ABSTRACT
Resilience research is gaining center stage at a time when fast social–ecological changes impose serious sustainability challenges to human societies and ecosystems. This paper conceptualizes resilience in temporary wetlands. A model is presented that demonstrates adaptive capacity, recovery, thresholds, regime shifts, and alternative regimes concepts, exemplified using wildland fires and their mitigation. The paper concludes with an examination of management challenges. Creating self-organizing temporary wetland landscapes similar to those existing prior to degradation may be elusive. Social–ecological tradeoffs may limit restoration and conservation.
Introduction
Temporary wetlands encompass a diverse range of habitats (e.g., vernal pools, wet meadows, seasonal ponds, and floodplains) that host unique flora and fauna (Williams Citation2012, Calhoun et al. Citation2017). In addition, they fulfill important functions in landscapes, contributing to regulating recreational, aesthetic, and provisioning ecosystem services (Gleason et al. Citation2008). Temporary wetlands also outnumber permanent lakes and streams and therefore typify the aquatic environment in many areas of the world (Van den Broeck et al. Citation2015), particularly Mediterranean-type and other dryland regions (Alvarez-Cobelas et al. Citation2005). However, temporary wetlands are seriously threatened by the compounded impacts of human activity (e.g., agriculture, land conversion, climate change; Barbosa et al. Citation2020) and are being lost at alarming rates (Zacharias and Zamparas Citation2010). Scientists and practitioners concur that sound management is required for restoration and conservation of these endangered ecosystems.
Resilience theory has gained center stage to address management challenges while accounting for the complexity that humans and ecosystems face on a fast-changing planet. However, development within the field of resilience has not been without problems. Increased normative use of concepts has resulted in loss of clarity (Brand and Jax Citation2007); consequently, resilience scholarship has frequently inspired opaque discourse, and competing frameworks are often inconsistent with the complexity inherent in (eco)systems (Allen et al. Citation2019). It is crucial to account for the multiple meanings of resilience in temporary wetland ecology, a prerequisite for confronting sustainability and management challenges.
Here a unified view of resilience is adopted that reconciles resilience as an emergent phenomenon of complex adaptive systems, which is inherent in ecological resilience (Holling Citation1973) and adaptive capacity (Gunderson Citation2000), with stability aspects (e.g., recovery, robustness) central to ecological stability theory (Allen et al. Citation2019; Appendix 1). These facets are discussed and resilience concepts are described, first generally and then applied to temporary wetlands. A conceptual model of resilience of temporary wetlands is illustrated with a case study, followed by management implications for these ecosystems.
Resilience concepts
Two camps of resilience have been crystalizing in ecosystem science in recent decades, one rooted in ecological stability and the other branching out of complex adaptive systems science. Both emphasize different levels of complexity and are not mutually exclusive (Allen et al. Citation2019). Ecological stability focuses resilience on the time it takes for a system to recover from a disturbance (“recovery,” e.g., Pimm Citation1991; a). This process rate is often portrayed as a rolling ball within a basin of attraction symbolized with the “ball-in-cup” heuristic (e.g., Gunderson Citation2000; ). Resilience in stability research is synonymous with engineering resilience, resiliency, bounce-back, and recovery (Angeler and Allen Citation2016).
Figure 1. Schematics contrasting (a) engineering resilience (recovery) with (b) ecological resilience. Engineering resilience is a process rate within a single basin of attraction. Ecological resilience invokes alternative regimes and threshold responses leading to emergent phenomena.
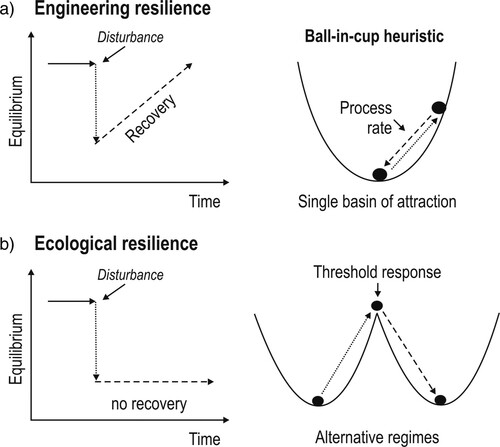
By contrast, ecological resilience (sensu Holling Citation1973) accounts for the existence of thresholds and the possibility of ecosystems to shift between and exist in alternative regimes. Rather than a process rate, ecological resilience accounts for multiple basins of attractions and emergent phenomena (Gunderson Citation2000; b). Emergent phenomena arise from stabilizing feedbacks that are more than the sum of all biophysical structures and linkages present in the system. Consider the example of a shallow lake: it can exist in a clear-water, macrophyte-dominated regime and shift to a turbid, plankton-dominated regime upon excessive nutrient enrichment (Scheffer et al. Citation1993). The turbid regime is stable, and the lake would not return to its previous condition without significant management. More generally, even with substantial management, but contrary to the notion of recovery in ecological stability theory, restoration to a previous system regime is not guaranteed, as documented by lake restoration research (e.g., Hansson et al. Citation1998).
Inherent in ecological resilience is the ability of ecosystems to absorb and cope with disturbances, a phenomenon referred to as adaptive capacity, which has been defined differently across the social and ecological sciences (Angeler et al. Citation2019). Adaptive capacity, which arises from cross-scale interactions (hierarchical interplay) of a plethora of factors (), is inclusive of structural and functional patterns and processes, different aspects of ecological stability (e.g., robustness, recovery), and ecological memory (Angeler et al. Citation2019). A system with high adaptive capacity is able to mold the basin of attraction in relation to disturbances in a way to “keep the ball inside the cup” (a). Conversely, a system with low adaptive capacity can be portrayed with a ball rolling from a shallow, rigid cup into another cup (alternative regime) when a disturbance threshold has been exceeded (b).
Figure 2. Schematics of (a) high and (b) low adaptive capacity. Shown are temporal snapshots of changing basins of attraction over time (sequence of gray arrows) in response to disturbances. High adaptive capacity facilitates engineering resilience; low adaptive capacity is conducive to regime shifts.
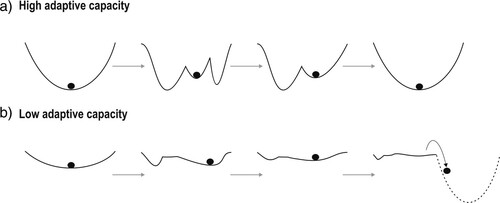
Table 1. Factors contributing to adaptive capacity within and across levels of biological organization. This table is meant to show the complexity of factors mediating adaptive capacity and is therefore not exhaustive. Modified from Angeler et al. (Citation2019).
Adaptive capacity and ecological resilience comprise different sides of the same coin (). From a system perspective, adaptive capacity is the latent potential structured by disturbances, whereas ecological resilience is the realization and expression of that potential (Angeler et al. Citation2019).
A conceptual model for temporary wetlands is presented that builds on this notion () and accounts for the recurring wet–dry phases that dictate the dynamics of temporary wetlands. The model indicates that adaptive capacity in temporary wetlands can be temporarily uncoupled from ecological resilience.
Figure 3. Conceptual model of adaptive capacity and ecological resilience in temporary wetlands emphasizing alternative regimes (white and gray basins of attraction) in which dry and wet phases alternate as part of the natural disturbance regime. Note that the association made with seasons is an overgeneralization made to support the case study (see text). The model associates the latent potential of adaptive capacity and the expression of this potential in the form of ecological resilience in the dry and wet phases, respectively. The model also emphasizes disturbances during dry and wet periods that can lead to different impact–ecological response patterns (Path 1, Path 2). Note: these impact–response patterns have been showcased for one regime in the model for simplicity, but they apply similarly for both regimes.
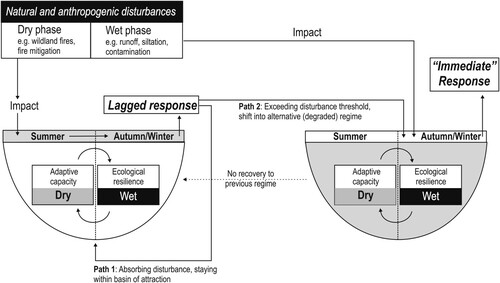
Resilience and adaptive capacity in temporary wetlands
The conceptual model () builds on a simplified representation of alternating dry and wet phase, a systemic characteristic of temporary habitats (e.g., Gasith and Resh Citation1999). These dynamics are inherent in the disturbance regimes of temporary wetlands rather than wet and dry phases comprising alternative system regimes per se. However, climate change with increased magnitudes, frequencies, and durations of extreme wet and dry spells (e.g., Heinrich and Gobiet Citation2012) can cause nonlinear transitions in wetland systems (Ireland et al. Citation2012), triggering regime changes in biological communities and hydrological functioning (e.g., Loverde-Oliveira et al. Citation2009). Thus, nonlinear abiotic and biotic changes triggered by extreme climate events can shift ecosystems between alternative humid and drought regimes on interannual time scales. These regime shifts must be discerned from the seasonally predictable and recurring wet–dry phases envisioned in the model.
The model associates dry phases with the latent potential of adaptive capacity and wet phases with the manifestation of ecological resilience. Temporary wetlands endure dry periods in the form of resting eggs, seeds, and other propagules banked in their dry soils (Bonis and Grillas Citation2002, Brendonck and De Meester Citation2003), forming part of a latent potential of biodiversity that manifests when ponds rewet (Brock et al. Citation2003). Recolonization from seed banks has been considered a form of dispersal in time (Mergeay et al. Citation2007), a useful view showing that the manifestation of ecological responses to natural and anthropogenic disturbances can be lagged (). Such delayed responses may be obvious, for instance when impact occurs during dry summer months, but the consequences of disturbances manifest upon rewetting in autumn and winter (“lagged response” in ). Associating dry and wet periods with seasons is a rough generalization because patterns of hydroperiods and flood frequencies can be more subtle and highly variable within and between temporary environments (Mitsch and Gosselink Citation2007). Although not universally applicable, this overgeneralization was chosen to showcase the model with the case study described later.
Although alternative regime models may not unambiguously characterize wetland ecology (e.g., Sim et al. Citation2006b), increasing evidence indicates that temporary wetlands can exist, like shallow lakes, in alternative system configurations (e.g., Hagerthey et al. Citation2008). More specifically, they can occur in either a clear-water regime dominated by submerged macrophytes or a turbid, phytoplankton-dominated regime (see case study discussed later).
The implication of delayed ecological responses is that ponds need to rewet to assess ecological resilience after dry periods. Specifically, the model shows one path in which disturbance(s) do not exhaust the adaptive capacity of the wetland. Consequently, ecological resilience manifests as the pond staying in its basin of attraction upon rewetting (path 1 in , left basin of attraction). By contrast, if disturbances significantly impact the latent biodiversity potential and other biophysical characteristics, adaptive capacity might be exhausted. This erosion of adaptive capacity leads to a path for ponds to shift to, reorganize, and stabilize in an alternative regime during flooding (path 2 in , right basin of attraction). Such a shift indicates that novel regimes are self-organizing and self-maintaining: adaptive capacity and ecological resilience attain new configurations, preventing the system from returning to previous configurations ().
Impact on wetlands can be direct or indirect and alter communities through changes in water quality and propagule bank potential (Angeler and García Citation2005) or ecological connectivity and dispersal between sites (Uden et al. Citation2014). The model thus simplifies complexity while recognizing the seasonality of impact of local and regional disturbances on temporary wetlands. The model also implicitly acknowledges that impact may occur during wet periods when local- and catchment-scale processes act in concert with hydrological and meteorological factors. Such disturbances include cattle grazing and trampling (Van den Broeck et al. Citation2019), nutrient and pollution runoff (Gathumbi et al. Citation2005), sediment accrual (Larson et al. Citation2020), salinization (Waterkeyn et al. Citation2010), and drainage (McCauley et al. Citation2015). In such wet-phase disturbance situations, adaptive capacity is not temporarily uncoupled from ecological resilience, thereby reflecting the situation of permanent lakes. Notwithstanding, resilience paths (recovery vs. regime shifts) in this “wet–impact–wet” response scenario may be similar to those envisioned for dry-phase impact and wet-period response (). In a “wet–wet” case, the plasticity of amphibian breeding behavior in a changing climate exemplifies recovery (Lowe et al. Citation2015). Conversely, salinizing wetlands affected by agriculture in Australia are instances of regime shifts (Sim et al. Citation2006a).
Case study
To demonstrate the model, a case study was chosen that fits lagged ecological disturbance–response patterns. Although the example focuses on the outcomes of wildland fires and their management, it is representative of eutrophication issues, a worldwide problem resulting in biodiversity and ecosystem service loss.
Wildland fires and fire mitigation
Summer droughts produce an annual fire hazard that contributes to a highly predictable fire regime (e.g., Keeley Citation2012). Wildland fires are an integral part of the natural disturbance regime in Mediterranean climates to which ecological systems have evolutionarily adapted (Keeley et al. Citation2011). Because of the coevolution between fire and ecosystems, Cunillera-Montcusí et al. (Citation2019) hypothesized that wildland fire impacts on temporary ponds will be direct and indirect but short-lived. In response to an intense wildfire during summer 2012 that burned 13 000 ha and exposed part of a temporary pond network to low and high fire intensities, they studied environmental and faunal responses in burned and unburned ponds using a before-after-control-impact design. The authors found different wildfire impacts, including indirect effects on water quality and phytoplankton and direct effects (decreased abundance) on invertebrates that remained in the pond sediment during drought in burned ponds. The authors concluded that one hydroperiod was enough for burned ponds to recover their ecological similarity to unburned ponds, highlighting, at least in their study, the high recovery potential of these ecosystems to the fire regimes present in their systems. Their finding follows expectations that temporary ponds may maintain high adaptive capacity in relation to wildland fires and fits the impact–recovery path 1 in the model (). Future research comprising different fire regimes and biophysical pond network settings will provide insight into the generality of such recovery patterns.
Fire management increasingly uses fire retardant chemicals to prevent and control fires. However, fire retardants are composed of ammonium sulfates and/or phosphates that cause substantial environmental impact (Marshall et al. Citation2016). Unfortunately, environmental factors (smoke development during fires, decreased visibility, winds, visual recognition of dry ponds) can complicate management and lead to accidental contamination and eutrophication of temporary wetlands. Fire retardant impacts in temporary ponds have been confirmed in field and laboratory experiments, although further study is needed to assess the generality of contamination severity and ecological responses of the studies presented.
Angeler and Moreno (Citation2006) assessed impact using a before-after-control-impact experimental design in artificial outdoor ponds. Simulating fire management in grasslands and woodlands, they found a substantial deterioration of water quality, more pronounced in the woodland treatment than in the grassland treatment. The ponds were experimentally contaminated with Fire-Trol 934 (Biogema, Cedex, France) when they were dry. Several months later, when they were reflooded, total phosphorus rose to concentrations unmatched in hypertrophic aquatic ecosystems and exceeded by far those in municipal wastewaters. Although trophic state conditions improved during the after-period, recovery was partial and stalled in both treatments during the second hydroperiod.
In a subsequent study, Angeler and Moreno (Citation2007) studied fire retardant impact on zooplankton community structure in the same ponds. The retardant caused a decline in species richness and an increase in rotifer abundances during summer, late autumn, and winter relative to controls and pretreatment dates. The duration of these changes varied across retardant treatments, and rotifer abundances peaked unpredictably over time. These temporal patterns suggested stochastic oscillations in community composition, punctuated by periods in which the community approached reference conditions. These findings imply that the detection of perturbation will require intensive sampling to devise suitable management and conservation strategies.
Overall, these results suggested that the experimental ponds likely underwent a fundamental reorganization as a result of fire-retardant contamination consistent with path 2 in the model (). If such profound changes can be further confirmed in future studies, a single fire-retardant pollution event, and potentially other anthropogenic disturbances, may be enough to irreversibly shift temporary wetlands into degraded regimes. In these regimes, natural and anthropogenic stress can lead to complex synergistic and antagonistic ecological interactions that further complicate management (Martín et al. Citation2014).
Synthesis and management outlook
The model presented in this study is at the preliminary and conceptual stage and informed by preliminary scientific evidence. However, given the pressing need to manage temporary wetlands for resilience, the model can inspire future empirical research. Methods can apply available quantitative approaches rooted in resilience theory (Angeler et al. Citation2016), and approaches can build on adaptive management, sampling, inference, and modeling to reduce uncertainty (Baho et al. Citation2017). Furthermore, the model can be used in synergy with heuristics from resilience that focus on cross-scale interactions and dynamic systems change (e.g., Panarchy; Allen et al. Citation2014). These studies can be tailored to different spatiotemporal and environmental contexts (local to regional) to derive the broadest possible insight in the complex organization of temporary wetland networks and their management (Garmestani et al. Citation2020). Adaptive approaches may eventually be insufficient for ecosystem management (i.e., when regime shifts are unavoidable), and managers may need to prepare for transformative change and engage in scenario planning to envision potentially different novel ecosystems with changed conditions for ecosystem service provisioning (Chaffin et al. Citation2016).
Despite the usefulness of the model, several issues related to ecological complexity must be considered to envision the full range of potential and limitations for future model development and testing. One aspect deserving consideration is the non-stationarity of nature, demonstrated in the past rise and fall of human cultures and historical extinctions of species. Non-stationary change entails fluctuations within changing envelopes of variability (Milly et al. Citation2008). Constant change of ecosystem baselines, rather than stasis, is an undeniable reality (Craig Citation2010). The practical implication is that returning to past conditions can be doomed to fail, more so in a fast-changing world where social, ecological, technological, and economic change occurs at accelerated speeds (Castree Citation2019).
Unfortunately, the non-stationary reality of nature has not melded well with traditional wetland science and management, which are often rooted in stationary thinking, as are the underlying regulating environmental laws (Garmestani et al. Citation2019). Specifically, regulations, such as the EU Water Framework Directive, from which temporary waters are largely exempt (Van den Broeck et al. Citation2015), require the achievement of “good” ecological status, invoking the attainment of desired reference conditions through either adaptive management or restoration. However, because such attainment can be elusive under non-stationary change (Heino et al. Citation2021), the paper concludes with scrutinizing management challenges of temporary wetlands. Temporary wetlands can be viewed as “coerced regimes” (Angeler et al. Citation2020), a view that builds on the need to consider both reactive and proactive approaches required for resilience-based management (Chaffin et al. Citation2016, Baho et al. Citation2017).
Temporary wetlands as coerced regimes
Coerced regimes are based on the notion that biophysical conditions in self-organizing ecosystems can no longer be maintained as a result of social–ecological change and that intensive management is needed to artificially maintain system conditions. The coercive nature of ecosystem conservation is evident in management that only simulates a self-sustaining ecosystem because such a regime has become obsolete as a result of novel conditions. Management focuses on metaphorical “ghosts” of past system regimes desired for humanity in terms of ecosystem service provisioning and biodiversity conservation. The broad relevance of coerced regimes has been exemplified with mental disorders in human subjects, terrestrial and aquatic ecosystems, and the global climate (Angeler et al. Citation2020).
Temporary wetlands and their networks potentially qualify as an additional example of coerced regimes. These ecosystems are islands embedded in—and biologically, chemically, physically, and hydrologically connected to—their surrounding terrestrial matrix. These linkages clearly emphasize that temporary wetlands are integral parts nested within a broader landscape (e.g., Calhoun et al. Citation2017). Alterations in land use, including the increasing conversion of natural habitats to agricultural lands, contribute to the disappearance of temporary wetlands and the ecological deterioration of those that remain (Zacharias and Zamparas Citation2010). This degradation, combined with agriculture’s competing demands for often limited water resources to feed an increasing human population, poses a further risk to temporary waters (Schyns et al. Citation2019). Therefore, from a resilience perspective, wetland waterscapes are either bound for irreversible change or may have already undergone fundamental change. The metaphor “dead regimes walking” has been coined to emphasize the magnitude and severity of these threats to ecosystems (Angeler et al. Citation2020). This term also highlights that wetland management, rather than full restoration, is frequently limited to mitigation through management of ghost regimes.
Landscape-scale management is often constrained by and designed to protect political and economic interests (Twidwell et al. Citation2018), constraints that often limit management to local habitat and wildlife conservation (e.g., fences). However, these conservation practices provide a striking example that successful management is problematic. A common fallacy stems from management assumptions about social–ecological systems being stationary. The “field of dreams” myth (Hilderbrand et al. Citation2005) exemplifies that assuming habitat restoration and species conservation go hand in hand is illusory. Local and regional change can ultimately lead to the dying out of species (Tilman et al. Citation1994), meaning that local habitat protection in profoundly altered and fragmented landscapes will not guarantee the survival of endangered species.
Temporary wetlands embody non-stationary landscapes unsuited for management policies that focus on habitat restoration in static landscapes. Changing magnitudes and rates of climate change are likely to amplify non-stationary change (Steffen et al. Citation2018), further worsening this problem. Temporary wetland complexes will need an ever-increasing amount of costly management to maintain ecological structures, functions, and ecosystem services, but whether recommended approaches to temporary wetlands management (education, inventory, protection, sustainable management, restoration; e.g., Calhoun et al. Citation2017) will be effective and sufficient in the long term is uncertain. Significant challenges remain for understanding temporary wetland responses to environmental change and how resilience-based approaches may contribute to the sound management of these unique ecosystems. Once fundamental change has occurred, reinstating past self-organizing temporary wetland landscapes may only be possible in an allegorical field of dreams.
Acknowledgements
This study was supported by Universidad Internacional de Andalucía through the workshop “The future of temporary wetlands in drylands under projected global change scenarios,” held 8–11 March 2020 in Baeza, Spain. The constructive comments of 2 anonymous reviewers helped to improve the paper.
Disclosure statement
No potential conflict of interest was reported by the author(s).
References
- Allen CR, Angeler DG, Garmestani A, Gunderson LH, Holling CS. 2014. Panarchy: theory and application. Ecosystems. 17:578–589.
- Allen CR, Angeler DG, Chaffin B, Twidwell D, Garmestani A. 2019. Resilience reconciled. Nat Sustain. 2:898–900.
- Alvarez-Cobelas M, Rojo C, Angeler DG. 2005. Mediterranean limnology: current state, gaps and the future. J Limnol. 64(1):13–29.
- Angeler DG, Allen CR. 2016. Quantifying resilience. J Appl Ecol. 53(3):617–624.
- Angeler DG, Allen CR, Barichievy C, Eason T, Garmestani AS, Graham NAJ, Granholm D, Gunderson L, Knutson M, Nash KL, et al. 2016. Management applications of discontinuity theory. J Appl Ecol. 53:688–698.
- Angeler DG, Chaffin B, Sundstrom S, Garmestani A, Pope K, Uden D, Twidwell D, Allen CR. 2020. Coerced regimes: management challenges in the Anthropocene. Ecol Soc. 25(1):4.
- Angeler DG, Fried-Petersen HB, Allen CR, Garmestani A, Twidwell D, Chuang W, Donovan VM, Eason T, Roberts CP, Sundstrom SM, Wonkka CL. 2019. Adaptive capacity in ecosystems. Adv Ecol Res. 60:1–24.
- Angeler DG, García G. 2005. Using emergence from soil propagule banks as indicators of ecological integrity in wetlands: advantages and limitations. J N Am Benthol Soc. 24(4):740–752.
- Angeler DG, Moreno JM. 2006. Impact-recovery patterns of water quality in temporary wetlands after fire retardant pollution. Can J Fish Aquat Sci. 63(7):1617–1626.
- Angeler DG, Moreno JM. 2007. Zooplankton community resilience after press-type anthropogenic stress in temporary ponds. Ecol Appl. 17(4):1105–1115.
- Baho DL, Allen CR, Garmestani AS, Fried-Petersen HB, Renes SE, Gunderson L, Angeler DG. 2017. A quantitative framework for assessing ecological resilience. Ecol Soc. 22(3):17.
- Barbosa LG, Amorim CA, Parra G, Laço Portinho J, Morais M, Morales EA, Menezes RF. 2020. Advances in limnological research in earth’s drylands. Inland Waters. 10(4):429–437.
- Bonis A, Grillas P. 2002. Deposition, germination and spatio-temporal patterns of charophyte propagule banks: a review. Aquat Bot. 72(3–4):235–248.
- Brand FS, Jax K. 2007. Focusing the meaning(s) of resilience: resilience as a descriptive concept and a boundary object. Ecol Soc. 12(1):23.
- Brendonck L, De Meester L. 2003. Egg banks in freshwater zooplankton: evolutionary and ecological archives in the sediment. Hydrobiologia. 491:65–84.
- Brock MA, Nielsen DL, Shiel RJ, Green JD, Langley JD. 2003. Drought and aquatic community resilience: the role of eggs and seeds in sediments of temporary wetlands. Freshwater Biol. 48(7):1207–1218.
- Calhoun AJ, Mushet DM, Bell KP, Boix D, Fitzsimons JA, Isselin-Nondedeu F. 2017. Temporary wetlands: challenges and solutions to conserving a ‘disappearing’ ecosystem. Biol Cons. 211:3–11.
- Castree N. 2019. The ‘Anthropocene’ in global change science: expertise, the earth, and the future of humanity. In: Biermann F, Lövbrand E, editors. Anthropocene encounters: new directions in green political thinking. Cambridge (UK): Cambridge University Press; p. 25–49.
- Chaffin BC, Garmestani AS, Gunderson L, Harm Benson M, Angeler DG, Arnold CA, Cosens, Craig RK, Ruhl JB, Allen CR. 2016. Transformative environmental governance. Annu Rev Resour Environ. 41:399–423.
- Craig RK. 2010. ‘Stationarity is dead’ – Long live transformation: five principles for climate change adaptation law. Harvard Environ Law Rev 34:9.
- Cunillera-Montcusí D, Gascón S, Tornero I, Sala J, Àvila N, Quintana XD, Boix D. 2019. Direct and indirect impacts of wildfire on faunal communities of Mediterranean temporary ponds. Freshwater Biol. 64:323–334.
- Garmestani A, Ruhl JB, Chaffin BC, Craig RK, van Rijkswick HFMW, Angeler DG, Folke C, Gunderson L, Twidwell D, Allen CR. 2019. Untapped capacity for resilience in environmental law. P Natl Acad Sci. 116(40):19899–19904.
- Garmestani A, Twidwell D, Angeler DG, Sundstrom SM, Barichievy C, Chaffin B, Eason T, Graham N, Granholm D, Gunderson L, et al. 2020. Panarchy: opportunities and challenges for ecosystem management. Front Ecol Environ. 18:576–583.
- Gasith A, Resh VH. 1999. Streams in Mediterranean climate regions: abiotic influences and biotic responses to predictable seasonal events. Annu Rev Ecol Evol Syst. 30:51–81.
- Gathumbi SM, Bohlen PJ, Graetz DA. 2005. Nutrient enrichment of wetland vegetation and sediments in subtropical pastures. Soil Sci Soc Am J. 69(2):539–548.
- Gleason RA, Laubhan MK, Euliss NH, eds. 2008. Ecosystem services derived from wetland conservation practices in the United States Prairie Pothole Region with an emphasis on the U.S. Department of Agriculture Conservation Reserve and Wetlands Reserve Programs. US Geological Professional Paper 1745; p. 58.
- Gunderson LH. 2000. Ecological resilience – in theory and application. Annu Rev Ecol Syst. 31:425–439.
- Hagerthey SE, Newman S, Rutchey K, Smith EP, Godin J. 2008. Multiple regime shifts in a subtropical peatland: community-specific thresholds to eutrophication. Ecol Monogr. 78(4):547–565.
- Hansson LA, Annadotter H, Bergman E, Hamrin SF, Jeppesen E, Kairesalo T, Luokkanen E, Nilsson PÅ, Søndergaard M, Strand J. 1998. Biomanipulation as an application of food-chain theory: constraints, synthesis, and recommendations for temperate lakes. Ecosystems. 1(6):558–574.
- Heino J, Alahuhta J, Bini LM, Cai Y, Heiskanen A-S, Hellsten S, Kortelainen P, Kotamäki N, Tolonen KT, Vihervaara P, et al. 2021. Lakes in the era of global change: moving beyond single-lake thinking in maintaining biodiversity and ecosystem services. Biol Rev. 96:89–106.
- Heinrich G, Gobiet A. 2012. The future of dry and wet spells in Europe: a comprehensive study based on the ENSEMBLES regional climate models. Int J Climatol. 32:1951–1970.
- Hilderbrand RH, Watts AC, Randle AM. 2005. The myths of restoration ecology. Ecol Soc. 10(1):19.
- Holling CS. 1973. Resilience and stability of ecological systems. Annu Rev Ecol Syst. 4:1–23.
- Ireland AW, Booth RK, Hotchkiss SC, Schmitz JE. 2012. Drought as a trigger for rapid state shifts in kettle ecosystems: implications for ecosystem responses to climate change. Wetlands. 32:989–1000.
- Keeley JE. 2012. Fire in Mediterranean climate ecosystems – a comparative overview. Isr J Ecol Evol. 58(2–3):123–135.
- Keeley JE, Bond WJ, Bradstock RA, Pausas JG, Rundel PW. 2011. Fire in Mediterranean ecosystems: ecology, evolution and management. Cambridge (UK): Cambridge University Press.
- Larson DM, Riens J, Myerchin S, Papon S, Knutson MG, Vacek SC, Winikoff SG, Phillips ML, Giudice JH. 2020. Sediment excavation as a wetland restoration technique had early effects on the developing vegetation community. Wetl Ecol Manag. 28:1–18.
- Loverde-Oliveira SM, Huszar VLM, Mazzeo N, Scheffer M. 2009. Hydrology-driven regime shifts in a shallow tropical lake. Ecosystems. 12:807–819.
- Lowe K, Castley JG, Hero JM. 2015. Resilience to climate change: complex relationships among wetland hydroperiod, larval amphibians and aquatic predators in temporary wetlands. Mar Freshwater Res. 66(10):886–899.
- Marshall A, Waller L, Lekberg Y. 2016. Cascading effects of fire retardant on plant–microbe interactions, community composition, and invasion. Ecol Appl. 26:996–1002.
- Martín S, Rodríguez M, Moreno JM, Angeler DG. 2014. Complex ecological responses to drought and fire-retardant contamination impacts in ephemeral waters. Water Air Soil Pollut. 225:2078.
- McCauley LA, Anteau MJ, van der Burg MP, Wiltermuth MT. 2015. Land use and wetland drainage affect water levels and dynamics of remaining wetlands. Ecosphere. 6(6):1–22.
- Mergeay J, Vanoverbeke J, Verschuren D, De Meester L. 2007. Extinction, recolonization, and dispersal through time in a planktonic crustacean. Ecology. 88(12):3032–3043.
- Milly PC, Betancourt J, Falkenmark M, Hirsch RM, Kundzewicz ZW, Lettenmaier DP, Stouffer RJ. 2008. Stationarity is dead: whither water management? Science. 319:573–574.
- Mitsch WJ, Gosselink JG. 2007. Wetlands. 4th ed. Hoboken, NJ: John Wiley & Sons, Inc.
- Pimm SL. 1991. The balance of nature? Chicago (IL, USA): University of Chicago Press.
- Scheffer M, Hosper SH, Meijer M-L, Moss B, Jeppesen E. 1993. Alternative equilibria in shallow lakes. Trends Ecol Evol. 8:275–279.
- Schyns JF, Hoekstra AY, Booij MJ, Hogeboom RJ, Mekonnen MM. 2019. Limits to the world’s green water resources for food, feed, fiber, timber, and bioenergy. P Natl Acad Sci. 116:4893–4898.
- Sim LL, Chambers JM, Davis JA. 2006a. Ecological regime shifts in salinised wetland systems. I. Salinity thresholds for the loss of submerged macrophytes. Hydrobiologia. 573:89–107.
- Sim LL, Davis JA, Chambers JM, Strehlow K. 2006b. What evidence exists for alternative ecological regimes in salinising wetlands? Freshwater Biol. 51:1229–1248.
- Steffen W, Rockström J, Richardson K, Lenton TM, Folke C, Liverman D, Summerhayes CP, Barnosky AD, Cornell SE, Crucifix M, et al. 2018. Trajectories of the earth system in the Anthropocene. P Natl Acad Sci. 115:8252–8259.
- Tilman D, May RM, Lehman CL, Nowak MA. 1994. Habitat destruction and the extinction debt. Nature. 371(6492):65.
- Twidwell D, Wonkka C, Bielski C, Allen C, Angeler DG, Drozda J, Garmestani A, Johnson J, Powell L, Roberts C. 2018. The perpetual state of emergency that sacrifices protected areas in a changing climate. Cons Biol. 32:905–915.
- Uden DR, Hellman ML, Angeler DG, Allen CR. 2014. The role of reserves and anthropogenic habitats for functional connectivity and resilience of ephemeral wetlands. Ecol Appl. 24:1569–1582.
- Van den Broeck M, Rhazi L, Waterkeyn A, El Madihi M, Grillas P, Kneitel JM, Brendonck L. 2019. Livestock disturbances in Mediterranean temporary ponds: a mesocosm experiment with sheep manure and simulated trampling. Freshwater Biol. 64:856–869.
- Van den Broeck M, Waterkeyn A, Rhazi L, Grillas P, Brendonck L. 2015. Assessing the ecological integrity of endorheic wetlands, with focus on Mediterranean temporary ponds. Ecol Ind. 54:1–11.
- Waterkeyn A, Vanschoenwinkel B, Grillas P, Brendonck L. 2010. Effect of salinity on seasonal community patterns of Mediterranean temporary wetland crustaceans: a mesocosm study. Limnol Oceanogr. 55(4):1712–1722.
- Williams DD. 2012. The ecology of temporary waters. Berlin/Heidelberg (Germany): Springer Science & Business Media.
- Zacharias I, Zamparas M. 2010. Mediterranean temporary ponds: a disappearing ecosystem. Biodivers Cons. 19(14):3827–3834.
1
Definitions
Ordered after first mention in the text (adapted from Angeler et al. Citation2019)
Emergent phenomenon: Complex (eco)systems often have properties produced by the interactions of properties of their individual components (e.g., species). These emergent properties are unpredictable or unknowable from observation of the parts and more than the sum of these parts.
Complex adaptive system: Hierarchically structured systems that consist of diverse and autonomous parts or components (“agents”), which depend on and relate with each other and are linked through many connections. Examples: ecosystems, social networks, climate, markets, and the brain.
Ecological resilience: A measure of the amount of change needed to shift an ecosystem from one set of processes and structures to a different set of processes and structures.
Recovery: Return time (i.e., a process rate) to equilibrium after disturbances.
Robustness: Structural and functional system components remain unchanged after disturbances.
Ecological stability: System components remain within a particular system regime (basin of attraction) after disturbances. These components include variability, resistance, recovery, robustness, and persistence.
Basin of attraction (stability domain): A region of the state space where the system tends to remain and has a definable configuration in terms of the abundance of components, composition, and processes of a system.
Alternative regime or state: A potential alternate configuration in terms of the structural and functional composition, processes, and feedbacks of a system.
Engineering resilience: Synonym of recovery.
Resiliency: Synonym of recovery.
Bounce-back: Synonym of recovery.
Threshold: A point where a small change in external conditions causes a rapid change in an ecosystem. When an ecological threshold has been passed, an ecosystem may no longer be able to return to its previous regime.
Adaptive capacity: Latent potential of an ecosystem to respond to disturbances in a way that maintains the system within its current basin of attraction by molding the depth and/or width of that basin.
Feedback: Circuits in complex adaptive systems reinvest some of the yield to the input of a system to allow self-correction and adjustment to internal and external variables. The 2 categories of feedback loops are as follows. (1) Balancing (or negative) feedback loops occur when a disturbance is diminished over time. For instance, when predator numbers increase, the consumption of their prey also rises, resulting in a decrease in their population numbers. Because of the increasing shortage of prey, the number of predators decreases because of limited food availability. (2) Reinforcing (or positive) feedback loops occur when an initial condition promotes its change in the future. For instance, the number of offspring increases with population size of a species. Turning into adults, further offspring will be produced resulting in rapid population growth. Reinforcement of species growth rates can, in the absence of resource limitation and predator control, eventually have a significant impact on natural resources.
Ecological memory: The collective representation of functional and structural attributes in an ecosystem that has been shaped by the systems’ disturbance history.
Non-stationarity: Fluctuations within changing envelopes of system variability. For example, changing ecological baselines make historical references obsolete. Stationary change implies static envelopes of variability.