Alexander RB, Billen G, Howarth RW, Mayer B, Van Breemen N. 2002. Nitrogen retention in rivers: model development and application to watersheds in the northeastern USA. In: Boyer EW, Howarth RW, editors. The nitrogen cycle at regional to global scales. New York (NY, USA): Springer; p. 199–237.
- Sigua GC. 2009. Recycling biosolids and lake-dredged materials to pasture-based animal agriculture: alternative nutrient sources for forage productivity and sustainability. A review. Agron Sustain Dev. 29(1):143–160.
- Smil V. 2000. Phosphorus in the environment: natural flows and human interferences. Ann Rev Energy Env. 25(1):53–88.
- Smolders AJP, Lamers LPM, Lucassen ECHET, Van der Velde G, Roelofs JGM. 2006. Internal eutrophication: How it works and what to do about it—a review. Chem Ecol. 22(2):93–111.
- Smolders AJP, Lucassen ECHET, Roelofs JGM. 2002. The isoetid environment: biogeochemistry and threats. Aquat Bot. 73(4):325–350.
- Sollie S, Coops H, Verhoeven JTA. 2008. Natural and constructed littoral zones as nutrient traps in eutrophicated shallow lakes. Hydrobiologia. 605(1):219–233.
- Søndergaard M. 1988. Seasonal variations in the loosely sorbed phosphorus fraction of the sediment of a shallow and hypereutrophic lake. Environ Geol Water Sci. 11(1):115–121.
- Søndergaard M, Jensen PJ, Jeppesen E. 2001. Retention and internal loading of phosphorus in shallow, eutrophic lakes. Sci World J. 1:427–442.
- Stanley EH, Doyle MW. 2002. A geomorphic perspective on nutrient retention following dam removal: geomorphic models provide a means of predicting ecosystem responses to dam removal. BioScience. 52(8):693–701.
- Strokal M, Kahil T, Wada Y, Albiac J, Bai Z, Ermolieva T, Langan S, Ma L, Oenema O, Wagner F. 2020. Cost-effective management of coastal eutrophication: a case study for the Yangtze River basin. Resour Conserv Recycl. 154:104635.
- Strokal M, Kroeze C, Wang M, Bai Z, Ma L. 2016. The marina model (model to assess river inputs of nutrients to seas): model description and results for China. Sci Total Environ. 562:869–888.
- Svendsen LM, Kronvang B. 1993. Retention of nitrogen and phosphorus in a Danish lowland river system: implications for the export from the watershed. In: Hillbricht-Ilkowska A, Pieczynska E, editors. Nutrient dynamics and retention in land/water ecotones of lowland, temperate lakes and rivers. New York (NY, USA): Springer; p. 123–135.
- Tang H, Xie P. 2000. Budgets and dynamics of nitrogen and phosphorus in a shallow, hypereutrophic lake in China. J Freshwater Ecol. 15(4):505–514.
- Tang T, Strokal M, van Vliet MTH, Seuntjens P, Burek P, Kroeze C, Langan S, Wada Y. 2019. Bridging global, basin and local-scale water quality modeling towards enhancing water quality management worldwide. Curr Opin Environ Sustain. 36:39–48.
- ter Veld D. 2014. Decoratief of effectief?: Natuurvriendelijke oevers: Thema [Decorative or effective? Nature-friendly banks: Theme]. H twee O: tijdschrift voor watervoorziening en afvalwaterbehandeling [H2O: a magazine for water supply and wastewater treatment]. 47(12):30–33.
- Teurlincx S, van Wijk D, Mooij WM, Kuiper JJ, Huttunen I, Brederveld RJ, Chang M, Janse JH, Woodward B, Hu F. 2019. A perspective on water quality in connected systems: modelling feedback between upstream and downstream transport and local ecological processes. Curr Opin Environ Sustain. 40:21–29.
- Tundisi J, Rocha O, Matsumura-Tundisi T, Braga B. 1998. Reservoir management in South America. Int J Water Resour Dev. 14(2):141–155.
- Tysmans DJJ, Löhr AJ, Kroeze C, Ivens WPMF, van Wijnen J. 2013. Spatial and temporal variability of nutrient retention in river basins: a global inventory. Ecol Indic. 34:607–615.
- Uhlmajnn D, Horn H. 1992. The significance of sedimentation and sediments to phytoplankton growth in drinking-water reservoirs. In: Sutcliffe DW, Jones JG, editors. Eutrophication: research and application to water supply. Ambleside (UK): Freshwater Biological Association; p. 94–106.
- United Nations 2015. Transforming our world: the 2030 agenda for sustainable development. UN: New York, NY, USA; p. 1–41.
- Van Cappellen P, Maavara T. 2016. Rivers in the Anthropocene: global scale modifications of riverine nutrient fluxes by damming. Ecohydrol Hydrobiol. 16(2):106–111.
- Van Dam AA, Dardona A, Kelderman P, Kansiime F. 2007. A simulation model for nitrogen retention in a papyrus wetland near lake Victoria, Uganda (East Africa). Wetl Ecol Manag. 15(6):469–480.
- Van Drecht G, Bouwman AF, Boyer EW, Green P, Siebert S. 2005. A comparison of global spatial distributions of nitrogen inputs for nonpoint sources and effects on river nitrogen export. Global Biogeochem Cy. 19(4). doi:https://doi.org/10.1029/2005GB002454
- Van Gerven LPA, Kuiper JJ, Janse JH, Janssen ABG, Jeuken M, Mooij WM, De Klein JJM. 2017. How regime shifts in connected aquatic ecosystems are affected by the typical downstream increase of water flow. Ecosystems. 20(4):733–744.
- Van Gerven LPA, Smit AAMFR, Groenendijk P, Van der Bolt FJE, De Klein JJM. 2009. Retentieschatting van N en P in het oppervlaktewater op verschillende schaalniveaus [Retention estimation of N and P in the surface water on different scales]. Wageningen (the Netherlands): Alterra Report 1848:1566–7197.
- Van Zuidam JP, Peeters ETHM. 2012. Cutting affects growth of Potamogeton lucens L. and Potamogeton compressus L. Aquat Bot. 100:51–55.
- Veraart AJ, de Bruijne WJJ, de Klein JJM, Peeters ETHM, Scheffer M. 2011. Effects of aquatic vegetation type on denitrification. Biogeochemistry. 104(1–3):267–274.
- Vila-Costa M, Pulido C, Chappuis E, Calvino A, Casamayor EO, Gacia E. 2016. Macrophyte landscape modulates lake ecosystem-level nitrogen losses through tightly coupled plant-microbe interactions. Limnol Oceanogr. 61(1):78–88.
- Vörösmarty CJ. 1997. The storage and aging of continental runoff in large reservoir systems of the world. Ambio. 26:210–219.
- Vörösmarty CJ, Meybeck M, Fekete B, Sharma K, Green P, Syvitski JPM. 2003. Anthropogenic sediment retention: major global impact from registered river impoundments. Global Planet Change. 39(1–2):169–190.
- Vroom RJE, Xie F, Geurts JJM, Chojnowska A, Smolders AJP, Lamers LPM, Fritz C. 2018. Typha latifolia paludiculture effectively improves water quality and reduces greenhouse gas emissions in rewetted peatlands. Ecol Eng. 124:88–98.
- Wang Y, Kong X, Peng Z, Zhang H, Liu G, Hu W, Zhou X. 2020. Retention of nitrogen and phosphorus in lake Chaohu, China: implications for eutrophication management. Environ Sci Pollut Res. 27:41488–41502.
- Wang Z, Zhang Z, Zhang Y, Zhang J, Yan S, Guo J. 2013. Nitrogen removal from lake Caohai, a typical ultra-eutrophic lake in China with large scale confined growth of Eichhornia crassipes. Chemosphere. 92(2):177–183.
- Weisner SEB, Eriksson PG, Granéli W, Leonardson L. 1994. Influence of macrophytes on nitrate. Ambio. 23(6):363–366.
- White JS, Bayley SE. 2001. Nutrient retention in a northern prairie marsh (Frank Lake, Alberta) receiving municipal and agro-industrial wastewater. Water Air Soil Pollut. 126(1–2):63–81.
- Wollheim WM, Vörösmarty CJ, Peterson BJ, Seitzinger SP, Hopkinson CS. 2006. Relationship between river size and nutrient removal. Geophys Res Lett. 33(6):L06410.
- Wu H, Zhang J, Ngo HH, Guo W, Hu Z, Liang S, Fan J, Liu H. 2015. A review on the sustainability of constructed wetlands for wastewater treatment: design and operation. Bioresour Technol. 175:594–601.
- Ye S, Covino TP, Sivapalan M, Basu NB, Li HY, Wang SW. 2012. Dissolved nutrient retention dynamics in river networks: a modeling investigation of transient flows and scale effects. Water Resour Res. 48(6):W00J17.
- Yin C, Zhao M, Jin W, Lan Z. 1993. A multi-pond system as a protective zone for the management of lakes in China. In: Hillbricht-Ilkowska A, Pieczynska E, editors. Nutrient dynamics and retention in land/water ecotones of lowland, temperate lakes and rivers. New York (NY, USA): Springer; p. 321–329.
- Yuan Z, Jiang S, Sheng H, Liu X, Hua H, Liu X, Zhang Y. 2018. Human perturbation of the global phosphorus cycle: changes and consequences. Environ Sci Technol. 52(5):2438–2450.
- Zhang T, Soranno PA, Cheruvelil KS, Kramer DB, Bremigan MT, Ligmann-Zielinska A. 2012. Evaluating the effects of upstream lakes and wetlands on lake phosphorus concentrations using a spatially-explicit model. Landsc Ecol. 27(7):1015–1030.
- Zhu M, Zhu G, Nurminen L, Wu T, Deng J, Zhang Y, Qin B, Ventelä A-M. 2015. The influence of macrophytes on sediment resuspension and the effect of associated nutrients in a shallow and large lake (Lake Taihu, China). PloS One. 10(6):e0127915.