ABSTRACT
Approximately 120 years ago the link between mosquito and the malaria transmission was discovered. However, even today it remains an open question whether the parasite is able to direct the blood-seeking and feeding behavior of its mosquito vector to maximize the probability of transmission. If the parasite has this ability, could it occur only through the alteration of the vertebrate host’s volatile organic compounds (VOCs) and/or the parasite alteration of the behavior of the infected vector in a manner that favors its transmission? Although some recent empirical evidence supports the hypothesis regarding the parasite ability in alteration of the vertebrate host’s VOCs, the role of parasite alteration and behavioral differences between infected and uninfected female mosquitoes toward infected and uninfected hosts has not yet been considered in the implementation of control measures. This review will discuss the current evidence, which shows 1. Plasmodium can direct uninfected mosquito blood-seeking and feeding behavior via alteration of vertebrate-host odor profiles and production of phagostimulants and 2. Plasmodium also manipulates its vector during the sporogony cycle to increase transmission. Briefly, we also consider the next generation of methods for moving the empirical laboratory evidence to potential application in future integrated malaria control programs.
Introduction
A key requirement for transmission of malaria parasites is the uptake of an infected blood meal by a mosquito which initiates the parasite transmission cycle (). The rate of transmission, as shown by Ross-MacDonald equation, is exponentially related to the human biting rate, which is the proportion of the mosquitoes that feed on humans each day [Citation1,Citation2]. The host seeking and biting rate of Anopheles mosquitoes on an individual human host are suggested to be correlated with host skin microbial composition and, recent work, also identified malaria parasite-associated cues [Citation3,Citation4].
Figure 1. Plasmodium falciparum transmission cycle.
The human malaria transmission cycle, including the first bite of an uninfected mosquito (top left) on a gametocyte carrier (infective human: schizogony cycle). Mature gametocytes are taken up by female mosquitoes, initiating the mosquito developmental stages of the parasites (sporogony cycle), and during this stage the parasite follows replication stages and the mosquito becomes infected during ~7–10 days and infective ~11–14 days. When an infective mosquito bites an uninfected human host (top right), it can transmit the infectious stage (sporozoites) to a second human host and malaria transmission cycle continues.
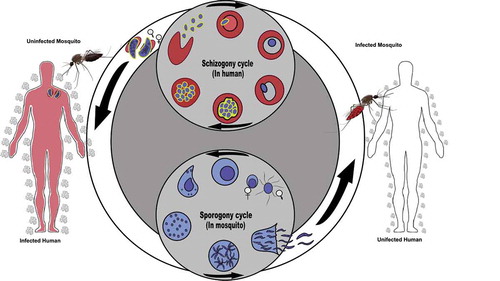
Mosquitoes’ unique bionomics guarantee an efficient vector–host interaction, favorable for malaria transmission. Mosquitoes use several senses to locate and choose a host, predominantly olfaction [Citation5,Citation6]. The semiochemical basis of human host seeking has been studied during past years [Citation7–Citation9], allowing us to further characterize the basis of this important behavior. The best-known cue for activation and attraction is carbon dioxide (CO2), which is emitted by vertebrates through red blood cells, breath and skin, and indicates the presence of a potential host [Citation6,Citation10,Citation11]. Mosquitoes also respond to the temperature of mammals and birds and the faster transport of odor by the heat convention current, resulting from metabolic activity [Citation12,Citation13]. Body heat and mass have a direct effect on the dispersal of chemicals, thus influencing mosquito [An. atroparvus and An. gambiae s.s.] attraction to the host behavior [Citation14]. Once mosquitoes detect, land, and start to feed on a suitable host the volume and speed at which blood is taken varies. This complex behavior is dependent on feeding stimulation, olfactory apparatus, host defense, and neurophysiological pathways connected to the mosquito’s mouthparts which consequently boost the basic reproduction rate of pathogen transmission as well [Citation5,Citation15,Citation16]. The avidity of the vector for a blood meal is measured as persistence (time spent feeding) and the number of times a mosquito attempts to find blood by inserting its proboscis (probing). Increased probing can enhance the chance of malaria parasite sporozoite transmission to the host blood vessels. It also risks being killed by the host defense or picking up of increased dose of insecticide. The volume of blood taken, and the speed of feeding has a direct effect on the likelihood of a mosquito becoming infected and subsequently transmitting the parasite [Citation17]. Larger blood meals increase the chances of taking up infectious gametocytes and therefore infection and faster feeding rates are correlated with higher survival of mosquitoes due to host bite avoidance behavior. Whatever the mechanisms responsible for blood seeking/feeding behavior, malaria parasite seems to modify them to increase its transmission.
Infamous pathogen vectors such as other anthropophilic anopheles such as An. funestus and An gambiae tend not to feed on sugar when human blood are available [Citation18]. Carbohydrate availability is important for the distance a mosquito is able to fly (mosquito flight range). Mosquitoes with low carbohydrate reserves have limited flight ability [Citation19]. Mosquitoes can also utilize part of the blood meal to produce the energy needed for flight; the remainder of the digestion products are converted to glycogen and triacylglycerol. Approximately 30% of the energy value of the blood meal is used for energy production in An. gambiae [Citation20]. Blood-fed An. gambiae can fly further than those fed on sugar alone [Citation14,Citation20], but An. atroparvus can fly further with sugar-only – this species does not seem to be able to use the lipids from blood efficiently for glucose production [Citation14,Citation20]. If extra sugar meal makes infectious mosquitoes capable of longer flight, they have an increased chance of finding a host, and therefore may have an increased likelihood of being a major risk for pathogen transmission.
Here, the direct effect of Plasmodium on (1) uninfected mosquito blood-seeking and feeding behavior, and (2) manipulation of its vector during sporogony cycle will be discussed in detail in the following sections (3). We will also summarize whether parasites are virulent to their vectors based on published literature. Finally, the next generation of methods for moving the empirical laboratory evidence to potential application in future integrated malaria control programs will be indicated.
Parasite–vector interplay for the transmission success
The complex biology of the Plasmodium parasite includes sexual development in their definitive hosts, particularly mosquito species within the genus Anopheles. The parasite cycle starts with the desire of mosquitoes to uptake blood. The mosquito must ingest male and female sexual forms of the parasite in order to become infected and capable of subsequently infecting another human host. Vector-borne parasites may also alter the vector’s behavior to increase their transmission ability [Citation21]. The mechanisms by which the presence of the malaria parasite could influence malaria transmission by altering the senses of the vectors are discussed below.
All malaria parasites require two hosts, the vertebrate host where schizogony occurs within the erythrocytes (human blood stages of parasite), and the mosquito vector where sporogony (mosquito stages of parasite) occurs in the mosquito midgut wall [Citation22]. In the vertebrate host, an infection is initiated when sporozoites are injected with the saliva of an infected mosquito, and parasites replicate asexually, first in the liver, and then in the erythrocytes (reviewed in [Citation23]). Whilst the majority of parasites invading erythrocytes are destined to continue through the asexual cycle, a small fraction can develop into gametocytes, the first sexual stage of the life cycle. In Plasmodium falciparum, the presence of gametocytes in the peripheral blood appears 7–15 days after the initial asexual wave which is long compared to the other human malaria species 1–3 days. The ratio of gametocytes to asexual stages in P. falciparum is usually found at very low levels [Citation24]. Only people carrying gametocytes can be infectious to mosquitoes. Recent studies showed that microscopy is insufficiently sensitive to detect low densities of asexual parasites and gametocyte [Citation25,Citation26]. In most endemic settings, a small proportion of infecting parasites are gametocytes [Citation27].
Mosquitoes become infected after biting malaria-infected people carrying the gametocytes; there is a complex period of sexual reproduction and development within the mosquito that results in sporozoite infection in the salivary glands, infective mosquitoes [Citation22]. Human malaria parasites alter the probing and persistence behavior of mosquitoes, resulting in increased transmission [Citation28]. There is also evidence that parasites and their metabolites affect both mosquito and human host physiology and behavior to increase the probability of transmission [Citation11,Citation21,Citation29–Citation33]. In rodent and bird malaria model systems, infected hosts have been found to be more attractive to mosquitoes [Citation30,Citation34]. The same has been observed for humans infected with both Plasmodium falciparum [Citation35] and Plasmodium vivax [Citation36]. It has also been reported that salivary gland malaria-infected mosquitoes show increased attraction to human odor [Citation37].
1 – Plasmodium direct the host-seeking mosquitoes toward infected hosts (manipulation beyond the host)
Does transmission begin with an ancient chemical coding? Mosquitoes favor malaria-infected individuals, especially hosts carrying gametocytes or parasite signaling metabolites, more frequently than uninfected individuals [Citation4,Citation11,Citation35,Citation36]. This phenomenon can be influenced by two routes, direct emission of volatile organic compounds and/or skin microbial composition (reviewed in [Citation4]). Increased vector attraction to the infected vertebrate host would provide an evolutionary advantage to the parasite as it increases host-vector contact, thus likely enhancing the chances of transmission [Citation38].
Emami et al. [Citation11] recently showed enhanced mosquito preference for blood containing a P. falciparum metabolite, (E)-4-hydroxy-3-methyl-but-2-enyl pyrophosphate (HMBPP) over parasite-free blood due to enhanced release of certain volatiles. In the field, western Kenya, children harboring gametocytes of P. falciparum attracted about twice as many mosquitoes compared to naturally infect with asexual stage and uninfected children [Citation35]. Increased vertebrate-host attraction during non-transmissible stages of the parasite would be disadvantageous to the malaria parasite since vertebrates frequently swat mosquitoes; hence, there is a higher risk of vector mortality. However, a recent study on attractiveness of P. vivax gametocyte carriers which has been grouped the patients into fever and non-fever categories. They showed a higher attractiveness to mosquitoes only in the fever group of gametocyte carriers, indicating a synergistic effect of temperature and gametocytes in the host attractiveness to Anopheles darlingi [Citation36]. There is a need for high-quality studies of the interactions and differences in clinical symptoms, associated pathological factors and gametocytaemia in malaria due to P. vivax and P. falciparum. In summary, we believe these studies usher in a new era in the investigation of the host–pathogen interaction with exciting opportunities to counteract the malarial scourge on human lives.
Plasmodium induced volatile organic compounds (VOCs) and mosquito olfaction
The olfactory apparatus of mosquitoes comprises three specialized head appendages, the antennae, maxillary palp and labella, that carry specialized sensory hairs called sensilla that typically contain two to three olfactory sensory neurons (OSNs). At the molecular level, OSNs are thought to express unique types of chemosensory receptors on dendritic membrane to detect a wide array of environmental odorants, reviewed in [Citation39]. Like in other insects, the genome of An. gambiae and Ae. aegypti encodes three classes of chemosensory receptors. These chemoreceptors include odorant receptors (Ors), ionotropic receptors (Irs) and gustatory receptors (Grs) that are devoted to detect a range of cues associated with mosquito host-seeking, feeding, oviposition and several other key behaviors that enhance their functional traits [Citation40,Citation41].
It is well documented that mosquito search behavior for hosts is triggered by olfactory perception of VOCs emitted from animals and humans [Citation30,Citation42,Citation43]. Plasmodium parasites are able to increase the infected host attraction to vectors by manipulating host-VOC profiles [Citation30,Citation44]. A recent study demonstrated increases in the production of the heptanal, octanal, nonanal, (E)-2-octenal, (E)-2-decenal and 2-octanone by malaria parasite-infected Kenyan children. Increases were broadly associated with infections with high malaria parasite load, compared to patients having either low malaria parasite density or being parasite-free [Citation44]. Kelly et al. [Citation45] reported that P. falciparum infected erythrocytes released few VOCs under in vitro culture conditions. Amounts of the monoterpene, α-pinene, were higher in the samples containing erythrocytes infected by P. falciparum strain 3D7-MR4 at 2% parasitemia compared to those bearing uninfected erythrocytes as well as to the samples containing infected erythrocytes treated with 5 µM of fosmidomycin [Citation45], an inhibitor of parasite isoprenoid biosynthesis by affecting the first enzyme of the MEP pathway, deoxyxylulose phosphate reductoisomerase [Citation46, Citation47]. Identification of other four compounds, namely, 3,5-bis (1,1-dimethylethyl)-4-methyl-1H-pyrazole, 6-hydroxy-5,7-dimethoxy-naphtho[2,3-c]furan-1(3H)-one, 4,5,9,10-dehydro-isolongifolene and 8,9-dehydro-9-formyl cycloisolongifolene is putative, due to insufficient match factors of mass spectra obtained from analytes and those available in electronic libraries as well as the absence of standards during identification [Citation45]. Recently, Emami and colleagues [Citation11] showed that a P. falciparum-derived compound, HMBPP, significantly enhances the release of CO2 by 16% in the headspace of treated erythrocytes compared to untreated cells. HMBPP addition also increased the release of three monoterpenes, α- and ß-pinene and limonene by 1.2-to-1.6 fold as well as three aldehydes octanal, nonanal and decanal by 1.7-to-5.2 fold. A mixture of all seven components was essential to trigger the host-seeking behavior of An. gambiae s.s. under laboratory conditions. These findings suggest the mechanism which underpins the Plasmodium’s requirement to manipulate their mosquito hosts in order to elevate the odds of infection to a degree sufficient for success at sustained transmission. These elements of the chemical signaling belonged to three main categories of volatiles, CO2, aldehydes and terpenes and appear highly evolved between these parasites and their definitive hosts, suggesting a powerful selection and survival advantage linked to it [Citation11].
A study using mice malaria-mosquito system revealed that mice at the chronic stage harboring relatively high levels of Plasmodium chabaudi (clone AS) gametocytes emitted significantly larger amounts of N,N-dibutylformamide, tridecane, 2-pyrrolidone, 2-hexanone, 2-methyl-butanoic acid, 2-phenyl ethanol and two unidentified compounds. In addition, amounts of two acids, namely 3-methyl butanoic and hexanoic acids tend to differ from the emissions of infected versus uninfected mice. It is interesting to note that in the 3 months after initial infection, volatile collections showed a decrease in overall volatile emissions from infected mice during the early, acute stage of infection compared to healthy controls as well as periodic cycles of elevated volatile emissions which corresponded to increase attractiveness of mice to mosquitoes [Citation30].
Of note, there are studies which followed infected individuals by detecting and quantifying parasite manipulated VOCs. Odors present in breath of symptomatic malaria-infected children (80% of pediatric patients with fever) and healthy individuals as a control were sampled in a medical facility. The samples were collected on absorbent resins in sorbent tubes which were later transported to the laboratory. The breath composition was analyzed and chromatographic patterns obtained from infected and healthy individuals were compared aiming to find malaria-associated volatile chemical markers. Data analyses revealed a cumulative abundance metric with a set of six VOCs, 4-methyl-undecane, 3,7-dimethyl-decane, 2,3,4-trimethyl-hexane, nonanal, tridecane and isoprene [Citation32]. In addition, it was found that infection correlates with significantly higher breath levels of two mosquito-attractant terpenes, α-pinene and 3-carene. This work was conducted in Malawi [Citation32]. However, another breathprint study of infected P. falciparum volunteers reported other compounds including isoprene, acetone, benzene, cyclohexanone, allyl methyl sulfide, 1-methylthio-propane, (Z)-1-methylthio-1-propene, and (E)-1-methylthio-1-propene [Citation48]. The major difference in these studies was that in the experimentally induced blood-stage malaria model, patients had very low parasitaemias (i.e. the percentage of RBCs that contained parasite to uninfected RBCs), being on the order of 100,000-fold lower than in the Malawian patients (parasitaemias ~2%). It is also plausible that Malawian patients were likely to harbor mature gametocytes which are the transmissible form of blood stage of parasite (human infection) to mosquitoes. A study of Kenyan patients also reported that malaria patients who carried the gametocyte stage of P. falciparum parasite were highly attractive to An. gambiae vectors [Citation49]. An extensive collections of skin volatiles from primary-school children with high rates of malaria infection in western Kenya revealed consistent effects of malaria infection on human volatile profiles, as well as significant divergence in the effects of symptomatic versus asymptomatic infections. Ten volatiles including ethyl-cyclohexane, ethyl-benzene, m-xylene or p-xylene, o-xylene, propylcyclohexane, 1-ethyl-3-methylbenzene, decane, nonanal, and two unidentified compounds were released at significantly higher amounts in asymptomatic compared to uninfected individuals. In symptomatic individuals, amounts of six compounds, namely, 2,4-dimethylhept-1-ene, 1-ethyl-3-methylbenzene, 1,2,4-trimethylbenzene, decane, s(-)-limonene, and 2-ethylhexan-1-ol were significantly higher compared to those in uninfected individuals () [Citation31]. These studies highlighted that the mature gametocyte (sexual stage of the parasite in human blood) could have been the key contributor to the VOCs [Citation50], chemical codes which communicate between mosquito and parasite to enhance the efficiency of parasite transmission (). Ultimately, what will be happened to parasite transmission success, once the decoding chemical information (VOCs) of parasite go straight to what the vector control strategies are conveying? We highlight key knowledge gaps that need to be addressed for the successful development of strategies that interrupt malaria transmission.
Figure 2. Plasmodium induced volatile organic compounds (VOCs).
Plasmodium associated compounds implicated in host responses (in vitro or in vivo studies). Chemical structures of volatile compounds have been shown. CAS indicates Chemical Abstracts Service which provides unique numerical identifier to every chemical substance described in the open scientific literature.
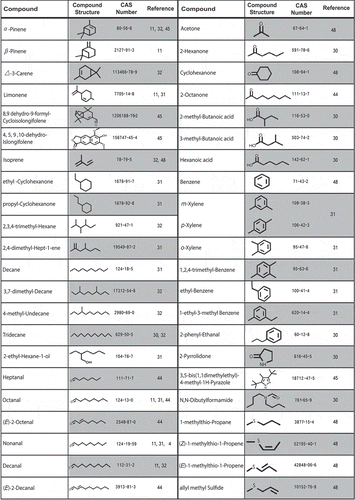
Plasmodium metabolite and mosquito gustation
Based on studies in human and other primates, two putative P. falciparum derived elicitors, glycosylphosphatidyl-inositols (GPIs) and hemozoin (Hz), have been investigated previously in mosquito vectors. GPIs function as universal cell membrane anchors for proteins and are the most common carbohydrate modification in Plasmodium [Citation51]. The GPI anchors are necessary for parasite survival and they must be synthesized de novo during intraerythrocytic stages [Citation52]. P. falciparum GPIs mediate many of the symptoms associated with severe human malaria pathophysiology. It also influences the mosquito immune system and suppressed fecundity strongly and the egg viability of An. gambiae (reviewed in [Citation53]). Provision of PfGPIs in the blood meal induces nitric oxide synthase (NOS) through extracellular signal-regulated kinase (ERK)/protein kinase B (Akt) signaling in the midgut of An. stephensi [Citation54]. P. falciparum GPIs also induce several AMP genes and decrease fecundity in An. gambiae mosquitoes [Citation55]. Plasmodium Hz is a brownish pigment generated during digestion of hemoglobin with host red blood cells [Citation56]. P. falciparum Hz can also induce NOS and stimulate An. gambiae and An. stephensi cell lines as well as in An. stephensi midgut tissues [Citation57].
Isoprenoids are widespread molecules and are necessary for all living organisms [Citation58]. These molecules are involved in a vast spectrum of metabolic processes and serve as building blocks in the synthesis of various compounds such as cholesterol, steroid hormones and vitamins [Citation59]. Animals, fungi and a few bacteria produce isoprenoids through a biosynthetic route called mevalonate pathway. By contrast, eubacteria, plastid-containing eukaryotes and most bacteria use an alternate metabolic route, the non-mevalonate or methylerythritol phosphate (MEP) pathway. Plants use both pathways, the chloroplast-localized MEP pathway that is used for biosynthesis of the terpene volatiles that contributs their characteristic flavors and fragrances [Citation60]. MEP pathway is used by parasitic apicomplaxan protozoa, including Plasmodium (reviewed in [Citation61]). The MEP pathway is one of the recognizable pathways in malaria parasite apicoplast and this pathway might have evolved due to its lower energy consumption (reviewed in [Citation45]). Due to its non-host specificity, biochemical reactions of MEP pathway have been favored as a highlighted target for novel antiparasitic drugs in human host. For example, fosmidomycin and its derivative, FR-900098 have an antibiotic activity that targets DOXP reductoisomerase and inhibits the growth of asexual stage of malaria parasite [Citation62]. Parasites lost their apicoplast after non-antifolate antibiotic treatments such as doxycycline. Interestingly, parasite growth (asexual stage) is rescued upon simultaneous supplementation with the central isoprenoid precursor, isopentenyl pyrophosphate (IPP) [Citation63].
Emami et al., 2017 recently published a compelling piece of evidence that the presence of one of the malaria parasite metabolites, called (E)-4-hydroxy-3-methyl-but-2-enyl pyrophosphate (HMBPP) in a blood meal, or in a meal of serum or physiological saline, is sufficient to stimulate 80–100% of the An. gambiae s.l. to engorge. This metabolite doubles the number of mosquitoes that will engorge on a blood meal alone [Citation11]. Previously, it has been shown that all blood-sucking insects have one phagostimulant in common, adenosine triphosphate (ATP) identified in mosquitoes [Citation64,Citation65]; tsetse flies [Citation66]; tabanids [Citation67]; simulids [Citation68]; fleas [Citation69] and rhodnius [Citation70]. With regards to malaria cycle, there is some evidence that Plasmodium metabolites, possibly other protozoans metabolites, and conserved signaling pathways might manipulate host/vector metabolism and feeding behavior, for offering a unique opportunity in the animal kingdom that intersect across more than 600 million years of evolution (reviewed in [Citation71]).
2 – Plasmodium manipulates its vector during the sporogony cycle (manipulation within the host)
Parasite development in the mosquito can also affect mosquito behavior. Mosquitoes are more avid to bite a host when malaria parasites have developed into transmissible sporozoites in the salivary glands. Laboratory evidence showed that feeding persistence of female An. stephensi mosquitoes depends on the presence and development stage of the parasite (Plasmodium yoelii nigeriensis). In 10-min trials, it was shown that 33% of uninfected, 53% of those harboring oocysts and only 20% of those infected with sporozoite mosquitoes, respectively, gave up feeding on human host [Citation72]. It seems that the feeding behavior of mosquitoes is not only mediated by the presence of parasite but also it is sensitive to the developmental stage of parasite. Mosquito are more eager to bite the host when malaria parasite has developed into transmissible sporozoites. Also, field study showed that sporozoite-infected mosquitoes took larger blood meals than uninfected mosquitoes and 82% of infected mosquitoes had engorged fully. In addition, individual mosquitoes carrying sporozoite were more likely to bite several people per night [Citation73]. Mosquitoes infected with sporozoites of Plasmodium gallinaceum needed a larger blood meal to inhibit host-seeking behavior than those not infected [Citation74]. For example, An. gambiae and Anopheles punctulatus infected by P. falciparum and P. vivax at sporozoite stage attempted to take more blood meals than uninfected mosquitoes, a behavior which could increase the rate of parasite transmission by increasing their biting rate [Citation17,Citation73].
Plasmodium and Anophelines interplay in response to the interventions against them
Complex biology of the plasmodium parasite, differences in vector capacity as well as biological and ecological features of vector mosquitoes requires integrated approach to malaria control [Citation75]. To achieve malaria reduction and elimination goals, the World Health Organization (WHO) has recommended wide-scale actions falling into three categories: (i) efficient diagnosis and treatment of disease; (ii) quality-assured vector control; and (iii) preventive treatments [Citation76].
The clinical symptoms of malaria are nonspecific. Consequently, parasite-specific laboratory tests must be carried out to confirm the infection [Citation77]. Currently, microscopic examination of stained blood films and rapid diagnostic tests (RDTs) detecting parasite antigens are the most common tests [Citation78]. In 2016 alone, an estimated 312 million RDTs were delivered globally, of which 269 million were in Africa [Citation79]. Most of RDTs tests rely on the detection of the particular pathogen’s proteins. For example, in the case of the malaria, P. falciparum histidine-rich protein HRP2 is selected. The hrp2 gene, however, is dispensable, and parasite strains that have deleted this gene and escape RDT detection are on the rise. In a recent modeling study, false-negative rates with HRP2-based tests were predicted to attain 20% in many parts of Africa by 2030 [Citation80]. Nucleic acid amplification tests (NAAT) are superior for detecting mixed infections, cases with low parasitemia. Nucleic acid amplification tests also use commonly for the identification of species when the parasite morphology is inadequate for microscopic identification. Although nucleic acid amplification tests resolve discrepant results compare to other methodologies, however, the method is expensive and highly-complex [Citation78]. Serologic tests are used in cases where parasites are not seen on peripheral blood smears, for screening donors and in some other occasions. However, the tests cannot differentiate between past and current infections [Citation78]. There are also different lines of research for identifying malaria parasites in blood smears using new methods such as a Focal Plane Array (FPA), Fourier transform infrared imaging spectroscopy. This technique, in combination with hyper-spectral processing, enables imaging and diagnosis of early stage malaria parasites at the single cell level in a blood smear [Citation81]. The next one is magneto-optic technology which is also used for early stage malaria diagnosis in human based on the detection of the malaria pigment, hemozoin [Citation82]. These methods will be challenging; however, recent advances suggest that they may be a possibility in the foreseeable future.
The accumulating data that pathogens manipulate host odor profiles to influence vector behavior reveal a possibility for developing new non-interventional, volatile-based malaria detection methods that have to be reliable, user-friendly, and affordably for the screening of human populations. Number of studies dealing with VOCs collection from human skin, breath, or blood showed volatile signature of parasite presence. Certain volatiles have been repeatedly found as indicators of the presence of malaria parasite, for example, octanal, nonanal, decanal, isoprene, tridecane, α-pinene and limonene (). However, a single study has been reported that the amount of these volatiles can significantly differ with respect to the presence/absence of parasite as well as the presence of various parasite stages with regard to large structural diversity (). Moreover, so far no parasite-specific compound has been identified, except in the study that is carried out by Kelly et al. [Citation47] where identifications remain putative and have to be confirmed. The absence of parasite-specific volatiles is in accordance with the deceptive chemical signal hypothesis that predicts parasite manipulates existing host-cues rather than produces novel signals resulting in increased attractiveness of infected vertebrate hosts [Citation83,Citation84]. It is much more challenging to build a model that classifies samples based on quantitative rather than the qualitative difference of diagnostic compounds. The next step will be to determine whether quantitative differences of diagnostic volatiles between healthy and different stage malaria bearing individuals are large enough with respect to genetic variation among individuals, geographical regions, and diet, as well as the presence of other pathogens and parasites.
Prevention of human contacts with malaria vectors relies almost wholly upon a few essential tools that greatly mitigate the harm done by malaria [Citation79]. These tools include long-lasting insecticide-treated netting (LLINs), and indoor residual spraying of insecticides (IRS) where the latter method strongly contributed to malaria decline in sub-Saharan Africa [Citation85]. Mosquitoes show an impressive ability to adapt to new environments; consequently, LLINs and IRS became vulnerable to the development of mosquito resistance and behavior changes. For example, mosquito resistance to the pyrethroid insecticides exclusively used in LLINs is emerging [Citation86] and in the case of the many hundreds of millions of LLINs distributed for minimizing human contact with the night-feeding anophelines, selection for anophelines feeding earlier in the evening (before people retreat to their bed nets) or outdoors is occurring [Citation87]. The rapid development of eco-friendly formulations of novel insecticides [Citation88,Citation89] and nanostructured materials [Citation90] is a temporal solution to the vector control problem. Use of attractive toxic sugar baits (ATSB) had an environmental issue due to the lack of mosquito attraction specificity, and targeting beneficial insect including pollinators. Highly mosquito attractive synthetic odor blend mimicking human smell has been reported [Citation91], which in turn could be further improved by imitating malaria-infected human dour. Malaria-infected human dour might specifically attract mosquitoes to toxic agent containing HMBPP, which stimulates toxin uptake. This lure and kill set up could be environment-friendly alternative to ATSB.
Eco-friendly mosquito control strategies such as sterile insect technique (SIT) and Wolbachia driven population suppression techniques as well as insects carrying a dominant lethal (RIDL) require mass production of mosquitoes [Citation92,Citation93]. Production of high-quality mosquitoes in mass amounts requires optimized breeding solutions, where phagostimulants are essential component ensuring sufficient engorgement rates of a meal. Adenosine triphosphate (ATP) is the most effective phagostimulant known for mosquitoes, however, ATP cannot be stored at room temperature and is relatively unstable in aqueous solutions [Citation83]. Finding a replacement compound for ATP is therefore desirable. HMBPP is reported as a stable compound and is efficient as a phagostimulant at low concentrations. HMBPP might have the ability to replace ATP and reduce breeding cost in the mass production of mosquitoes.
The discovery of parasite metabolites (e.g. HMBPP) signaling points to a highly evolved ‘trick’ by the plasmodium spp., a chemical beacon guiding the anophelines not only to the rare host actually carrying parasites (as in most endemic areas globally, i.e., <2% prevalence), but also to sites on that host tissue bearing parasites for consumption [Citation11,Citation30,Citation31]. These effects observed at miniscule concentrations (picomolar), suggesting an obligate dependency upon parasite signaling to improve the odds of mosquito infection. It is plausible that transmission in the wild may be unsustainable in the absence of the parasite chemical beacon. If so, switching off that beacon by any one of the many possible technological means may suffice to suppress the efficiency of anopheline infection to below sustainable levels. Those ways and means effectively represent a wide range of possible new molecular targets (in mosquitoes, parasites, and humans) for blocking malaria transmission among humans. At present, biochemical, genetic and crystallographic approaches with the MEP pathway enzymes are now starting to characterize the inhibition kinetics and identify which residues play a structural or catalytic role. However, there is still a gap in our knowledge regarding the transportation of these compounds out of malaria parasite-infected cells. The underlying mechanism by which HMBPP transports and mediates signaling has not been investigated. Current efforts should eventually contribute to an effective drug designed to fight against pathogens that show resistance to currently available agents.
A genetics-based population suppression and elimination approach is yet another strategy which might provide a better control to vector-borne diseases. In this system, the main problem is the means of driving a refractory construct quickly and efficiently through the vector mosquito population so that the population of susceptible mosquitoes will be replaced. Transposable elements (TEs) were one of the first gene drive systems to gain widespread attention for population replacement [Citation94]. These elements are able to spread quickly through a population due to their ability to replicate within a host genome and hence to be inherited more frequently in the offspring’s genome. This increase in inheritance enables TEs to spread even in the presence of a fitness cost to the host [Citation95]. One note, new techniques such as CRISPR-Cas9 gene drive targeting doublesex have also been reported a complete population suppression in caged An. gambiae mosquitoes [Citation96]. Obviously, there is some doubts about the potential success of this strategy in malaria-endemic regions, where harboring a resistance allele will only be a piece of the complex puzzle of pathogen–vector interactions. Apart from the undeniable social and ethical issues associated with the release of genetically modified organisms [Citation97], transgenic mosquitoes might still be considered as a realistic strategy for malaria control. The vigorous and technologically cutting-edge effort to produce a vaccine against malaria over the past four decades has yet to yield a useful product [Citation98].
Finally, vector-borne disease caused by mosquitoes has been a threat to billions of human life around the globe. In such setting, the development of any new methods from novel mosquitoes lures to genetics-based population techniques that can control the transmission of the pathogens and/or population suppression and elimination ability is a promising approach. These new hopes could potentially reduce threats exerted by mosquitoes to humans. The work carried out so far has shown successful results in the laboratory can be the leading strategies in the future to control mosquito-borne diseases.
Concluding remarks
The ability of parasites to manipulate the behavior of their hosts fascinates both scientists and non-scientists alike. Undoubtedly, the design and interpretation of investigations in this field is hampered by our inadequate understanding of the natural-based studies. Here we highlight the studies which show 1. Plasmodium can direct uninfected mosquito blood-seeking and feeding behavior and 2. Plasmodium also manipulates its vector during sporogony cycle to increase transmission. Ultimately, we discuss whether parasites are virulent to their vector-based on published literature. In our idea, the utility of studying the natural system of vector-host–parasite manipulations is to use them as potential avenues for further research into vector control strategies. We require new methods/tools to combat this scourge in order to avoid catastrophic loss of lives as conventional tools become increasingly ineffective or economically unsustainable. Although there is much in this age that is new, many of the current debates and arguments about malaria control are echoes of the past. History shows that gains made against malaria parasite are invariably eroded with great rapidity unless driven to local extinction. Experience from the past is a vital tool in the formulation of new malaria control. An understanding of the evolution and context of those challenges and innovative ideas can help us to direct the malaria control of today and the future.
Author contributions
All authors listed have made a direct, and intellectual contribution to the work and approved the manuscript for publication.
Acknowledgments
The authors thank members of the Emami lab for careful reading of the manuscript and for helpful discussions. We are grateful to Dr. Jeremy Adler for proofreading of the manuscript.
Disclosure statement
No potential conflict of interest was reported by the authors.
Additional information
Funding
References
- Davidson G, Draper CC. Field studies of some of the basic factors concerned in the transmission of malaria. Trans R Soc Trop Med Hyg. 1953;47:522–535.
- Smith DL, Battle KE, Hay SI, et al. Ross, macdonald, and a theory for the dynamics and control of mosquito-transmitted pathogens. PLoS Pathog. 2012;8:e1002588.
- Lemasson JJ, Fontenille D, Lochouarn L, et al. Comparison of behavior and vector efficiency of Anopheles gambiae and An. arabiensis (Diptera: Culicidae)in Barkedji, a Sahelian area of Senegal. J Med Entomol. 1997;34:396–403.
- Busula AO, Verhulst NO, Bousema T, et al. Mechanisms of Plasmodium-enhanced attraction of mosquito vectors. Trends Parasitol. 2017;33:961–973.
- Bowen MF. The sensory physiology of host-seeking behavior in mosquitoes. Annu Rev Entomol. 1991;36:139–158.
- Takken W, Knols BG. Odor-mediated behavior of Afrotropical malaria mosquitoes. Annu Rev Entomol. 1999;44:131–157.
- Carey AF, Wang G, Su CY, et al. Odorant reception in the malaria mosquito Anopheles gambiae. Nature. 2010;464:66–71.
- Omondi BA, Majeed S, Ignell R. Functional development of carbon dioxide detection in the maxillary palp of Anopheles gambiae. J Exp Biol. 2015;218:2482–2488.
- Rinker DC, Pitts RJ, Zhou X, et al. Blood meal-induced changes to antennal transcriptome profiles reveal shifts in odor sensitivities in Anopheles gambiae. Proc Natl Acad Sci USA. 2013;110:8260–8265.
- Frame GW, Strauss WG, Maibach HI. Carbon dioxide emission of the human arm and hand. J Invest Dermatol. 1972;59:155–159.
- Emami SN, Lindberg BG, Hua S, et al. A key malaria metabolite modulates vector blood seeking, feeding, and susceptibility to infection. Science. 2017;355:1076–1080.
- Khan AA, Maibach HI, Strauss WG. The role of convection currents in mosquito attraction to human skin. Mosq News. 1968;28:462–464.
- Takken W, Verhulst N. Host preferences of blood-feeding mosquitoes. Annu Rev Entomol. 2013;58:433–453.
- De Jong R, Knols BG. Selection of biting sites on man by two malaria mosquito species. Experientia. 1995;51:80–84.
- Scott TW, Takken W. Feeding strategies of anthropophilic mosquitoes result in increased risk of pathogen transmission. Trends Parasitol. 2012;28:114–121.
- Jung JW, Baeck S-J, Perumalsamy H, et al. A novel olfactory pathway is essential for fast and efficient blood-feeding in mosquitoes. Sci Rep. 2015;5:13444.
- Koella JC, Packer MJ. Malaria parasites enhance blood-feeding of their naturally infected vector Anopheles punctulatus. Parasitology. 1996;113(Pt 2):105–109.
- Gary RE Jr., Foster WA. Diel timing and frequency of sugar feeding in the mosquito Anopheles gambiae, depending on sex, gonotrophic state and resource availability. Med Vet Entomol. 2006;20:308–316.
- Klowden MJ, Briegel H. Mosquito gonotrophic cycle and multiple feeding potential: contrasts between Anopheles and Aedes (Diptera: Culicidae). J Med Entomol. 1994;31:618–622.
- Kaufmann C, Briegel H. Flight performance of the malaria vectors Anopheles gambiae and Anopheles atroparvus. J Vector Ecol. 2004;29:140–153.
- Cator LJ, Lynch PA, Read AF, et al. Do malaria parasites manipulate mosquitoes? Trends Parasitol. 2012;28:466–470.
- Baton LA, Ranford-Cartwright LC. Spreading the seeds of million-murdering death: metamorphoses of malaria in the mosquito. Trends Parasitol. 2005;21:573–580.
- Cowman AF, Healer J, Marapana D, et al. Malaria: biology and disease. Cell. 2016;167:610–624.
- Talman AM, Domarle O, McKenzie FE, et al. Gametocytogenesis: the puberty of Plasmodium falciparum. Malar J. 2004;3:24.
- Vafa Homann M, Emami SN, Yman V, et al. Detection of malaria parasites after treatment in travelers: A 12-months longitudinal study and statistical modelling analysis. EBioMedicine. 2017;25:66–72.
- Stone W, Goncalves BP, Bousema T, et al. Assessing the infectious reservoir of falciparum malaria: past and future. Trends Parasitol. 2015;31:287–296.
- Taylor LH, Read AF. Why so few transmission stages? Reproductive restraint by malaria parasites. Parasitol Today. 1997;13:135–140.
- Schwartz A, Koella JC. Trade-offs, conflicts of interest and manipulation in Plasmodium-mosquito interactions. Trends Parasitol. 2001;17:189–194.
- Koella JC. Malaria as a manipulator. Behav Processes. 2005;68:271–273.
- De Moraes CM, Stanczyk NM, Betz HS, et al. Malaria-induced changes in host odors enhance mosquito attraction. Proc Natl Acad Sci USA. 2014;111:11079–11084.
- De Moraes CM, Wanjiku C, Stanczyk NM, et al. Volatile biomarkers of symptomatic and asymptomatic malaria infection in humans. Proc Natl Acad Sci USA. 2018;115:5780–5785.
- Schaber CL, Katta N, Bollinger LB, et al. Breathprinting reveals malaria-associated biomarkers and mosquito attractants. J Infect Dis. 2018;217:1553–1560.
- Berna A, Schaber C, Katta N, et al. Breathprinting reveals malaria-associated biomarkers and mosquito attractants. In Vitro Cell Dev-An. 2018;54:S7–S7.
- Cornet S, Nicot A, Rivero A, et al. Malaria infection increases bird attractiveness to uninfected mosquitoes. Ecol Lett. 2013;16:323–329.
- Lacroix R, Mukabana WR, Gouagna LC, et al. Malaria infection increases attractiveness of humans to mosquitoes. PLoS Biol. 2005;3:e298.
- Batista EP, Costa EF, Silva AA. Anopheles darlingi (Diptera: Culicidae) displays increased attractiveness to infected individuals with Plasmodium vivax gametocytes. Parasit Vectors. 2014;7:251.
- Smallegange RC, van Gemert G-J, van de Vegte-bolmer M, et al. Malaria infected mosquitoes express enhanced attraction to human odor. Plos One. 2013;8:e63602.
- Hurd H. Manipulation of medically important insect vectors by their parasites. Annu Rev Entomol. 2003;48:141–161.
- Suh E, Bohbot J, Zwiebel LJ. Peripheral olfactory signaling in insects. Curr Opin Insect Sci. 2014;6:86–92.
- Tauxe GM, MacWilliam D, Boyle SM, et al. Targeting a dual detector of skin and CO2 to modify mosquito host seeking. Cell. 2013;155:1365–1379.
- Suh E, Choe DH, Saveer AM, et al. Suboptimal larval habitats modulate oviposition of the malaria vector mosquito Anopheles coluzzii. PLoS One. 2016;11:e0149800.
- van Loon JJ, Smallegange RC, Bukovinszkiné-Kiss G, et al. Mosquito attraction: crucial role of carbon dioxide in formulation of a five-component blend of human-derived volatiles. J Chem Ecol. 2015;41:567–573.
- Zwiebel LJ, Takken W. Olfactory regulation of mosquito-host interactions. Insect Biochem Mol Biol. 2004;34:645–652.
- Robinson A, Busula AO, Voets MA, et al. Plasmodium-associated changes in human odor attract mosquitoes. Proc Natl Acad Sci USA. 2018;115:E4209–E4218.
- Kelly M, Su C-YSchaber C, et al. Malaria parasites produce volatile mosquito attractants. Mbio. 2015;6:e00235–15.
- Zhang B, Watts KM, Hodge D, et al. A second target of the antimalarial and antibacterial agent fosmidomycin revealed by cellular metabolic profiling. Biochemistry. 2011;50:3570–3577.
- Heuston S, Begley MGahan CG, et al. Isoprenoid biosynthesis in bacterial pathogens. Microbiology. 2012;158:1389–1401.
- Berna AZ, McCarthy JS, Wang RX, et al. Analysis of breath specimens for biomarkers of Plasmodium falciparum infection. J Infect Dis. 2015;212:1120–1128.
- Busula AO, Bousema T, Mweresa CK, et al. Gametocytemia and attractiveness of Plasmodium falciparum-infected Kenyan children to Anopheles gambiae mosquitoes. J Infect Dis. 2017;216:291–295.
- Liu C, Emami SN, Pettersson J, et al. Vgamma9Vdelta2 T cells proliferate in response to phosphoantigens released from erythrocytes infected with asexual and gametocyte stage Plasmodium falciparum. Cell Immunol. 2018;334:11–19.
- Gowda DC, Gupta P, Davidson EA. Glycosylphosphatidylinositol anchors represent the major carbohydrate modification in proteins of intraerythrocytic stage Plasmodium falciparum. J Biol Chem. 1997;272:6428–6439.
- Macrae JI, Lopaticki S, Maier AG, et al. Plasmodium falciparum is dependent on de novo myo-inositol biosynthesis for assembly of GPI glycolipids and infectivity. Mol Microbiol. 2014;91:762–776.
- Arrighi RB, Faye I. Plasmodium falciparum GPI toxin: a common foe for man and mosquito. Acta Trop. 2010;114:162–165.
- Lim J, Gowda DC, Krishnegowda G, et al. Induction of nitric oxide synthase in Anopheles stephensi by Plasmodium falciparum: mechanism of signaling and the role of parasite glycosylphosphatidylinositols. Infect Immun. 2005;73:2778–2789.
- Arrighi RB, Debierre-Grockiego F, Schwarz RT, et al. The immunogenic properties of protozoan glycosylphosphatidylinositols in the mosquito Anopheles gambiae. Dev Comp Immunol. 2009;33:216–223.
- Francis SE, Sullivan DJ Jr., Goldberg DE. Hemoglobin metabolism in the malaria parasite Plasmodium falciparum. Annu Rev Microbiol. 1997;51:97–123.
- Akman-Anderson L, Olivier M, Luckhart S. Induction of nitric oxide synthase and activation of signaling proteins in Anopheles mosquitoes by the malaria pigment, hemozoin. Infect Immun. 2007;75:4012–4019.
- Belmant C, Espinosa E, Halary F, et al. A chemical basis for selective recognition of nonpeptide antigens by human delta T cells. Faseb J. 2000;14:1669–1670.
- Morita CT, Jin C, Sarikonda G, et al. Nonpeptide antigens, presentation mechanisms, and immunological memory of human Vgamma2Vdelta2 T cells: discriminating friend from foe through the recognition of prenyl pyrophosphate antigens. Immunol Rev. 2007;215:59–76.
- Frank A, Groll M. The methylerythritol phosphate pathway to Isoprenoids. Chem Rev. 2017;117:5675–5703.
- Rohmer M. The discovery of a mevalonate-independent pathway for isoprenoid biosynthesis in bacteria, algae and higher plants. Nat Prod Rep. 1999;16:565–574.
- Jomaa H, Wiesner J, Sanderbrand S, et al. Inhibitors of the nonmevalonate pathway of isoprenoid biosynthesis as antimalarial drugs. Science. 1999;285:1573–1576.
- Yeh E, DeRisi JL, Striepen B. Chemical rescue of malaria parasites lacking an apicoplast defines organelle function in blood-stage Plasmodium falciparum. PLoS Biol. 2011;9:e1001138.
- Galun R. The evolution of purinergic receptors involved in recognition of a blood meal by hematophagous insects. Mem Inst Oswaldo Cruz. 1987;82(Suppl 3):5–9.
- Werner-Reiss U, Galun R, Crnjar R, et al. Factors modulating the blood feeding behavior and the electrophysiological responses of labral apical chemoreceptors to adenine nucleotides in the mosquito Aedes aegypti (Culicidae). J Insect Physiol. 1999;45:801–808.
- Galun R, Margalit J. Adenine nucleotides as feeding stimulants of the tsetse fly Glossina austeni Newst. Nature. 1969;222:583–584.
- Lall SB. Feeding behavior of haematophagous tabanids (Diptera). J Med Entomol. 1970;7:115–119.
- Friend WG, Smith JJ. Factors affecting feeding by bloodsucking insects. Annu Rev Entomol. 1977;22:309–331.
- Galun R, Kindler SH. Chemical specificity of the feeding response in Hirudo medicinalis (L.). Comp Biochem Physiol. 1966;17:69–73.
- Friend WG, Smith JJ. Feeding in Rhodnius prolixus: mouthpart activity and salivation, and their correlation with changes of electrical resistance. J Insect Physiol. 1971;17:233–243.
- Luckhart S, Riehle MA. The insulin signaling cascade from nematodes to mammals: insights into innate immunity of Anopheles mosquitoes to malaria parasite infection. Dev Comp Immunol. 2007;31:647–656.
- Anderson RA, Koella JC, Hurd H. The effect of Plasmodium yoelii nigeriensis infection on the feeding persistence of Anopheles stephensi Liston throughout the sporogonic cycle. Proc R Soc B Biol Sci. 1999;266:1729–1733.
- Koella JC, Sorensen FL, Anderson RA. The malaria parasite, Plasmodium falciparum, increases the frequency of multiple feeding of its mosquito vector, Anopheles gambiae. Proc Biol Sci. 1998;265:763–768.
- Koella JC, Sorense FL. Effect of adult nutrition on the melanization immune response of the malaria vector Anopheles stephensi. Med Vet Entomol. 2002;16:316–320.
- Shiff C. Integrated approach to malaria control. Clin Microbiol Rev. 2002;15:278–293.
- Sergiev VP, Gasymov EI. The world health organization new global technical strategy for 2015–2030. Med Parazitol (Mosk). 2017;1:59–62.
- World Health Organization (WHO). Guideline for the treatment for malaria. 3rd ed. Geneva, Switzerland; 2015.
- Mathison BA, Pritt BS, Kraft CS. Update on malaria diagnostics and test utilization. J Clin Microbiol. 2017;55:2009–2017.
- World Health Organization (WHO). World malaria report. Geneva, Switzerland; 2018.
- Aung T, White C, Montagu D, et al. Improving uptake and use of malaria rapid diagnostic tests in the context of artemisinin drug resistance containment in eastern Myanmar: an evaluation of incentive schemes among informal private healthcare providers. Malar J. 2015;14:105.
- Wood BR, Bambery KR, Dixon MWA, et al. Diagnosing malaria infected cells at the single cell level using focal plane array Fourier transform infrared imaging spectroscopy. Analyst. 2014;139:4769–4774.
- McBirney SE, Chen D, Scholtz A, et al. Rapid diagnostic for point-of-care malaria screening. ACS Sens. 2018;3:1264–1270.
- Gonzales KK, Hansen IA. Artificial diets for mosquitoes. Int J Environ Res Public Health. 2016;13:1267.
- Mauck KE, De Moraes CM, Mescher MC. Deceptive chemical signals induced by a plant virus attract insect vectors to inferior hosts. Proc Natl Acad Sci USA. 2010;107:3600–3605.
- Benelli G, Beier JC. Current vector control challenges in the fight against malaria. Acta Trop. 2017;174:91–96.
- Strode C, Donegan S, Garner P, et al. The impact of pyrethroid resistance on the efficacy of insecticide-treated bed nets against African anopheline mosquitoes: systematic review and meta-analysis. PLoS Med. 2014;11:e1001619.
- Yohannes M, Boelee E. Early biting rhythm in the Afro-tropical vector of malaria, Anopheles arabiensis, and challenges for its control in Ethiopia. Med Vet Entomol. 2012;26:103–105.
- Alkenani NA. Influence of the mixtures composed of slow-release insecticide formulations against Aedes aegypti mosquito larvae reared in pond water. Saudi J Biol Sci. 2017;24:1181–1185.
- Isman MB. A renaissance for botanical insecticides? Pest Manag Sci. 2015;71:1587–1590.
- Mishra P, Tyagi BK, Chandrasekaran N, et al. Biological nanopesticides: a greener approach towards the mosquito vector control. Environ Sci Pollut Res Int. 2018;25:10151–10163.
- Okumu FO, Killeen GF, Ogoma S, et al. Development and field evaluation of a synthetic mosquito lure that is more attractive than humans. PLoS One. 2010;5:e8951.
- Lees RS, Knols B, Bellini R, et al. Review: improving our knowledge of male mosquito biology in relation to genetic control programmes. Acta Trop. 2014;132(Suppl):S2–11.
- Mains JW, Brelsfoard CL, Rose RI, et al. Female adult Aedes albopictus suppression by Wolbachia-infected male mosquitoes. Sci Rep. 2016;6:33846.
- Ribeiro JM, Kidwell MG. Transposable elements as population drive mechanisms: specification of critical parameter values. J Med Entomol. 1994;31:10–16.
- Charlesworth B, Sniegowski P, Stephan W. The evolutionary dynamics of repetitive DNA in eukaryotes. Nature. 1994;371:215–220.
- Kyrou K, Hammond AM, Galizi R, et al. A CRISPR-Cas9 gene drive targeting doublesex causes complete population suppression in caged Anopheles gambiae mosquitoes. Nat Biotechnol. 2018;36:1062–1066.
- Lambrechts L, Koella JC, Boete C. Can transgenic mosquitoes afford the fitness cost? Trends Parasitol. 2008;24:4–7.
- Coelho CH, Doritchamou JYA, Zaidi I, et al. Advances in malaria vaccine development: report from the 2017 malaria vaccine symposium. NPJ Vaccines. 2017;2:34.