Abstract
Graphene has excellent properties which can be a promising material as nanocomposites for application in a drug delivery system (DDS). In this study, reduced graphene oxide/silver nanoparticles (rGO/AgNPs) nanocomposites was synthesized using a facile in-situ method for drug loading application of acetylsalicylic acid (ASA). The synthesis results were characterized by FESEM, EDX, UV-Vis, XRD, and Raman spectrophotometers analyses. The results showed that rGO/AgNPs nanocomposites was synthesized successfully. Drug loading application was performed using UV-Vis measurements. The loading results depicted that rGO/AgNPs is successfully created in high-capacity loading for ASA. Drug loading performances enhanced with the increasing loading time of ASA on rGO/AgNPs. The optimum percentage of drug loading capacity for ASA by rGO/AgNPs was ∼83% in a contact time of 30 hrs.
Graphical Abstract
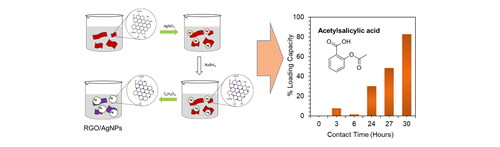
1. Introduction
The emergence of nanotechnology over the last few decades has attracted the research interest of scientists on nanomaterial development [Citation1]. Among the nanomaterials, graphene and its derivatives have emerged as distinctive materials due to their unique structure and excellent properties such as high electron mobility, high electrical conductivity and excellent mechanical stiffness [Citation2]. Graphene is the single layer of graphite in the form of a two-dimensional enclosed hexagonal honeycomb lattice with sp2 hybridization. Due to its large 2 D surface area and those excellent properties, graphene has been considered as an attractive matrix of nanocomposites for the integration with the metal nanoparticles (NPs) in realizing further novel and superior properties for various specific applications such as in fuel cells, energy storage, catalysts, sensors and biomedical applications [Citation3–5]. Among the metal nanoparticles, silver nanoparticles (AgNPs) are one of the most extensively utilized nanomaterials due to their antibacterial capability, catalytic activity, large specific surface area, small particle diameter, fast electron transfer ability, optical and photothermal properties [Citation6, Citation7].
Acetylsalicylic acid (ASA; Aspirin) is a common anti-inflammatory drug widely prescribed as an anti-platelet to prevent cardiovascular events [Citation8]. It is categorized as Class II drugs according to biopharmaceutics classification systems (BCS) which have poor solubility and bioavailability [Citation9]. The prolonged use of ASA is associated with gastrointestinal mucosa ulcers and gastrointestinal hemorrhaging in severe cases [Citation8]. Here in the present work, the reduced Graphene Oxide/Silver Nanoparticles (rGO/AgNPs) nanocomposite was synthesized by in-situ method and applied as drug loading for ASA. Hybrid materials optimized with nanotechnology synthesized via in-situ method from rGO by decorating AgNPs for a drug delivery system are quite efficient labor and cost. Besides that, the nanocomposites can be applied in the biomedical application with several benefits, including biocompatible carbon materials as a drug delivery system (DDS); delivery of drug molecules in nanoscale sizes which provide targeted delivery of optimal dose with reduced side effects and toxicity [Citation10]. Moreover, nanocarriers can solve drug solubility and bioavailability problems, thus increasing drug loading on the surface [Citation9].
2. Experimental method
2.1. Materials
Graphite powder, sulfuric acid (H2SO4), sodium nitrate (NaNO3), potassium permanganate (KMnO4), hydrogen peroxide (H2O2), hydrochloric acid (HCl), sodium borohydride (NaBH4) and silver nitrate (AgNO3) were all purchased from Merck.
2.2. In-situ synthesis of rGO/AgNPs
The rGO/AgNPs nanocomposites were prepared by in-situ synthesis method. Firstly, GO was synthesized from natural graphite via a modified Hummers' method [Citation11, Citation12]. Synthesis of rGO/AgNPs nanocomposite in-situ was carried out by adding 500 mg of GO to a 50 mL ethanol and 50 mL distilled water followed by a 30 min sonication process. Then the 15 mL of AgNO3 0.1 M were added, followed by a sonication process for 30 min. Stirring was carried out for 30 min, then added 25% NH3 slowly until the pH was close to 10, then stirred again for 30 min. After that, 30 mL NaBH4 0.1 M was added, followed by the reflux process for 18 hrs at a constant temperature of 150 °C. The dilution process with 100 mL of distilled water is continued with filtering and washing by centrifugation for 15 min. Finally, the drying process was carried out for 24 hrs at 80 °C in the oven.
2.3. Characterization of GO and rGO/AgNPs
The maximum wavelengths of GO and rGO/AgNPs were characterized by UV-Vis spectrophotometer (UV-Vis, LABTRON LUS B-12). The crystal structure characterization of GO and rGO/AgNPs composites was carried out using X-ray Diffraction (XRD, Smartlab Rigaku X-ray diffraction) with Cu-Kα radiation (λ = 1.5406 Å). Surface morphology and analysis were characterized by Field Emission Scanning Electron Microscopy-Energy Dispersive X-ray Spectroscopy (FESEM JIB-4610F, EDX Oxford Instruments XMaxN), the nanostructure of rGO/AgNP was characterized by Transmission Electron Microscope (TEM, TECNAI G220 S-TWIN). The vibration spectra of GO and rGO/AgNPs was performed using Raman spectroscopy (Labspec 6-HORIBA Scientific Type iHR320).
2.4. Loading ASA in rGO/AgNPs nanocomposite material
The loading of ASA drug in rGO/AgNPs material begins with preparing a standard solution of ASA. Then the drug loading was done with a comparison ratio between rGO/AgNPs and drug at 2:1 with distilled water and stirred at room temperature and then measured its absorbance with UV-Vis spectrophotometer at time variations of 0, 3, 6, 24, 27 and 30 hrs.
3. Result and discussion
3.1. Morphology and structure characterization of rGO/AgNPs by FESEM-EDX and TEM
The morphology and structure characterization of GO and rGO/AgNPs were analyzed by FESEM and TEM. This analysis of EDX was applied as a support to determine the chemical composition contained in the material. FESEM-EDX analysis was conducted by coating the samples with gold (Au) to enhance the morphology in the characterization. The results of the FESEM-EDX characterization of GO and rGO/AgNPs are shown in . The distinct wrinkled layers of GO are obviously observed, as seen in . The EDX analysis result shows that the elemental analysis of the GO contains C and O elements (). The AgNPs distributed on the rGO surface are clearly observed in . The elemental analysis of rGO/AgNPs confirmed that 16.8% of AgNPs are observed in a spherical shape and bright image of silver nanoparticles distributed on the rGO surface (). The EDX analysis proves that the rGO/AgNPs nanocomposites have been successfully formed, marked by the presence of the carbon (C) and oxygen (O) elements from rGO and the Ag element from AgNPs. Furthermore, the reduction of O element detected on the EDX spectrum of rGO/AgNPs compared with GO confirmed that reduced graphene oxide is formed. The TEM image of rGO/AgNPs nanocomposites is shown in . The light-gray sheet of rGO is clearly seen with the black circle particles of AgNPs distributed on rGO sheet. The particles size of AgNPs decorated on rGO sheet is calculated by ImageJ with the particles size around 11 nm ().
3.2. XRD, UV-Vis and Raman spectroscopy analyses of GO and rGO/AgNPs nanocomposites
The optical properties of GO and rGO/AgNPs nanocomposites are shown in . In the UV-Vis spectrum, the main peak at 230 nm and the shoulder peak at 300 nm indicates π–π* transition from the C = C bond and the n–π* transition from the C = O bond, respectively [Citation13]. Red-shifting absorbance peaks occur up to 260 nm for rGO/AgNPs, indicating the recovery of electronic conjugations of GO sheets due to reduction of GO to form rGO [Citation14]. Additionally, the absorption peak was 420 nm indicating the formation of AgNPs [Citation6]. The optical properties prove that the rGO/AgNPs nanocomposites has been synthesized successfully.
Figure 2. (a) UV-Vis analysis of GO and rGO/AgNPs. (b) XRD analysis of GO and rGO/AgNPs nanocomposite. (c) Raman spectra of GO and rGO/AgNPs nanocomposites.
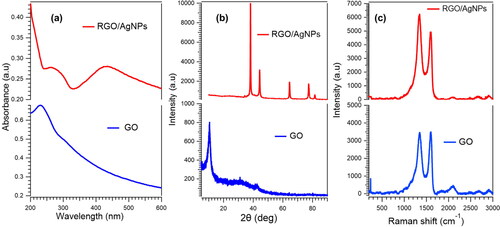
The results of diffraction characterization using the XRD instrument in showed the diffraction patterns of GO and rGO/AgNPs. The peak of GO is very sharp with reciprocal carbon atom (001) at 10.5°. It is obvious that the peak of GO is not observable due to the reduction of GO to be rGO and attachment of silver nanoparticles on rGO sheet. The absence of the weak and broad diffraction peak of rGO at ∼25° depicts that AgNPs are attached to the surface of the rGO sheets, avoiding the stacking of rGO sheets [Citation15]. The diffraction peaks appear at 38.16°, 44.31°, 64.43°, and 77.40°, corresponding to the (111), (200), (220), and (311) diffraction peaks of face-centered cubic silver (corresponding to PDF2-2004 card) [Citation16, Citation17].
The Raman spectra of GO and rGO/AgNPs are depicted in . The D band is ascribed to the sp3 carbon of defective sites and the G band is due to the plane vibrations with E2g phonon. GO exhibits D and G bands at 1348 and 1596 cm−1, respectively, whereas the D and G bands of the rGO/AgNPs nanocomposite appear at 1350 and 1598 cm−1, respectively. The ID/IG ratio for GO is ∼0.99 and for rGO/AgNPs is ∼1.22. The ID/IG of rGO/AgNPs nanocomposites increases due to the decoration of AgNPs on rGO, which is attributed to the surface-enhanced Raman scattering (SERS) of AgNPs [Citation18].
3.3. Drug loading performance of rGO/AgNPs for ASA
The peak of ASA standard shows the absorbance at the wavelength ∼270 nm (). It can be seen that after stirring time variations are applied, the peak is shifted slightly to a higher wavelength due to interaction rGO/AgNPs in the loading process of ASA (). The longer loading process until 30 hrs, the ASA drug being optimally loaded into the rGO/AgNPs nanocomposite (), which indicates that the ASA is effectively loaded in the nanocomposite by sticking to the surface of the rGO, which contains AgNPs active ions.
Figure 3. (a) Standard solution of ASA 200 ppm. (b) Relationship between wavelength vs. absorbance of ASA loading on rGO/AgNPs at 3, 6, 24, 27 and 30 hrs. (c) Relationship between Contact time vs. absorbance at 3, 6, 24, 27 and 30 hrs. (d) Percentage of loading capacity of ASA at 3, 6, 24, 27 and 30 hrs. (e) Proposed drug loading mechanism of ASA by rGO/AgNPs.
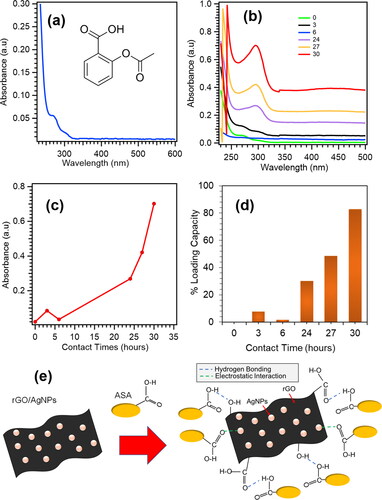
The percentage of drug loading for ASA by rGO/AgNPs is shown in . The result reveals that rGO/AgNPs nanocomposites are effective as DDS of ASA with the highest loading capacity achieve ∼83% at 30 hrs. Compared to the previous study of drug loading for ASA, the result of drug loading capacity of the current method is much higher. In the previous method, ASA was loaded into polymeric matrices of Poly(L-acid lactic) (PLLA) and linear low-density polyethylene (LLDPE) with supercritical CO2 impregnation which showed drug loading capacity ∼5% and ∼2%, respectively [Citation8]. The proposed drug loading mechanism of ASA by rGO/AgNPs is shown in . The ASA molecules are bound to the surface of rGO/AgNPs nanoparticles via intramolecular hydrogen bonding with residual oxygen functional groups of rGO sheet. In addition, the drugs interact by electrostatic interaction with silver nanoparticles as well [Citation19]. The hydrogen bonding and electrostatic interaction give rise to high drug loading and stability of rGO/AgNPs as DDS for ASA. The AgNPs particles on rGO sheet are still stable after the drug loading which is confimed from SEM morphology and EDS mapping of the elements (Figure S1(d), Supplementary Materials). The AgNPs are still uniformly distributed on RGO sheet.
4. Conclusion
The rGO/AgNPs nanocomposites was successfully synthesized by in situ method. The results were confirmed by FESEM, EDX, UV-Vis, XRD, and Raman spectrophotometers analyses. The rGO/AgNPs obtained are applied for acetylsalicylic acid's loading drug (ASA). The results show that the ASA loading on rGO/AgNPs obtained stable and high loading capacity. Furthermore, the performance of the rGO/AgNPs nanocomposite enhanced along with the increasing drug loading time, revealing that rGO/AgNPs are very potential applications as DDS.
Supplemental Material
Download MS Word (1.8 MB)Acknowledgements
We acknowledge sophisticated analytical instrumentation facilities from ELSA (E-Layanan Sains-BRIN) for the characterization of the materials.
Disclosure statement
No potential conflict of interest was reported by the authors.
Additional information
Funding
Notes on contributors
Murni Handayani
Murni Handayani received the B.Sc. degree from the Chemistry Department, Sebelas Maret University, Indonesia, in 2003. She received M.Sc. degree and the Ph.D. degree in Chemistry Department from the Osaka University, Japan, in 2013 and 2016, respectively, under supervision of Prof. Takuji Ogawa. After her doctoral graduation in 2017, she was a specially Assigned Assistant Professor in Chemistry Department, Osaka University, Japan. She was a visiting scientist in Nanyang Technological University (NTU), Singapore and TU Braunschweig, Germany in 2019. From 2005-2021 she was a researcher in Research Center for Metallurgy and Materials, the Indonesian Institute of Sciences (LIPI). In 2021 the Indonesian Institute of Sciences merged into the National Research and Innovation Agency (BRIN). She is currently a researcher in the Research Center for Advanced Materials, National Research and Innovation Agency (BRIN). Her current research interests include nanoscience, materials chemistry, molecular architectonics, nanocomposites and nanomaterials, especially nanocarbons (graphene and carbon nanotubes).
Bagus Indra Suwaji
Bagus Indra Suwaji received a B.Sc. degree from the Department of Chemistry, Sebelas Maret University, Indonesia, in 2021. His research interest is in copolymer as adsorbent and nanomedicine, especially drug delivery system.
Geolita Ihsantia Ning Asih
Geolita Ihsantia Ning Asih received a B.Sc. degree from the Department of Chemistry, Sebelas Maret University, Indonesia, in 2021. Currently, she is research assistant in Bandung Institute of Technology, Indonesia. Her research interest is in nanomaterials and nanomedicine, especially drug delivery system.
Triana Kusumaningsih
Triana Kusumaningsih received the B.Sc. degree, M.Sc. degree and the Ph.D. degree in Chemistry Department from Universitas Gadjah Mada, Indonesia, in 1997, 2000 and 2012, respectively. She is currently a lecturer in the Chemistry Department, Sebelas Maret University. Her current research interests include food chemistry and organic chemistry, especially Organic synthesis.
Yuni Kusumastuti
Yuni Kusumastuti is a lecturer in the Chemical Engineering Department of Universitas Gadjah Mada. She received her bachelor (2005) and master degree (2008) in Chemical Engineering at Universitas Gadjah Mada. As she continued her education, she obtained her doctoral degree (D.Eng.) from Nara Institute of Science and Technology, Japan. She is currently specializing in both organic and inorganic biomaterial, tissue engineering, functionalized material and bioresource engineering.
Rochmadi
Rochmadi is one of Professors in Chemical Engineering Department at Universitas Gadjah Mada Yogyakarta. He received his Bachelor and Master degree at Universitas Gadjah Mada Yogyakarta in 1980 and 1985. He received his Ph.D. at Chemical Engineering of Imperial College of Science and Technology, United Kingdom in 1991. He has been giving lectures for Chemical Engineering students since 1981. His research focuses on polymer synthesis and degradation, process modelling and simulation and chemical engineering design.
Isa Anshori
Isa Anshori received the B.Eng. degree from the Engineering Physics Department, Bandung Institute of Technology, Indonesia, in 2009, M.Eng. degree in materials science and the Ph.D. degree in nanoscience and nanotechnology both from the University of Tsukuba, Japan, in 2015 and 2018, respectively. He has been employed as an Assistant Professor with the Biomedical Engineering Department, Bandung Institute of Technology since 2018. His current research interests include bio/chemical sensors, microfluidics, the IoT devices, and lab-on-chip.
References
- Kumari S, Sharma P, Yadav S, et al. A novel synthesis of the graphene Oxide-Silver (GO-Ag) nanocomposite for unique physiochemical applications. ACS Omega. 2020;5(10):5041–5047.
- Gurunathan S, Han JW, Park JH, et al. Reduced graphene oxide-silver nanoparticle nanocomposite: a potential anticancer nanotherapy. Int J Nanomed. 2015;10:6257–6276.
- Yoon Y, Samanta K, Lee H, et al. Highly stretchable and conductive silver nanoparticle embedded graphene flake electrode prepared by in situ dual reduction reaction. Sci Rep. 2015;5:1–10.
- Aziz NSA, Azmi MKN, Hashim AM. One-pot green synthesis of highly reduced graphene oxide decorated with silver nanoparticles. J Sex Med. 2017;46(7):1083–1088.
- de Medeiros AMZ, Khan LU, da Silva GH, et al. Graphene oxide-silver nanoparticle hybrid material: an integrated nanosafety study in zebrafish embryos. Ecotoxicol Environ Saf. 2021;209(111776):1–14.
- Zhang X, Shi X, Gautrot JE, et al. Nanoengineered electrospun fibers and their biomedical applications: a review. Nanocomposites. 2021;7(1):1–34.
- Ito H, Sakata M, Hongo C, et al. Cellulose nanofiber nanocomposites with aligned silver nanoparticles. Nanocomposites. 2018;4(4):167–177.
- Coutinho IT, Maia-Obi LP, Champeau M. Aspirin-loaded polymeric films for drug delivery systems: comparison between soaking and supercritical CO2 impregnation. Pharmaceutics. 2021;13(6):824.
- Azeez NA, Saravanan M, Chandar NRK, et al. Enhancing the aspirin loading and release efficiency of silver oxide nanoparticles using oleic acid-based Bio-Surfactant from enteromorpha intestinalis. Appl Organomet Chem. 2020;34:1–16.
- Ivanova N, Gugleva V, Dobreva M, et al. Silver nanoparticles as Multi-Functional drug delivery systems. Nanomedicines. IntechOpen; 2019; p. 72–91. https://doi.org/10.5772/intechopen.80238.
- Febriana E, Handayani M, Susilo DNA, et al. A simple approach of synthesis of graphene oxide from pure graphite: Time stirring duration variation. AIP Conference Proceedings, Vol. 2382; 2021. p. 40006.
- Handayani M, Kepakisan KAA, Anshori I, et al. Graphene oxide based nanocomposite modified screen printed carbon electrode for qualitative cefixime detection. AIP Conference Proceedings, Vol. 2382; 2021. p. 40005.
- Zhang H, Liu W, Yang L, et al. Fabrication of reduced graphene Oxide-Ag nanocomposites and analysis on the interaction with BSA. J. Nanomater. 2019;2019:1–7.
- Wu T, Liu S, Luo Y, et al. Surface plasmon resonance-induced visible light photocatalytic reduction of graphene oxide: using Ag nanoparticles as a plasmonic photocatalyst. Nanoscale. 2011;3(5):2142–2144.
- Dat NM, Linh VNP, Huy LA, et al. Fabrication and antibacterial activity against Pseudomonas aeruginosa and Staphylococcus aureus of silver nanoparticle decorated reduced graphene oxide nanocomposites. Mater Technol. 2019;34(7):369–375.
- Zeng J, Tian X, Song J, et al. Green synthesis of AgNPs/reduced graphene oxide nanocomposites and effect on the electrical performance of electrically conductive adhesives. J Mater Sci Mater Electron. 2016;27(4):3540–3548.
- Anshori I, Nuraviana Rizalputri L, Althof RR, et al. Functionalized multi-walled carbon nanotube/silver nanoparticle (f-MWCNT/AgNP) nanocomposites as non-enzymatic electrochemical biosensors for dopamine detection. Nanocomposites. 2021;7(1):97–108.
- Gurunathan S, Hyun Park J, Choi Y-J, et al. Synthesis of graphene Oxide-Silver nanoparticle nanocomposites: an efficient novel antibacterial agent. CNANO. 2016;12(6):762–773.
- Joshi AS, Singh P, Mijakovic I. Interactions of gold and silver nanoparticles with bacterial biofilms: Molecular interactions behind inhibition and resistance. Int J Mol Sci. 2020;21:1–24.