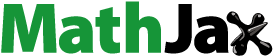
Abstract
In this study, amphiphilic Janus nanoparticles with dumbbell-like structure have been synthesized by connecting two silica nanospheres with differential wettability. In this method, silica nanospheres were modified to be hydrophobic and hydrophilic respectively and then the nanospheres with different wettability were covalently connected together on a one-to-one basis simply by chemical bonds generating between surface groups. The dumbbell-like structure of particles can be observed clearly under scanning electron microscope and transmittance electron microscope. Results showed that 58% of the nanospheres were successfully coupled to build the dumbbell-like structure. Since these anisotropic nanoparticles were based entirely on inorganic materials and owned well-defined surface areas of different wettability, they may have a highly potential for many application fields such as optical and electrical engineering.
Graphical Abstract
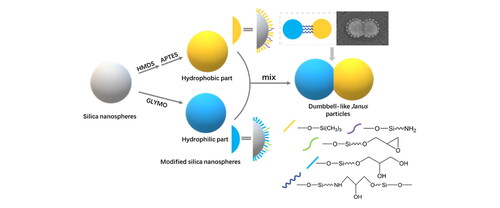
1. Introduction
Janus nanoparticles (JNPs) were named after the Roman God Janus who had two different faces. Consistent with the God Janus, JNPs possess asymmetric structure due to two incompatible areas of different chemistry or morphology. JNPs were firstly made by Casagrande and Veyssie in 1988 [Citation1], and were mentioned by de Genes during his Nobel lecture [Citation2] in 1991 as a new breed of functional materials. Over the past thirty years, JNPs have attracted enormous attention for their peculiar architectures and excellent performances so that the JNPs family expanded rapidly [Citation3] and a variety of Janus structures have come into existence [Citation4], including nanospheres [Citation5, Citation6], nanorods [Citation7], nanodiscs [Citation8], dumbbell-like [Citation9–11], bowl-like [Citation12], crescent-shaped [Citation13], sprout-like [Citation14], and acorn-like [Citation15]. These JNPs have developed diverse potential applications in fields such as Pickering colloidal stabilizers [Citation10], enhanced catalyst [Citation16, Citation17], optical materials [Citation11, Citation18], bio-sensors [Citation19], drug delivery vehicles (especially in cancer therapy) [Citation20].
As a representative category of JNPs, dumbbell-like nanoparticles have attracted much attention because of their typical structure and outstanding performance in many application areas [Citation21]. The dumbbell-like nanoparticles refer to nanoparticles with two spherical lobes which have different chemical properties or morphology. The two connected lobes are relatively independent and synthesis of two parts is also done separately, thus a weaker mutual effect would exist between two areas with different chemical properties and they would be more specifically sectioned comparing with dividing the surfaces of spherical particles into two hemispheres [Citation22]. Previous research [Citation23] has demonstrated that particles with well-defined surface areas of different wettability can be absorbed at oil-water interfaces considerably more strongly than particles of homogeneous wettability. Videlicet, the better-defined between two areas on surfaces, the more thermodynamically stable Pickering emulsions the JNPs would give. As a result, dumbbell-like JNPs may have much better interfacial properties than the spherical ones. In addition, due to the typical structure of dumbbell-like JNPs, different properties and functions can be combined freely by changing the chemicals loaded onto the two lobes, which can provide more possibilities for new species to be synthesized.
In studies on dumbbell-like JNPs reported so far, dumbbell-like JNPs can be divided into three categories, depending on the materials of the two lobes: organic polymer double spheres, polymer-inorganic double spheres and inorganic materials double spheres. dumbbell-like JNPs of different materials can be synthesized in different ways. For organic polymer double spheres, the most common synthesis methods are two-step emulsion polymerization [Citation9, Citation24] and two-step intramolecular cross-linking reactions [Citation25]. For polymer-inorganic double spheres, for example, E. Passas-Lagos et al. [Citation26, Citation27] introduced a technique to fabricated PS/Fe3O4/SiO2 mushroom-shaped nanoparticles: Fe3O4 particles were immobilized by PS at first and then were spatially selectively coated by SiO2. As for inorganic materials double spheres, the most important part in the synthesis process is the multi-step growth of materials. First, synthesize seed particles with material A, and material B was then nucleated on former seeds to form another lobe [Citation28–30]. We are particularly interested in dumbbell-like JNPs based on pure inorganic materials for their excellent performance and wide range of applications, such as amphiphilic coatings [Citation9], photonic crystals [Citation11] and supercapacitors [Citation29]. Thus, we believe that a more convenient synthesis method with good universality should be developed. We selected silica nanospheres as the raw material because of its simple preparation process, easy adjustment of size and structure and variable surface properties [Citation31–33]. Herein, we present a novel and facile method for preparing amphiphilic dumbbell-like Janus nanoparticles by coupling silica nanospheres with different hydrophilicity through chemical bonds. Different from our previous work [Citation34], in this work, monodispersed silica nanospheres with lager size synthesized through Stöber method [Citation31] were modified and different hydrophilicity was given. Then, each pair of hydrophilic and hydrophobic silica nanospheres were connected by silane couplers and finally built a dumbbell-like structure with asymmetric hydrophilicity, the whole process is carried out in ethanol. That is, we could easily fabricate the amphiphilic Janus nanoparticles with dumbbell-like structure by one-step coupling. To better understand the coupling process, we analyzed the proportion of nanospheres participating in the reaction through particle size distribution. Compared with other preparation strategies of Janus nanoparticle, such as “two-step growing method” of most dumbbell-like NPs [Citation28–30], this method is direct and template-free, preparing and removing templates were not needed during the experiment, and enables the preparation of dumbbell-like particles based on the homogeneous inorganic materials. According to the mechanism, this method can also be applied to many kinds of nanospheres with modifiable surfaces.
2. Experimental section
2.1. Chemical agents and materials
Tetraethyl orthosilicate (TEOS, 99.9%) was procured from Acros Organics Inc., America. Ethanol (C2H5OH, 99.5%), ammonia (NH3·H2O, 28%) and 3-Glycidoxypropyl trimethoxysilane (GLYMO, 99.0%) and Hexamethyldisiloxane (HMDS, 98%) were purchased from Kelong Chemical Reagent Co., Ltd., Chengdu, China. 3-(aminopropyl) triethoxysilane (APTES, 98%) was purchased from TCI Co., Ltd., Shanghai, China. Deionized water was prepared in laboratory by ultra-pure water system (Ulupure Co., Ltd., China) and used throughout the experiments. All the above reagents were used directly in experiments without further purification.
2.2. Preparation of monodispersed SiO2 nanospheres
Monodispersed SiO2 nanospheres were synthesized through Stöber method, which is a hydrolysis-condensation process of TEOS that catalyzed by ammonia. Typically, TEOS (10 mL), EtOH (80.0 g), and NH3·H2O (6.0 g) were mixed and stirred at 30 °C for 3 h. The solution was aged at 25 °C for 7 days and then a milky SiO2 sol was formed. Followed by the sol was refluxed at 110 °C for 24 h until ammonia was completely removed. Then original SiO2 nanospheres for following experimental steps were obtained. The sol contained 3.0 wt% SiO2.
2.3. Preparation of surface-modified SiO2 nanospheres
The surfaces of silica nanospheres synthesized by Stöber method is rich in hydroxyl groups, which makes the surface modification simple and diversiform. The as-prepared nanospheres were hydrophilic and hydrophobic modified by 3-Glycidoxypropyltrimethoxysilane (GLYMO) and Hexamethyldisiloxane (HMDS) respectively. Typically, GLYMO and HMDS added into the silica sol were 100% and 50% weight of silica, respectively. After 3-day aging, the chemical modified nanospheres were obtained.
2.4. Preparation of dumbbell-like JNPs
Before the coupling between two categories of nanospheres, S50HMDS should be decorated by a small quantity of APTES (70 μL for 1 g SiO2) and stirred for 6 h at 30 °C. At the same time, a small quantity of GLYMO (0.1 g for 1 g SiO2) added to the hydrophilic particles to supply epoxy group which mostly hydrolyzed during 3-day aging. Then mixed the hydrophilic and hydrophobic nanoparticles and immediately stirred for 30 min at 30 °C and aged for 3 days at 25 °C.
2.5. Characterization
In order to demonstrate the changes of hydrophilicity of silica nanospheres after modification, films were prepared on glass substrates with sols by dip-coating process. The hydrophilicity of films was characterized by water contact angle (WCA). The size distributions of particles mentioned above were measured by a laser particle size analyzer (Zetasizer nano-ZS, Malvern Panalytical Ltd., the UK) at 25 °C using EtOH as dispersant, automatically test 3 times and 11 runs for each time. The size and the morphology of particles were determined by field emission scanning electron microscope (FE-SEM, Hitachi S-4800, Japan) and transmittance electron microscope (TEM, FEI Tecnai F20, America). The samples were coated with a thin layer of gold before FE-SEM measurement.
3. Results and discussion
3.1. Properties of silica nanospheres
Original silica nanospheres (o-S) were first synthesized via the Stöber method. The distribution curve and SEM image of original silica nanospheres were shown in . The average size of silica nanospheres was 44 ± 2nm and polydispersity index (PDI) value for the nanospheres was below 0.05, which indicates a good uniformity for the nanospheres. The resulting products after surficial modification can be denoted as S100GLYMO, S50HMDS and S50HMDS-APTES respectively. Also, different ratios of HMDS were added to achieve different hydrophobicity and the modified samples were denoted as S75HMDS and S100HMDS. In order to demonstrate the changes of hydrophilicity of silica nanospheres after modification, coatings were prepared on silicon wafers with sols by dip-coating process. The hydrophilicity of films was characterized by water contact angle (WCA) and the results were shown in . WCAs of coatings constructed by o-S, S50HMDS, S75HMDS and S100HMDS were 30°, 108°, 121° and 136°, respectively (). The WCA of S100GLYMO coating decreased from 82° to 5° over aging time due to the increase of hydroxyls from the hydrolysis of epoxy groups and the evolution of the hydrophilicity was shown in . Thus, after modification by HMDS and GLYMO respectively, two kinds of silica nanospheres with significant difference in hydrophilicity were obtained.
3.2. Dumbbell-like silica JNPs
The whole process of preparing the dumbbell-like JNPs, start with monodispersed SiO2 nanospheres, was shown in . illustrates the fabrication procedure of the dumbbell-like silica JNPs, while presents the detailed reactions between groups on the surfaces of different silica nanospheres [Citation35]. shows a TEM image of dumbbell-like structure of S100GLYMO-S50HMDS-APTES, in which two silica nanospheres were covalently connected and the dumbbell-like JNPs were monodispersed. The particles had different postures in the TEM images since they were randomly deposited on the background. The shooting angle in the TEM was the top view, different morphologies of the dumbbell-like structure appeared in the pictures as shown .
Figure 3. (a) Schematic representation of silica nanospheres’ modification and coupling; (b) The main reactions between groups on the surfaces of silica nanospheres.
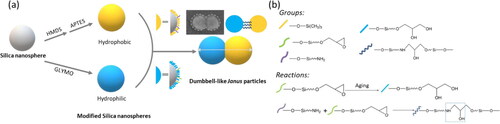
Figure 4. (a) TEM image showing the dumbbell-like structure of S100GLYMO-S50HMDS-APTES; (b-c) different postures of single JNPs in the TEM image. The scale bars of micrographs in (b-c) represent 20 nm.
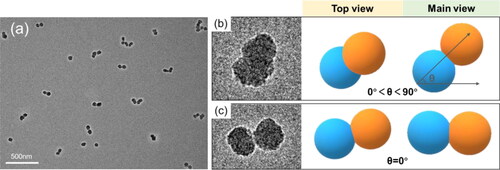
By mixing two sols with different hydrophilicity in equal amounts, the average particle size increased from 44 ± 2nm to 61 ± 1nm, and PDI value still remained below 0.15 (), which indicated that after mixing, silica nanospheres with good monodispersity may be beneficial to studying of the changes in particle sizes before and after coupling. Since the particle size distributions were characterized by dynamic light scattering (DLS), the measurement sizes of non-spherical particles should follow the Equivalent Volume Theory [Citation36–40], which means the measurement result is equal to the diameter of a sphere with equal volume to this particle. Here, we can think of dumbbell-like structure as a cylinder, as shown in , then the equivalent diameter can be calculated using the equations as follow:
(1)
(1)
(2)
(2)
(3)
(3)
Figure 5. (a) Particle size distribution curves for silica nanospheres before and after coupling (blue curve stands for the size distribution of S100GLYMO, orange one for S50HMDS and olive one for S100GLYMO-S50HMDS-APTES). (b) Equivalent volume model of dumbbell-like structure in DLS (dynamic light scattering) measurement (here, RS, RE represent the radius of silica nanosphere and equivalent sphere, respectively).
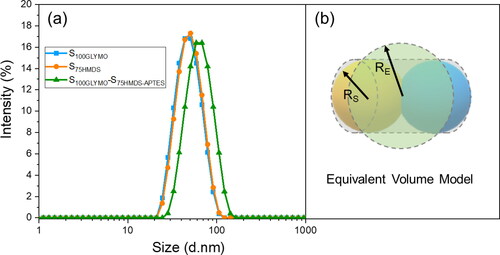
Then, we can obtain,
(4)
(4)
Thus,
(5)
(5)
Where, RS, RC and RE represent the radius of silica nanosphere, equivalent cylinder and equivalent sphere. The calculated value of the equivalent sphere’s radius RE is equal to 1.4422 RS, that is about 65 nm, which is very close to the measurement value 61 nm.
Combing the TEM images and the change in particle size, it can be determined that the dumbbell-like structure has been successfully fabricated.
3.3. Study on coupling reaction of the particles
To further demonstrate that the dumbbell-like structure was the consequence of covalent connection of two nanospheres with diverse hydrophilicity and the detail of the coupling reaction, three control experiments were set up, as shown in . Particle size distribution curves of each sample in were shown in . In sample c, hydrophilic nanospheres S100GLYMO were mixed with hydrophobic nanospheres S50HMDS, which were without modification of APTES in advance. The reaction conditions remained the same as the previous experiment and as a result, the nanospheres were failed to build the dumbbell-like structure since the particle size of sample c remained 44 ± 1nm. So, the coupling agent APTES played an important role in the process of the formation of the dumbbell-like structure. In sample d and e, all of the modification process were same as the previous experiment, but mixed two kinds of nanospheres S100GLYMO and S50HMDS in different ratios 2:1 and 1:2 respectively, the particle sizes were both monodispersed and values were both 56 ± 1nm. Then we studied the evolution of particles sizes within 480 min after mixing, as shown in . The particle size of each sample increased immediately within 40 min, and after that, the values tended to be stable and were the same as the final results. Through these control experiments, we can further determine that the dumbbell-like structure was the result of connecting two nanospheres with different hydrophilicity on one-to-one basis by chemical bonds rather than the hydroxyl condensation or hydrophobic association between nanospheres randomly regardless of hydrophilicity. Furthermore, even if one of the modified nanospheres were excessive, the obtained particle sizes were smaller than calculated value in 1:1 ratio and were unimodal, which means that no trimers or multimers were formed and once the coupling reaction occurred between the nanospheres, the dominant structure was dumbbell-like. In addition, in order to further prove that the connection between the nanospheres are chemical bonds, the sample S100GLYMO-S50HMDS-APTES (1:1) was treated with two kinds of sonication instruments, ultrasonic cleaner (SCIENTC, SB25-12DT, China) and ultrasonic cell disruptor (TL92-IID, Tianling Co., Ltd, Jiangsu Province, China). As shown in , the particle size of the samples remained stable during the 60 min of sonication treatment by two ultrasonic instruments. The agglomeration between particles in a dispersion system can be divided into two categories: soft agglomeration and hard agglomeration [Citation41, Citation42], where the forces of soft agglomeration include electrostatic force, van der Waals force and hydrogen bonding. Therefore, some mechanical external forces can be used to eliminate such forces; in addition to the above-mentioned several forces that cause soft agglomeration, there are also chemical bonds between the particles that cause hard agglomeration, which makes the force of hard agglomeration more effective, thus hard agglomeration cannot be broken simply by mechanical actions. So it proved that the coupling between the nanospheres is the effect of chemical bonds.
Figure 6. Particle size distribution curves of (a) S100GLYMO; (b) S50HMDS; (c) S100GLYMO/S50HMDS (with absence of APTES); (d) S100GLYMO-S50HMDS-APTES (2:1); (e) S100GLYMO-S50HMDS-APTES (1:2).
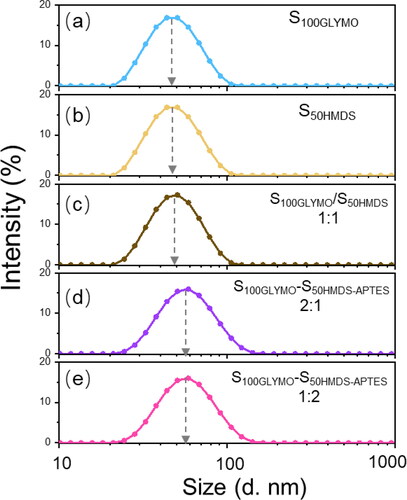
Figure 7. Particle size evolution of S100GLYMO mixed with S50HMDS-APTES in different ratios within 480 min.
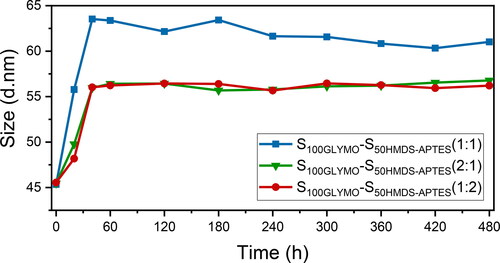
Figure 8. The size evolution of S100GLYMO-S50HMDS-APTES (1:1) under treatments of ultrasonic cleaner (red) and ultrasonic cell disruptor (blue) respectively within 60 min.
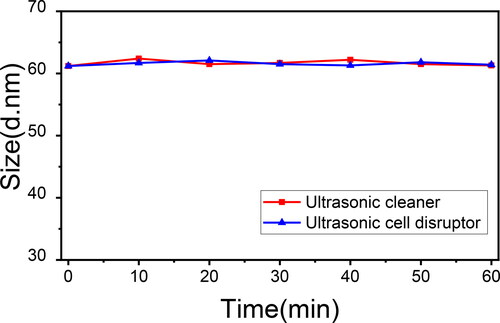
Table 1. Particle sizes and PDI values of modified silica nanospheres (a-b) and samples obtained with different modification or by different mixing ratio (c-e).
The dumbbell-like structure is the dominant conformation in the coupling process of modified nanospheres due to the small amount of coupling active groups as well as the steric hindrance. In the study of Kim et al. [Citation43] on programmable matter, they used an AuNP with one DNA strand as the starting material for the second DNA attachment, and the two for the next. Experiments showed that each step of the attachment, the DNA strand tend to form the largest angle with the previous one, which means the steric hindrance has a conspicuous influence on the arrangement of objects on the particle surface. Their results are comparable with our experiments that the steric hindrance has a major effect of preventing the mixture from the formation of triple or multiple structures.
In order to better understand more details of coupling process for nanospheres, the proportion of coupled silica nanospheres was calculated. According to the Rayleigh Approximation Theory, the intensity distribution is approximately proportional to the sixth power of particle size for small particles in a relatively stable dispersion system [Citation44], as presented in EquationEquation 6(6)
(6) . EquationEquation 7
(7)
(7) presents number distribution for a system containing two kinds of particles.
(6)
(6)
(7)
(7)
Where, Na and Nb are molecules with size a and b respectively. The %Ia and %Na present intensity-weighted and number-weighted distribution respectively for small particles with size a. Then, regardless of very small amount of multimers in the system, we assume that there were only dumbbell-like dimers and residual single nanospheres after the coupling reaction, and peak-fitting of the particle size distribution curve of the product (the curve of S100GLYMO-S50HMDS-APTES (1:1) in ) was performed. As a result, the obtained intensity ratios of o-S and equivalent diameter of dumbbell-like particles were plotted in , which were 13.8% (%Ia) and 86.2% (%Ib), respectively. Finally, combining EquationEquations 7(7)
(7) and Equation8
(8)
(8) and obtained values of %Ia and %Ib, we can obtain the ratio Na: Nb=1.45: 1. Thus, the coupled silica nanospheres account for 58.2% of the total number of nanospheres before the reaction. Moreover, the same calculation method was adopted for sample d and e (results were shown in ), the proportions of coupled spheres were 45.3% and 46.4%, respectively. Since the ratios of nanospheres S100GLYMO and S50HMDS-APTES in sample d and e were 2:1 and 1:2, 67.9% S50HMDS-APTES in sampled d and 69.6% S100GLYMO in sampled e were coupled. In other words, a more thorough transformation from monospheres to dumbbells occurred in a 1:1-mixing system compared with 2:1 or 1:2. As for 2:1- and 1:2-mixing system, the minorities transformed more thoroughly.
Figure 9. Peak fitting analysis of the size distribution of S100GLYMO-S50HMDS-APTES (1:1) was carried out into two monomodal peaks (blue one for monospheres o-S and green one for equivalent diameter of dumbbell-like JNPs).
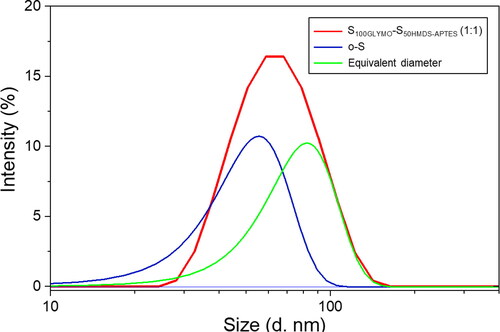
Table 2. The ratios of nanospheres transformed into dumbbell-like structure in sample S100GLYMO-S50HMDS-APTES, d and e.
During the coupling process, nanospheres move and collide randomly due to Brownian motion, hence the intensity of Brownian motion has a significant impact on the progress of the reaction between nanospheres, which is related to diffusion coefficient. According to the Stokes–Einstein relationship [Citation45], diffusion coefficient of small particles suspended in certain medium can be defined by EquationEquation 8(8)
(8) :
(8)
(8)
Where, k is the Boltzmann constant, T is temperature, η is the viscosity of the medium and R is the radius of the particles.
Therefore, if we want to make the particles coupled more thoroughly, we may use smaller nanospheres to build the dumbbell-like structure or in a medium with a lower viscosity.
4. Conclusion
In summary, a feasible and facile way was developed to prepare amphiphilic dumbbell-like Janus nanoparticles by coupling two different modified silica nanospheres through the coupling reaction between the amino groups and the epoxy groups. In this process, hydrophilic and hydrophobic lobes of the JNPs are separately prepared in advance, JNPs with well-defined anisotropic properties can be obtained after coupling. TEM images clearly showed the existence of the dumbbell-like structure, the particle size of the reaction system increased from 44 nm to 61 nm and more than 58% nanospheres were transformed successfully to dumbbell-like structure. This method can be applied to the synthesis of many other dumbbell-like JNPs with asymmetric chemical properties in large scale and also give inspiration to design and synthesis more peculiar nanoparticles with Janus property and nonspherical morphology.
Author contributions
Bo Jiang and Jianhui Luo conceptualized and designed the research; Meiying He performed the synthesis and characterization of the materials; Pingmei Wang, Peiwen Xiao, Xinli Jia and Meiying He analyzed and interpreted the experimental data; Meiying He and Bo Jiang finished writing the manuscript; Bo Xiao supervised the work.
Acknowledgement
We gratefully acknowledge financial support from PetroChina Scientific Research and Technology Development Project (2018A-0907).
Disclosure statement
No potential conflict of interest was reported by the authors.
Additional information
Funding
Notes on contributors
Meiying He
Meiying He received the B.Sc. degree from the Chemistry College of Sichuan University, Chengdu, China, and now is a Ph.D. candidate in polymer chemistry and physics in Sichuan University. Her current research interests include functional polymers, Janus nanocomposites and optical film technology.
Pingmei Wang
Pingmei Wang received the M.Sc. degree from the Department of Oil-Gas Field Development Engineering, Graduate Department, Research Institute of Petroleum Exploration and Development, PetroChina (RIPED) and now is an engineer in Research Institute of Petroleum Exploration & Development (RIPED), PetroChina. Her current research interests include nano chemistry and the technology of intelligent nano oil displacement.
Peiwen Xiao
Peiwen Xiao received the Ph.D. from National Center for Nanoscience and Technology, Chinese Academy of Sciences. He is now an engineer in Research Institute of Petroleum Exploration & Development (RIPED), PetroChina. He is committed to study in technology of intelligent nano oil displacement.
Xinli Jia
Xinli Jia received the M.Sc. degree from the Chemistry College of Sichuan University, Chengdu, China, and now is a Ph.D. candidate in polymer chemistry and physics in Sichuan University. Her current research interest is organic-inorganic nanocomposite materials.
Jianhui Luo
Jianhui Luo received the B.Sc. degree from the Department of Radiochemistry, Peking University. He is currently a professor in Research Institute of Petroleum Exploration & Development (RIPED), PetroChina. His research interests include the intelligent nano oil displacement agent and the technology of the third stage of oil recovery.
Bo Jiang
Bo Jiang received the Ph.D. from Free University of Brussels. He was a visiting scientist in Centre National de la Recherche Scientifique (CNRS), France in 1999. He is now a professor in the Key Laboratory of Green Chemistry & Technology, Ministry of Education, College of Chemistry, Sichuan University, Chengdu, China. His current research interests include thin films (especially antireflective films) technology and surface sciences, Janus nanocomposites, and modification of natural polysaccharides.
Bo Xiao
Bo Xiao received the Ph.D. from Sichuan University. He is in the Key Laboratory of Green Chemistry & Technology, Ministry of Education, College of Chemistry, Sichuan University, Chengdu, China. His current research interests include thin films (especially antireflective films) technology and surface sciences, Janus nanocomposites, and modification of natural polysaccharides.
References
- Casagrande C, Fabre P, Raphaël E, et al. Janus beads: realization and behaviour at water/oil interfaces. Europhys Lett. 1989;9(3):251–255.
- Gennes PGd. Soft matter (nobel lecture). Rev Mod Phys. 1992;64(3):645–648.
- Walther A, Müller AHE. Janus particles: synthesis, self-assembly, physical properties, and applications. Chem Rev. 2013;113(7):5194–5261.
- Yabu H. Fabrication of nanostructured composite microspheres based on the self-assembly of polymers and functional nanomaterials. Part Syst Charact. 2019;36(9):1900178.
- Perro A, Meunier F, Schmitt V, et al. Production of large quantities of “Janus” nanoparticles using wax-in-water emulsions. Colloids Surf A: Physicochem Eng Asp. 2009;332(1):57–62.
- Zenerino A, Peyratout C, Aimable A. Synthesis of fluorinated ceramic Janus particles via a Pickering emulsion method. J Colloid Interface Sci. 2015;450:174–181.
- Xu K, Guo R, Dong B, et al. Directed self-assembly of Janus nanorods in binary polymer mixture: towards precise control of nanorod orientation relative to interface. Soft Matter. 2012;8(37):9581–9588.
- Yang Y, Liu Z, Wu D, et al. Edge-modified amphiphilic Laponite nano-discs for stabilizing Pickering emulsions. J Colloid Interface Sci. 2013;410:27–32.
- Park J-G, Forster JD, Dufresne ER. High-yield synthesis of monodisperse dumbbell-shaped polymer nanoparticles. J Am Chem Soc. 2010;132(17):5960–5961.
- Wu D, Chew JW, Honciuc A. Polarity reversal in homologous series of surfactant-free Janus nanoparticles: toward the next generation of amphiphiles. Langmuir. 2016;32(25):6376–6386.
- Forster JD, Park J-G, Mittal M, et al. Assembly of optical-scale dumbbells into dense photonic crystals. ACS Nano. 2011;5(8):6695–6700.
- Mo AH, Landon PB, Emerson CD, et al. Synthesis of nano-bowls with a Janus template. Nanoscale. 2015;7(2):771–775.
- Kim S-H, Abbaspourrad A, Weitz DA. Amphiphilic crescent-moon-shaped microparticles formed by selective adsorption of colloids. J Am Chem Soc. 2011;133(14):5516–5524.
- Wang X, Guan B, He Y, et al. Synthesis of Janus mesoporous silica nanostructures with organic–inorganic hybrid components through a sprout-like growth method. ChemNanoMat. 2015;1(8):562–566.
- Pfau A, Sander R, Kirsch S. Orientational ordering of structured polymeric nanoparticles at interfaces. Langmuir. 2002;18(7):2880–2887.
- Chen MS, Goodman DW. The structure of catalytically active gold on titania. Science. 2004;306(5694):252–255.
- Zhang J, Shao Q, Wang P, et al. Catalytic hydrogen production by Janus CuAg nanostructures. ChemNanoMat. 2018;4(5):477–481.
- McConnell MD, Kraeutler MJ, Yang S, et al. Patchy and multiregion Janus particles with tunable optical properties. Nano Lett. 2010;10(2):603–609.
- Yi Y, Sanchez L, Gao Y, et al. Janus particles for biological imaging and sensing. Analyst. 2016;141(12):3526–3539.
- Shaghaghi B, Khoee S, Bonakdar S. Preparation of multifunctional Janus nanoparticles on the basis of SPIONs as targeted drug delivery system. Int J Pharm. 2019;559:1–12.
- Wang C, Xu C, Zeng H, et al. Recent progress in syntheses and applications of dumbbell-like nanoparticles. Adv Mater. 2009;21(30):3045–3052.
- Hong L, Jiang S, Granick S. Simple method to produce Janus colloidal particles in large quantity. Langmuir. 2006;22(23):9495–9499.
- Aveyard R. Can Janus particles give thermodynamically stable Pickering emulsions? Soft Matter. 2012;8(19):5233–5240.
- Kim J-W, Larsen RJ, Weitz DA. Synthesis of nonspherical colloidal particles with anisotropic properties. J Am Chem Soc. 2006;128(44):14374–14377.
- Ji X, Zhang Y, Zhao H. Amphiphilic Janus twin single-chain nanoparticles. Chemistry. 2018;24(12):3005–3012.
- Passas-Lagos E, SchüTh F. Amphiphilic pickering emulsifiers based on mushroom-type Janus particles. Langmuir. 2015;31(28):7749–7757.
- Mane G, Akilavasan J, Passas-Lagos E, et al. Site-selective TiO2 coating on asymmetric patchy particles. Langmuir. 2017;33(40):10561–10567.
- Chen T, Chen G, Xing S, et al. Scalable routes to Janus Au-SiO2 and ternary Ag-Au-SiO2 nanoparticles. Chem Mater. 2010;22(13):3826–3828.
- Liu S, Guo S, Sun S, et al. Dumbbell-like Au-Fe3O4 nanoparticles: a new nanostructure for supercapacitors. Nanoscale. 2015;7(11):4890–4893.
- Zeng L, Ren W, Xiang L, et al. Multifunctional Fe3O4–TiO2 nanocomposites for magnetic resonance imaging and potential photodynamic therapy. Nanoscale. 2013;5(5):2107–2113.
- Stöber W, Fink A, Bohn E. Controlled growth of monodisperse silica spheres in the micron size range. J Colloid Interface Sci. 1968;26(1):62–69.
- Khantan N, Shadjou N, Hasanzadeh M. Synthesize of dendritic fibrous nano-silica functionalized by cysteine and its application as advanced adsorbent. Nanocomposites. 2019;5(4):104–113.
- Xiong L, Zheng S, Xu Z, et al. Enhanced performance of porous silicone-based dielectric elastomeric composites by low filler content of Ag@SiO2 core-shell nanoparticles. Nanocomposites. 2018;4(4):238–243.
- Jia X, et al. Synthesis of dumbbell‑like SiO2 nanoparticles with amphiphilic properties in aqueous phase. Chin J Inorgan Chem. 2021;37(4):653–660.
- Sbirrazzuoli N, Mititelu-Mija A, Vincent L, et al. Isoconversional kinetic analysis of stoichiometric and off-stoichiometric epoxy-amine cures. Thermochim Acta. 2006;447(2):167–177.
- DeCarlo PF, Slowik JG, Worsnop DR, et al. Particle morphology and density characterization by combined mobility and aerodynamic diameter measurements. Part 1: theory. Aerosol Sci Technol. 2004;38(12):1185–1205.
- Briard P, Liu Z, Cai X. Measurement of the mean aspect ratio and two characteristic dimensions of polydisperse arbitrary shaped nanoparticles, using translational-rotational ultrafast image-based dynamic light scattering. Nanotechnology. 2020;31(39):395709–395717.
- Chen Y, Briard P, Cai X. Two-dimensional particle-size measurement of nanoparticles in imagery by using dynamic light scattering. Acta Optica Sinica. 2019;39(6):0612005.
- Correia RF, Viseu MI, Prazeres TJV, et al. Spontaneous vesicles, disks, threadlike and spherical micelles found in the solubilization of DMPC liposomes by the detergent DTAC. J Colloid Interface Sci. 2012;379(1):56–63.
- Sate D, Janssen MHA, Stephens G, et al. Enzyme aggregation in ionic liquids studied by dynamic light scattering and small angle neutron scattering. Green Chem. 2007;9(8):859–867.
- Pampach R, Haberkc K. Ceramic powders. Amsterdam: Elsevier Scientific Pub, 1983. p. 623–650.
- Luo P, Nieh TG, Schwartz AJ, et al. Surface characterization of nanostructured metal and ceramic particles. Mater Sci Eng: A. 1995;204(1–2):59–64.
- Kim J-W, Kim J-H, Deaton R. DNA-Linked nanoparticle building blocks for programmable matter. Angew Chem Int Ed Engl. 2011;50(39):9185–9190.
- Stetefeld J, McKenna SA, Patel TR. Dynamic light scattering: a practical guide and applications in biomedical sciences. Biophys Rev. 2016;8(4):409–427.
- Alexander M, Dalgleish DG. Dynamic light scattering techniques and their applications in food science. Food Biophys. 2006;1(1):2–13.