Abstract
l-Cysteine-modified dendritic fibrous nanosilica grafted by amine groups (Cys-DFNS-NH2) have been synthesized by a novel hydrothermal method. The N2 adsorption–desorption isotherms analysis on the Cys-DFNS-NH2 show that the average pore volume and surface area of the prepared fibrous Cys-DFNS-NH2 were 2.2 cm3/g and 205 m2/g, respectively, while the average pore size is 6.06 nm. Adsorption behavior of the Cys-DFNS-NH2 for Cd2+, Cu2+, Ag+, and Pb2+ was investigated by electrochemical methods. The results show that Cys-DFNS-NH2 can selectively adsorb Cd2+, Ag+, and Pb2+ in different potentials. Finally, the application of the engineered adsorbent for the removal of Cd2+, Ag+, and Pb2+ from contaminated water samples was examined. This work provides a new platform to the synthesis of Cys-DFNS-NH2 with high specific surface area for efficient adsorbent of specific metal ions.
Graphical Abstract
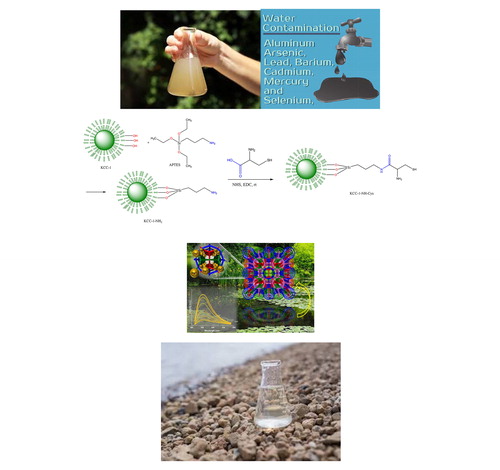
Introduction
Water polluted by toxic compounds is an important environmental issue. Recently, there has been a growing interest in materials capable of removing toxic compounds and ions from contaminated water. The removal of toxic metal ions, polyaromatic hydrocarbons, phosphorus, etc., from water is a critical environmental concern. Recently, there is a growing demand for the application of super-adsorbents for the removal of metals ions and organic pollutants such as oil, dyes, and volatile organic compounds from contaminated water [Citation1–6].
Among different methods, adsorption suggest many advantages such as simple and stable operation, easy handling of waste, absence of toxic reagents, compact facilities, and generally lowers operation cost [Citation7,Citation8]. Adsorption is a fundamental process involving the enrichment of guest species at the interface of a certain adsorbent [Citation9–11]. Such a process provides one of the most efficient ways to dramatically reduce release of contaminations [Citation12,Citation13]. Therefore, one of the most efficient routes for removal of metal ions is adsorption-based processes. The fundamental and great challenge is developing highly efficient adsorbents. An important requirement for an excellent adsorbent is a large substrate [Citation7]. Mesoporous nanomaterials with large pore volume and uniform mesopores show a fast removal of contaminations with high efficiency. Traditionally, functionalized mesoporous silica materials are widely adopted as adsorbents [Citation7]. Due to their high porosity, mesoporous silica nanomaterials with more sophisticated functions are of great interest for adsorption processes and separation of contaminations from waste waters [Citation7]. Dendritic fibrous nano-silica is one of the recently introduced nanomaterials by Polshettiwar and coworkers [Citation14,Citation15] which showed excellent potential in energy storage, sensor technology, catalysis, gas capture, solar energy harvesting, and biomedical applications. According to the fibrous morphology of these materials, they are named fibrous nano-silica (KCC-1) [Citation14]. However, the fibers are more like thin sheets of 3.5 to 5.2 nm thickness (like petals of flowers) rather than sharp needle shaped rods, and hence several other names have been used in the literature, such as fibrous, wrinkled, nanoflower, dendritic, dandelion, lamellar, etc.
The uniqueness of DFNS is mainly due to its dendritic fibrous morphology, which is in principle accessible from all sides (as compared to the tubular pores of MCM-41 and SBA-15). This improved accessibility allowed for increased loading of active sites (such as metals, metal oxides, organometallics, organic molecules, etc.) onto the silica surface without blocking the pores (channels) and, lead to an increment in accessibility of these generated active sites. The surface area of DFNS can be differ from 450 m2g–1 to 1244 m2g–1 again font size by tuning their particle size (from 40to 1120 nm) and fiber density (number of fibers within one sphere) [Citation15]. Importantly, unlike the tiny pore size distribution in conventional silica materials, DFNS possesses radially oriented pores (fibrous channels), the size of which increase from the center of the sphere to its outer surface. Control over the fiber density of DFNS allowed for tunable pore size and pore volume. Therefore, its tunable pore size (from 3.7 to 25 nm) allowed for tailor-made pores to fit specific guest molecules with various sizes, while its tunable pore volume (up to 2.18 cm3/g) allowed for high loadings of these guests. Guests can be organometallic complexes, organic molecules, metals, metal oxides, peptides, inorganic salts, proteins, enzymes, polymers, carbon, etc. DFNS also show excellent stability (chemical, mechanical, thermal, and hydrothermal), which is of key importance in conventional mesoporous materials due to their silica walls. Interestingly, these materials have low toxicity and are biocompatible. Control over the particle size allows for the fine tuning of the dispersion, uptake, and toxicity of these nanospheres.
Interestingly, Huang and coworkers, applied lanthanum (La)-doped DFNS for the adsorption of phosphorus [Citation16]. Also, Xie et al., grafted DFNS with cyano groups and used these for the removal of some ions (Fe3+, Cu2+, and Pb2+) from waste contaminated solution [Citation17]. Also, Yan and coworker used multifunctional and magnetic DFNS for both the removal of Zn2+ ions and their detection (sensing probe) [Citation18].
Amine functionalized mesoporous silica [Citation19–22] have been used as super-adsorbent for metal cations, phosphate and nitrate anions, and chromate and arsenat oxyanions [Citation23–25]. Application of these materials for the removal of metals cations has been the subject of extensive research recently, while our knowledge of the adsorption of cations using silica-based mesoporous materials is, comparatively, incomplete. Following our work on synthesis of new modified mesoporous sorbents [Citation26] in this work, the synthesis of amino-functionalized DNFS functionlized by l-Cysteine (l-Cys), its properties and sorption behavior for some cations from water samples using electrochemical methods was investigated.
Experimental
Materials
Tetraethyl orthosilicate (TEOS, 98%), (3-aminopropyl)triethoxysilane (APTES, 99%), N-hydroxysuccinimide (NHS, 98.0%), and 1-ethyl-3-(3-dimethylaminopropyl) carbodiimide (EDC, 98.0%) were purchased from Sigma-Aldrich Co. (USA). Cyclohexane, toluene, hexanol, dimethylsulfoxide (DMSO), and cetyltrimethylammonium bromide (CTAB) were obtained from Merck (Germany).
Instruments
Electrochemical cyclic voltammetry (CV), differential pulse voltammetry (DPV), and square wave voltammetry (SWV) measurements were carried out using a potentiostat/glavanostat system AUTOLAB system with PGSTAT302N boards (Eco Chemie, Utrecht, Netherlands) at room temperature, using a standard electrochemical cell with three-electrodes. A conventional three-electrode electrochemical cell comprising glassy carbon electrode (GCE), Ag/AgCl/KCl (saturated) and platinum wire were used as working, reference, and auxiliary electrodes, respectively. The system was controlled by a PC with GPES operating software.
Characterization
X-ray diffraction (XRD) patterns of KCC-1-based materials were recorded on a Siemens D 5000 X-Ray diffractometer (Texas, USA) with a Cu Kα anode (λ = 1.54 Å) operating at 40 kV and 30 mA. TEM analysis was conducted on a Carl Zeiss LEO 906 electron microscope operated at 100 kV (Oberkochen, Germany). Fourier transform infrared (FTIR) spectra were measured using a Shimadzu model FTIR prestige 21 spectrophotometer (Tokyo, Japan) using KBr discs. Brunauer–Emmett–Teller (BET) was recorded on a Micromeritics NOVA 2000 apparatus at 77 K using nitrogen as the adsorption gas (Florida, USA). The surface morphology of the KCC-1, KCC-1-NH2, and KCC-1-NH2-Cys modified GCE electrodes and the porous nanoparticles and energy dispersive X-ray (EDX) spectroscopy were evaluated with a FESEM analysis, which was conducted on TESCAN system of FEG-SEM MIRA3 TESCAN (Brno, Czech Republic). The particle size distribution and zeta potential values were determined using Malvern particle size analyzer (Malvern, UK).
Synthesis
Preparation of KCC-1 and KCC-1-NH2, KCC-1-NH2-Cys
KCC-1 was synthesized according to the methods which was reported by Bayal and coworkers [Citation27]. Briefly, 1 g CTAB was added to 10 cc distilled water and after addition of 0.6 g urea, the mixture was stirred for about 3 h at room temperature. Then, the mixture of 2 g TEOS, 30 cc cyclohexane, and 1.5 cc hexanol was added to the flask and sonicated for 30 min. Afterwards, the mixture was refluxed at 120 °C for 4 h and subsequently refluxed at 80 °C for 24 h. Next, the mixture was cooled to room temperature and centrifuged to collect the KCC-1 as white sediment. The collected KCC-1 was washed several times with water and ethanol and dried at 60 °C for 24 h. Finally, the as-synthesized KCC-1 was calcinated at 550 °C for 6 h to remove the CTAB as templating agent. As mechanism of the KCC-1 synthesis, urea was used to hydrolyze the TEOS to produce negatively charged (SiO4)−4 silicate. Using of CTAB induce the silicate molecules to form self-assembled linear structures where the CTAB help to the aggregating of the silicates [Citation14,Citation28].
To functionalize the KCC-1 surface with NH2 moieties, 0.02 g of KCC-1 was dispersed on 1.2 mL dried toluene and sonicated for 30 min. Then 50 μL 3-aminopropyltriethoxysilane (APTES) was added to the mixture and refluxed for 20 h at 80 °C. Then the mixture was separated and washed with toluene several times and dried at 80 °C for at least 24 h. Finally, Cys was conjugated to the dendritic fibers of KCC-1-NH2 by EDC/NHS chemistry which provides the attaching of the Cys molecules at room temperature. Scheme 1 summarized all of the synthesizing procedures (Scheme 1).
Characterization of KCC-1, KCC-1-NH2, and KCC-1-NH2-Cys
TEM was used for the further characterization of the KCC-1, KCC-1-NH2, and KCC-1- NH2-Cys (). The TEM images revealed the fibrous, porous, and dendritic shape of the nanomaterials, of which the fibrous form is the result of using the CTAB for templating. Also, the particle sizes of KCC-1, KCC-1-NH2, and KCC-1-NH2-Cys were about 167, 188, and 216 nm, respectively.
Supporting information Figure S1 (see supporting information), show the morphological features of the KCC-1, KCC-1-NH2, and KCC-1-NH2-Cys using FE-SEM. The fibrous-sphere indicates that the formation of KCC-1-based materials, which the functionalization have not any effect on the morphology of the KCC-1. EDX results reveal the atomic composition of the KCC-based materials where the KCC-1 is composed only with O and Si. However, the carbon is arising from the FE-SEM grid and the templating agent (Supporting information Figure S2 (see supporting information)). Upon functionalization with APTES, the weight percent of N, O, and C are increased which implies the effective surface modification of KCC-1 with APTES.
FTIR was applied to approve the proper functionalization of the KCC-1 with –NH2. As shown in Supporting information Figure S3 (see supporting information), the characteristic peaks of the silica-based materials could be observed in the range of 1020 to 1110 cm−1 representing the Si—O—Si asymmetric stretching while a Si—OH peak is observed at 960 cm−1, which represents the stretching vibration and asymmetric bending.
The BET and BJH analyses of the KCC-1, KCC-1-NH2, and KCC-1-NH2-Cys were used to evaluate the porous nature of the nanoparticles. The specific surface area and porosity of the materials were determined using the adsorption isotherm and calculated by BET. BJH method was used to determine the pore volume of the KCC-1, KCC-1-NH2, and KCC-1-NH2-Cys. Supporting information Table S1 (see supporting information) lists the average pore size, surface area, and pore volume of KCC-1, KCC-1-NH2, and KCC-1-NH2-Cys. The BJH pore volumes were changed from 1.52 to 1.1 and 2.2 cm3/g for KCC-1, KCC-1-NH2, and KCC-1-NH2-Cys, respectively, where the surface area of KCC-1 changed from 617 m2/g to 367 and 205 m2/g for KCC-1-NH2, and KCC-1-NH2-Cys, respectively. Mean pore diameter distribution of the materials were 9.9, 11.9, and 6.09 for KCC-1, KCC-1-NH2, and KCC-1-NH2-Cys, respectively (Supporting information Figure S4 (see supporting information)). The pore size, pore volumes, and surface area of KCC-1, KCC-1-NH2, and KCC-1-NH2-Cys are obviously approved by the reported results [Citation27,Citation29].
Zeta potentials of KCC-1, KCC-1-NH2, and KCC-1-NH2-Cys were checked at pH 7.5 to determine the surface charge to identify the possible surface modification. The KCC-1 bare fibrous nanomaterials display negative charge at pH 7.5 which could be the result of Si-OH functional groups. However, the zeta potential of the KCC-1-NH2, and KCC-1-NH2-Cys show positive charges which confirms the anchoring amine and -SH groups on the surface of the fibrous materials, respective. DLS results of the KCC-1, KCC-1-NH2, and KCC-1-NH2-Cys also show an increase in the hydrodynamic diameter of the nanomaterials from KCC-1 to KCC-1-NH2-Cys which supports again the surface functionalization with NH2 and FA groups. The XRD patterns of KCC-1, KCC-1-NH2, and KCC-1-NH2-Cys were performed from 3.0° (2θ) to 70.0° (2θ) (Supporting information Figure S5 (see supporting information)) to test the crystallinity of the produced KCC-1-based nanomaterials where two major peaks could be observed, indicating that the crystallinity increased from KCC-1 to KCC-1-NH2-Cys. The broad peak at 20° and 30° is assigned to the amorphous silica [Citation30]. Also, compared to the KCC-1, the KCC-1-NH2 and KCC-1-NH2-Cys patterns were shifted to the higher 2θ values which are again an indication of the surface functionalization of KCC-1.
Results and discussion
For the investigation of functionalized DNFS adsorbent capacity for the removal of metal ions, some electrochemical techniques such as cyclic voltammetry (CV), linear sweep voltammetry (LSV), and differential pulse voltammetry (DPV) were used. For this purpose, 0.02 g of KCC-1-NH2-Cys was incubated with some of the metal ions. Then CVs, LSV, and DPVs of Cd2+, Cu2+, Ag+, and Pb2+ ions were recorded before and after incubation with synthesized adsorbent (Cys-DFNS-NH2) using GCE as working electrode.
As can be seen in , the peak current of the GCE was reduced after incubation of Cd2+ with synthesized adsorbent in the 25 °C. It is found that the peak location of CVs, LSV, and DPVs was about –0.9 V. Also, it is found that the redox peak potentials of Cd2+ appeared at –0.9 V vs. Ag/AgCl () before incubation with Cys-DFNS-NH2 using CV technique and GCE as working electrode. Interestingly, after incubation of this metal ion with a concentration of 2.5 mM with synthesized absorbent, the oxidation peak potential was reduced and reached –0.83 V vs. Ag/AgCl. These results were confirmed by other techniques (DPV and LSV) which are shown in . Therefore, Cd2+ was strongly captured by Cys-DFNS-NH2. In other words, Cys-DFNS-NH2 has excellent capacity for entrapment of Cd2+ ions and its efficient removal from aqueous solution.
Figure 2. (A-C) CVs, DPVs, and LSVs of GCE in the presence of Cd2+ (2.5 mM) before and after incubation with KCC-1-NH2-Cys (0.02 g). Sweep rate of CV is 50 mV/s. Sweep rate of CV is 100 mV/s. Sweep rate of LSV is 100 mV/s. Modulation time = 0.05, Interval time = 0.25, Modulation amplitude= 0.025.
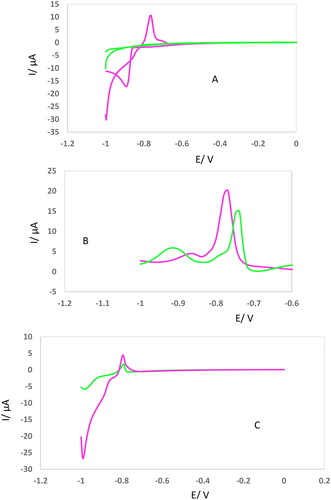
Figure 3. (AC) CVs, DPVs, and LSVs of GCE in the presence of Cd2+ (2.5 mM) after incubation with KCC-1-NH2-Cys (0.02 g) in different incubation time (15, 30, 45, 60 min). All of conditions and parameters are similar to .
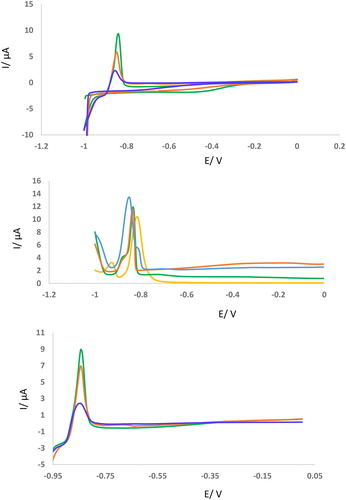
The second approach is based on the idea of interaction of Cys-DFNS-NH2 and Cd2+ as an example of electroactive metal ions at different incubation times. Correlated electrochemical responses shown in indicate that by increasing the time of incubation between adsorbent and Cd2+, the electrochemical peak currents also decreased. This demonstrates that as incubation time increases, the interaction between Cys-DFNS-NH2 and Pb2+ gets tighter and bolstered. Nevertheless, the influence of the incubation time on the electrochemical measurements of Cd2+ could not be extended for other ions.
Combining two approaches, it can be concluded that the interaction of Cys-DFNS-NH2 and Cd2+ can be verified via electrochemical procedures while also the strength of the interaction can be checked through electrochemical techniques.
Scheme 2 shows the removal mechanism of Pb2+ KCC-1-NH2-Cys as a model.
Incubation time of Cd2+ with synthesized adsorbent was evaluated by CV, DPV, and LSV, techniques. For this purpose, 0.02 g of KCC-1-NH2-Cys was incubated with 2.5 mM of Cd2+ ions at vigoros stirring for different times (15, 30, 45, 60 min). Then, the homogeneous solution was centrifuged. At the final step, super-naturated solution was collected and above mentioned voltammograms were recorded. As can be seen in , different incubation times lead to a 4 fold decrease in peak current. As can be seen, after 60 min incubation of Cd2+ with 0.02 g of KCC-1-NH2-Cys, the peak current reaches about 1 µA. According to the obtained results, it is found that the candidate adsorbent saturated by 2.5 mM of Cd2+. On the other hand, a change of peak potential of GCE after incubation at various times (15–60 min) confirmed the excellent interaction of the suggested adsorbent with this heavy metal ion. These results were confirmed by other techniques (DPV and LSV) which are shown in .
Finally, we need to investigate the concentration profile of the adsorbent using a sensitive technique. For this purpose, DPVs of GCE were recorded for different concentrations of metal ions incubated with KCC-1-NH2-Cys (). As can be seen, the peak current of GCE was decreased with decrement of Cd2+ concentration. According to the obtained results, a linear relation was obtained between peak current of GCE and Cd2+ concentration using the DPV technique.
Figure 4. DPVs of GCE in the presence of different concentration of Cd2+ (2.5, 5, 7.5, 10, 20 mM) after incubation with KCC-1-NH2-Cys (0.02 g). Modulation time = 0.05, Interval time = 0.25, Modulation amplitude= 0.025.
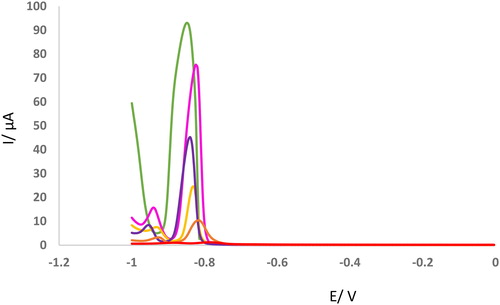
Similar investigations were applied for the removal of Pb2+ ions from aqueous solution using KCC-1-NH2-Cys. In contrast to Cd2+, the oxidation potential of Pb2+ ions appeared at –0.65 V vs. Ag/AgCl and few changes in oxidation potential were observed after incubation with KCC-1-NH2-Cys. However, similar to Pb2+ ions, peak currents were decreased after incubation of the Pb2+ with KCC-1-NH2-Cys. Therefore, this adsorbent can be used for the removal of Pb2+ at the potential of –0.65 V vs. Ag/AgCl (Supporting information Figure S6 (see supporting information)).
CV technique was used for the evaluation and optimization of incubation time of Pb2+ with KCC-1-NH2-Cys. For this purpose 2.5 mM of Pb2+ was incubated with KCC-1-NH2-Cys in different times (15, 30, 45 min) and DPVs, LSVs were recorded. As can be seen in Supporting information Figure S7 (see supporting information), an increment of incubation time lead to a decrement of peak current while peak potential was not changed. These results show that, KCC-1-NH2-Cys has excellent capacity for the removal of Pb2+ from aqueous solution.
Finally, we need to investigate the concentration profile of the adsorbent using a sensitive technique. For this purpose, DPVs of GCE were recorded in different concentrations of metal ions incubated with KCC-1-NH2-Cys (Supporting information Figure S8 (see supporting information)). As can be seen, peak current of the GCE were decreased with decrement of metal ion concentration. According to obtained results, a linear relation was obtained between peak current of GCE and Pb2+ concentration using DPV technique.
CV, DPV, and LSV techniques were applied for the investigation of Ag+ ions removal from aqueous solution using KCC-1-NH2-Cys. Interestingly, the oxidation potential of Ag+ ions appeared in the more positive potentials (0.1 V vs. Ag/AgCl) and few changes in oxidation potential were observed after incubation with KCC-1-NH2-Cys. But, similar to Pb2+ and Cd2+ ions, peak currents were decreased after incubation of the Ag + with KCC-1-NH2-Cys. Therefore, this adsorbent can be used to removal of Ag+ from aqueous solution in the potential of +0.1 V vs. Ag/AgCl ((Supporting information Figure S9 (see supporting information)).
The effect of incubation time of Ag+ (2.5 mM) on the efficient removal of this ion by KCC-1-NH2-Cys was investigated using CV, DPV, and LSV techniques. As can be seen in (Supporting information Figure S10 (see supporting information)), increment of incubation time lead to decrement of peak current while peak potential was not changed approximately. These results show that, KCC-1-NH2-Cys has excellent capacity for the removal of Ag+ from aqueous solution. Also, surface of KCC-1-NH2-Cys was saturated by 2.5 mM of Ag+ after 60 min.
Similar to previous parts, the concentration profile of the candidate adsorbent for the removal of Ag+ was investigated by a sensitive technique (DPV). For this purpose, DPVs of GCE were recorded in different concentrations of metal ions incubated with KCC-1-NH2-Cys (Supporting information Figure S11 (see supporting information)). As can be seen, peak current of the GCE were decreased with decrement of metal ion concentration. According to obtained results, a linear relation was obtained between peak current of GCE and Ag+ concentration using DPV technique. This approach related to high affinity of Ag + to –SH functionalized group of candidate adsorbent. Another idea is high and strong interaction of Ag + with –NH and –SH groups which lead to saturation of proposed adsorbent by this ion.
Interestingly, in the case of Cu2+ ion, different behavior was observed. At the first part and in contrast to previous ions (Cd2+, Pb2+, and Ag +), peak current of CVs, LSVs, and DPVs were not changed after incubation of Cu2+ with KCC-1-NH2-Cys (Supporting information Figure S12 (see supporting information)). Also, the effect of incubation time on the performance of adsorbent show new and different results. As can be seen in Supporting information Figure S13 (see supporting information), with increment of incubation times (15 and 60 min), peak current was not changed. These results indicated that, DNFS was not sensitive for this ion.
Finally, it is found that KCC-1-NH2-Cys is very effective in removing candidate ions from ground natural water with low ions concentrations as most cases in the natural environment. The method was also applied to the analysis of local natural ground water samples. gives the possibility for accurate analysis of low concentration of Pb2+, Cd2+, and Ag+, and Cu2+ in natural ground water and river samples by electrochemical methods and based on the proposed adsorbent.
Table 1. Amount of ions in river-water and local natural ground water samples analyzed by proposed method.
Conclusion
In summary, KCC-1-NH2-Cys was found to be a new and efficient adsorbent for the removal of some ions (Pb2+, Cd2+, and Ag+) from water samples. Also, it showed high efficiency in the removal of selected cations from aqueous samples to improve the quality of water samples. The results show that Cys-DFNS-NH2 can selectively adsorb Pb2+, Cd2+, and Ag+ in different potentials. This work introduced novel methods to prepare Cys functionalized DFNS-NH2 with high specific surface area and total pore volumes, which have the potential to be applied as an efficient adsorbent for specific metal ions. In addition, KCC-1-NH2-Cys can be potentially employed as an excellent adsorbent in large-scale plants for contaminated water treatment due to their easy, efficient separation and high adsorption capacity.
Notes on contributors
Nilufar Khantan is an MSc student at Urmia University. Recently, she focused on the synthesis of dendritic nano-silca materials and its application in separation science.
Nasrin Shadjou received his Ph.D. in organic chemistry from K.N. Toosi University of Technology, Tehran, Iran. She currently is an assistant professor of Nanochemistry at Department of Nanochemistry, Nano Technology Research Center, Urmia University. She is a Researcher with over 151 publications and research interests in synthesize of advanced nanomaterials. Her research interests include the preparation of metal doped silica mesoporous and graphene quantum dot materials in particular smart silica materials, and their applications in health science and drug/biomarkers analysis.
Mohammad Hasanzadeh is an assistant professor of pharmaceutical analysis at Tabriz University of Medical Sciences (TUOMS). In 2010, he founded Drug Applied Research Center and worked at that center as the PhD candidate for 4 years. Dr. Hasanzadeh has also received International Razi Award for excellence research in health sciences in 2010. Recently, he has focused on the application of advanced nanomaterials towards biomedical science, biosensing and tissue engineering.
Supplemental Material
Download MS Word (3.3 MB)Acknowledgment
We gratefully acknowledge the support of this work by Urumia University and Tabriz University of Medical Sciences.
Disclosure statement
There is no conflict of interest.
References
- Yavuz CT, Mayo JT, Yu WW, et al. Low-field magnetic separation of monodisperse Fe3O4 nanocrystals. Science. 2006;314(5801):964.
- Oliveira LCA, Petkowicz DI, Smaniotto A, et al. Magnetic zeolites: a new adsorbent for removal of metallic contaminants from water. Water Res. 2004;38(17):3699.
- Yantasee W, Warner CL, Sangvanich T, et al. Removal of heavy metals from aqueous systems with thiol functionalized superparamagnetic nanoparticles. Environ Sci Technol. 2007;41(14):5114.
- Orbell JD, Godhino L, Bigger SW, et al. Oil spill remediation using magnetic particles: an experiment in environmental technology. J Chem Educ. 1997;74(12):1446.
- Safarik I, Safarikova M, Buricova V. Sorption of water soluble organic dyes on magnetic poly(oxy-2,6-dimethyl-1,4-phenylene). Collect Czech Chem Commun. 1995; 60:1448.
- Oliveira LCA, Rios RVRA, Fabris JD, et al. Activated carbon/iron oxide magnetic composites for the adsorption of contaminants in water. Carbon. 2002;40(12):2177.
- Wu Z, Zhao D. Ordered mesoporous materials as adsorbents. Chem Commun (Camb). 2011;47(12):3332–3338.
- Akin I, Arslan G, Tor A, et al. Arsenic(V) removal from underground water by magnetic nanoparticles synthesized from waste red mud. J Hazard Mater. 2012;235–236:62–68.
- Marin P, Borba CE, Módenes AN, et al. Determination of the mass transfer limiting step of dye adsorption onto commercial adsorbent by using mathematical models. Environ Technol. 2014;35(18):2356–2364.
- Vistuba JP, Nagel-Hassemer ME, Lapolli FR, et al. Simultaneous adsorption of iron and manganese from aqueous solutions employing an adsorbent coal. Environ Technol. 2013;34(2):275–282.
- He S, Zhang D, Gu L, et al. Bromate adsorption using Fe-pillared bentonite. Environ Technol. 2012;33(20):2337–2344.
- Jaafarzadeh N, Amiri H, Ahmadi M. Factorial experimental design application in modification of volcanic ash as a natural adsorbent with Fenton process for arsenic removal. Environ. Technol. 2012;33(2):159–165.
- RaúlPedroza FC, Aguilara M, Martínez-Luevanosb A, et al. Blast furnace (BF) residues for arsenic removal from mining-contaminated groundwater. Environ Technol. 2014;35(23):2895–2902.
- Polshettiwar V, Cha D, Zhang X, et al. High-surface-area silica nanospheres (KCC-1) with a fibrous morphology. Angew Chem Int Ed Engl. 2010;49(50):9652–9656.
- Polshettiwar V, Basset JM. High surface area fibrous silica nanoparticles. US20110253643. 2010.
- Huang W, Yu X, Tang J, et al. Enhanced adsorption of phosphate by flower-like mesoporous silica spheres loaded with lanthanum. Microporous Mesoporous Mater. 2015; 217:225–232.
- Xie Y, Wang J, Wang M, et al. Fabrication of fibrous amidoxime-functionalized mesoporous silica microsphere and it’s selectively adsorption property for Pb2+ in aqueous solution. J Hazard Mater. 2015; 297:66–73.
- Sun Z, Li H, Guo D, et al. A multifunctional magnetic core–shell fibrous silica sensing probe for highly sensitive detection and removal of Zn2+ from aqueous solution. J Mater Chem C. 2015;3(18):4713–4722.
- Du X, He JH. Amino-functionalized silica nanoparticles with center-radially hierarchical mesopores as ideal catalyst carriers. Nanoscale. 2012;4(3):852–859.
- Zhao GX, Li JX, Ren XM, et al. Few-layered graphene oxide nanosheets as superior sorbents for heavy metal ion pollution management. Environ Sci Technol. 2011;45(24):10454–10462.
- Hakami O, Zhang Y, Banks CJ. Thiol-functionalised mesoporous silica-coated magnetite nanoparticles for high efficiency removal and recovery of Hg from water. Water Res. 2012;46(12):3913–3922.
- Zhang S, Shu XW, Zhou Y, et al. Highly efficient removal of uranium(VI) from aqueous solutions using poly(acrylic acid)-functionalized microspheres. Chem Eng J. 2014;253:55–62.
- Stein A, Melde BJ, Schroden RC. Hybrid inorganic-organic mesoporous silicates—nanoscopic reactors coming of age. Adv Mater. 2000;12(19):1403.
- Yoshitake H, Yokoi T, Tatsumi T. Adsorption of chromate and arsenate by amino-functionalized MCM-41 and SBA-1. Chem Mater. 2002;14(11):4603.
- Hamoudi S, Saad R, Belkacemi K. Adsorptive removal of phosphate and nitrate anions from aqueous solutions using ammonium-functionalized mesoporous silica. Ind Eng Chem Res. 2007;46(25):8806.
- Hasanzadeh M, Farajbakhsh F, Shadjou N, et al. Mesoporous (organo) silica decorated with magnetic nanoparticles as a reusable nanoadsorbent for arsenic removal from water samples. Environ Technol. 2015;36(1):36–44.
- Bayal N, Singh B, Singh R, et al. Size and fiber density controlled synthesis of fibrous nanosilica spheres (KCC-1). Sci Rep. 2016;6:24888.
- AbouAitah KEA, Farghali AA, Swiderska-Sroda A, et al. Mesoporous silica materials in drug delivery system: pH/glutathione-responsive release of poorly water-soluble pro-drug quercetin from two and three-dimensional pore-structure nanoparticles. J Nanomed Nanotechnol. 2016;7:1–12.
- Ge S, Zhang Y, Zhang L, et al. Ultrasensitive electrochemical cancer cells sensor based on trimetallic dendritic Au@PtPd nanoparticles for signal amplification on lab-on-paper device. Sens Actuators B Chem. 2015;220:665–672.
- Sadeghzadeh SM. Ionic liquid immobilized onto fibrous nano-silica: A highly active and reusable catalyst for the synthesis of quinazoline-2,4(1H,3H)-diones. Catal Commun. 2015;72:91–96.