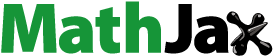
Abstract
Monometallic (Ni, Co or Fe-SiC(O)) and bimetallic (Ni, Co or FeM-SiC(O) with M = Ni or Co) ceramic nanocomposites have been successfully prepared using polymeric precursors obtained by chemical modification of polycarbosilane with metal acetylacetonate. The nanocomposites consist of homogeneously distributed metal nanoparticles within an amorphous SiC(O). The specific surface area (SSA) of Ni, Co or Fe-SiC(O)600 nanocomposites were found to be 155, 50 or 14 m2/g. However, the addition of Fe to the Ni-or Co-containing precursors tends to increase the SSA to 290 or 170 m2/g. Maximum CO2 conversion for monometallic samples was found to be 40% at 500 °C for Ni-SiC(O)600 and maximum CH4 selectivity was 61% at 300 °C for Co-SiC(O)600. The additional presence of Co and Ni in the respective nanocomposites helps to increase the CO2 conversion and selectivity at 500 °C whereas Fe modification shows high methane selectivity at lower temperature < 350 °C.
Graphical Abstract
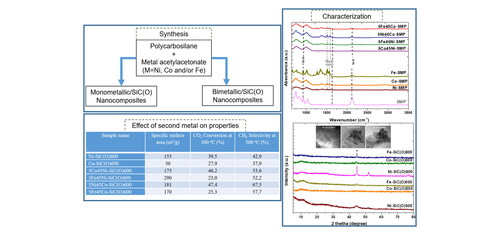
Highlights
Novel nanocomposites consist of bimetallic and SiC(O) ceramic with high specific surface area (SSA) for catalytic applications synthesized via polymer-derived route.
Homogeneously distributed metal nanoparticles (5–7 nm) within amorphous SiC(O) ceramics.
Among monometallic composites, Ni-SiC(O) and Co-SiC(O) show better catalytic results in methanation reaction than Fe-SiC(O).
Introduction of second metal (Ni, Co or Fe) into Ni and Co-SiC(O) ceramic nanocomposites enhances the SSA and catalytic activity in methanation reaction.
In bimetallic nanocomposites Ni and Co increase the catalytic activity and selectivity whereas Fe helps to improve the selectivity at lower temperature.
Introduction
Catalytic hydrogenation of carbon dioxide (CO2) to methane is a potential way to reduce the greenhouse effect and storing energy sources [Citation1–3]. A series of metals, such as Fe, Co, Ni, Ru, Pt, Rh and Pd supported on various porous materials, are usually applied as catalysts in CO2 methanation [Citation4–7]. Mills and Steffgen classified the important metals for methanation catalysts by its activity (Ru>Fe>Ni>Co>Mo) and selectivity to methane (Ni>Co>Fe>Ru) [Citation8]. The catalyst support plays a crucial role in catalysis by stabilizing the active catalysts as well as enhancing the adsorption of the key reactants. However, commercially used catalyst supports like alumina, silica or carbon have some disadvantages, such as poor heat conductivity, high chemical reactivity of alumina and silica and weak oxidation resistance of carbon at high temperatures [Citation9]. Therefore, great efforts are being made to improve the efficiency with development of new catalyst support. Si-based polymer-derived ceramics (PDCs) have been found to be a solution for the above-mentioned drawbacks of alumina, silica and carbon [Citation9–11]. PDCs can be synthesized by a thermolysis of a suitable preceramic polymeric precursor. The process involves the conversion of the polymer to a ceramic with tailored structures, chemical composition and properties. The starting material is a Si-based polymer which opens up the possibility of further modification with metal precursors resulting in a single-source precursor. The ceramic derived from the single-source precursor represent homogeneously built nanocomposites with controlled metal particles size [Citation12–16]. The proposed synthesis route also helps to overcome the problem of conventionally used methods (blending or impregnation) leading to heterogeneous metal distribution (low dispersion of catalyst within support) which in turns reduces the catalytic activity [Citation17,Citation18].
Allyl-hydrido polycarbosilane (SMP-10) is a liquid polymer precursor that has a nominal structure of [Si(CH2CH = CH2)2CH2]0.1[SiH2CH2]0.9. The choice of this particular polymer was favored by the fact that it leads to the direct formation of Si-based ceramic and has Si-H as well as allyl functional groups in its network, which contribute to the tailoring of its chemistry and network architecture. However, the SiC(O) based ceramics produced using metal-modified SMP-10 (M-SMP) (M = V, Ni) have a lower specific surface area (SSA; ∼64 m2/g) or are not porous at higher temperatures (above ∼450 °C) due to the collapse of microporosity [Citation19,Citation20]. However, our previous study showed that the use of metal acetylacetonates increases the specific surfaces area of the ceramic nanocomposites due to the decomposition of the acetylacetonate anion while better cross-linking helps to preserve them up to high temperatures [Citation20].
Even though the catalytic activity of the conventional monometallic catalysts (on commercial supports, such as Al2O3, SiO2, etc.) is well described, further attempts are made to improve the stability (catalyst and support), low-temperature activity and high selectivity of the catalyst in the hydrogenation of CO2. For this purpose, the addition of small amounts of a second metal (Ni, Fe, Co, Ce, La, Mo and Cu) is suitable to further improve the properties of M-based catalysts (M = Co, Ni) [Citation21]. The main drawback of Co and Ni catalysts is their deactivation by coke deposition in the course of the reaction [Citation3,Citation22,Citation23]. However, the presence of iron as a second metal has shown to increase the stability of Ni/Co and improve dispersion. This further help to improve the catalytic activity and selectivity of the catalyst during methanation.
Therefore, this work, on one hand, aims at the production of homogeneously distributed monometallic and bimetallic catalyst in Si-based ceramics. At the same time, it investigates the effect of a second metal (Fe, Co or Ni) on the properties and catalytic activity of respective M-SiC(O) ceramic nanocomposites (M = Ni or Co). For this purpose, metal-containing ceramic nanocomposites were synthesized by the thermal conversion of an allyl hydrido polycarbosilane modified with metal acetylacetonate in an inert gas atmosphere. The produced precursors and the ceramics were investigated in terms of their chemical and phase composition as well as their microstructure. Finally, a preliminary investigation of the catalytic activity of monometallic and bimetallic-SiC(O) ceramic nanocomposites for the hydrogenation of CO2 to methane was carried out.
Experimental section
Materials and synthesis of metal-containing SiC(O) ceramics
Allylhydrido polycarbosilane (SMP, Starfire) was used as the preceramic polymer, while metal acetylacetonate (Macac with M = Ni, Co and Fe, Sigma-Aldrich) has been used for the metal modification in anhydrous toluene (Sigma-Aldrich) as solvent.
The typical synthesis of the metal-containing single-source precursor (M-SMP) was carried out using the Schlenk technique. The desired amount of metal acetylacetonate was first dissolved in toluene and then the liquid polycarbosilane was slowly added with constant stirring (). The resulting mixture was stirred for 24 h at room temperature under argon. The mixture was then heat treated at 120 °C in an oil bath for 4 h to remove the solvent under vacuum. The samples obtained were cross-linked at 250 °C for 3 h and then pyrolyzed at 600 or 800 °C in an argon atmosphere for 2 h at a heating/cooling rate of 50 °C/h.
Table 1. Overview of SMP-10: M(acac) weight ratios of all M-SMP preceramic precursors (M = Ni, Co or Fe) prepared in this work.
Characterization
In order to investigate the chemical structure of the obtained precursors attenuated-total-reflectance FTIR (ATR-FTIR) spectroscopy was performed (Bruker, Ettlingen-Germany, Device Alpha II). Crystalline phases of the produced ceramic materials were investigated using a powder X-ray diffractometer (XRD, Seifert C 3000 Diffraktometer MZ IV ISO, Ahrensburg, Germany) with CuKα radiation. Thermogravimetric analysis (TGA) was used to investigate the polymer-to-ceramic transformation. This analysis was performed under flowing argon (2 L h−1) within a temperature range of 20–800 °C with a heating rate of 10 °C min−1 (TGA; STA503, Bähr-Thermoanalyse GmbH, Hüllhorst, Germany). The SSA was determined by the Brunauer, Emmett and Teller (BET) method from the linear part of the N2 adsorption/desorption isotherm (Belsorp-Mini, Bel Japan, Inc., Toyonaka, Osaka, Japan). In order to minimize nitrogen diffusion effects during the measurements, the samples were ground and sieved with a 300 µm mesh, and degassed at 120 °C for 3 h before the analysis. The distribution of nanoparticles within the ceramic nanocomposites was investigated by transmission electron microscope (FEI Titan 80, at 300 kV). The size analysis, indicating the mean particle diameter of the metallic particles, was carried out by measuring at least 75 particles in the TEM image. A tubular fixed-bed reactor was used to carry out catalytic tests at atmospheric pressure in order to compare the activity and selectivity of each catalyst. First, a homogeneous mixture of 50 mg of the catalyst powder and 300 mg of Al2O3 was made to ‘dilute’ the catalyst powder and transferred to a quartz-glass tube reactor (inner diameter of 6 mm). The total flow rate was adjusted to 50 ml/min composed of H2/CO2/Ar = 1/4/5, resulting in a weight hourly space velocity (WHSV) of 60 L gcat−1 h−1. The catalytic activity was measured in between 200 and 500 °C in intervals of 50 °C. The outflowing gas was continuously monitored with an online compact gas chromatograph equipped with a thermal conductivity detector (HP 5890 series II TCD cell). Furthermore, the CO2 conversion (XCO2) as well as CH4 yield (YCH4) and selectivity (SCH4) were determined according to the following equations:
Results and discussion
Characterization of produced M-SMP precursors
In order to understand the effect of the metal acetylacetonate on the chemical properties of the produced ceramic, the virgin polycarbosilane (SMP) was treated under the same reaction conditions used for the metal-containing precursors and then subsequently analyzed. The ATR-FTIR spectra of the polycarbosilane (SMP) and M-SMP samples are shown in . The absorption band at 1034 cm−1 can be attributed to the Si-CH2-Si stretching of the backbone of SMP-10 and shows the presence of the preceramic polymer. Bands at ca. 1253 and 933 cm−1 are related to SiCH3 stretching and rocking vibration respectively, and confirm the existence of Si-CH3 groups. In the M-SMP samples additional bands associated with the acetylacetonate precursor were observed: the C–O stretching vibration at ∼1593 cm−1, the symmetrical CH3 deformation vibration at 1396 cm−1 and the C–C stretching vibration at 1564 cm−1. In the ATR-FTIR SMP, the strong bands at ca. 2119 cm−1 (Si-H stretching) and 933 cm−1 (Si-H bending) can be assigned to Si-H groups, whereas the bands at 1631 (C = C stretching in –CH = CH2) and 3076 cm−1 (C–H stretching in –CH = CH2) were assigned to the allyl groups [Citation24].
The ATR-FTIR spectrum of the precursors shows a relative decrease in the intensity of the Si-H band and disappearance of allyl-group (unlike in SMP) indicating that the hydrosilylation occurred at the reaction condition. However, the spectra of metal-modified polycarbosilane like Ni and Co-SMP precursors showed an almost complete disappearance of the Si-H band (933 cm−1). The fact that at the same time the amount of allyl substituents is significantly lower than that of Si-H bonds indicates that a dehydrocoupling reaction (cf. Si-H + H-Si = Si-Si + H2) took place under these conditions. For the Fe-containing precursors, the Si-H band (933 cm−1) is still observable, while the C = C band has almost disappeared, indicating that the Fe-SMP undergoes hydrosilylation but the dehydrocoupling reaction has not yet started. Thus, the Ni- and Co-SMP precursors show high reactivity and better cross-linking than the Fe-containing precursor.
The ATR-FTIR spectra of the bimetallic precursors showed almost complete disappearance of the Si-H band and allyl-group indicating that the hydrosilylation and dehydrocoupling reaction occurred in bimetallic precursor under these conditions. It can be concluded that the reactivity and strong crosslinking in all bimetallic precursors (including Fe-containing precursors) are similar to those in the monometallic precursors. This can be explained by the fact that the Ni and Co acetylacetonate were present as the main metal source while the Fe acetylacetonate was present in smaller amounts.
Polymer to ceramic transformation
The thermal decomposition behavior of SMP and M-SMP was investigated by TGA. For this study, the SMP as well as M-SMP and MxMy-SMP precursors were heated to 800 °C in Ar atmosphere (). The results show that Ni-SMP (86 wt%) and Co-SMP (75 wt%) precursors have shown better ceramic yield than the pure SMP (71 wt%) and Fe-SMP (64 wt%) precursors. From our previous studies, it appears that the ceramic yield depends on the degree of cross-linking of the preceramic polymer. The higher the degree of cross-linking, the better the ceramic yield. The FTIR results in this work show that the nickel and cobalt-containing precursors are better cross-linked compared to the Fe-containing precursors and in turn affect the ceramic yield. Furthermore, in the samples containing iron, the weight loss at ≤ 300 °C is very high, which shows that a decomposition of the precursor takes place instead of cross-linking.
Figure 2. TG curves of polymer to ceramic conversion for mono- and bimetallic allylhydrido-polycarbosilane precursors.
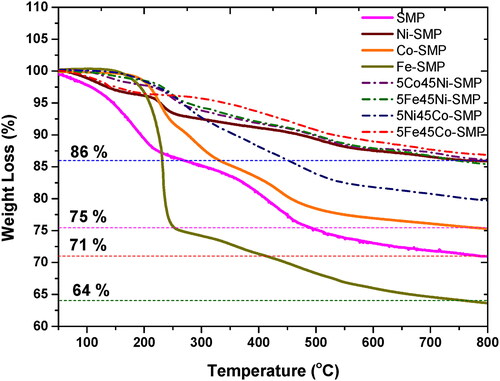
A comparable trend is also found for the bimetallic precursors. The iron-containing nanocomposites 5Fe45Ni-SMP (86 wt%) and 5Fe45Co-SMP (87 wt%) show comparatively high ceramic yield. The higher contents of Ni or Co in the Fe-containing precursor help to increase the degree of cross-linking, which in turn increases the ceramic yield.
It has been shown that Ni-containing precursors produce better cross-linking than Co-containing precursors. In the case of 5Co45Ni-SMP, a ceramic yield of 87 wt% was observed, which almost corresponds to the value of Ni-SMP (86 wt%), since the more cross-link-active nickel is replaced by the weaker cross-linking cobalt. Furthermore, the yield of 5Ni45Co-SMP (81 wt%) is comparatively higher than that of Co-SMP (75 wt%), as cobalt is replaced here by a small amount of the more cross-link-active nickel.
Phase separation, specific surface area and microstructure at high temperature
The phase composition of the monometallic samples pyrolyzed at 600 and 800 °C was investigated by means of XRD measurements (). The samples show main reflection at ca. 45° indicating the crystallization of the different metals in an amorphous SiC(O) matrix. Considering the fact that the metal ions in the precursor are coordinated by oxygen atoms, it can be assumed that MOx/SiC(O) nanocomposite is initially formed during pyrolysis. In the further course of pyrolysis, MOx is then reduced to metallic particles within the SiC(O) ceramic. It is known that the phase composition of the ceramic is thermodynamically controlled by oxidation–reduction reactions that depend on the type of metal used [Citation19,Citation25]. The thermodynamic stability of the metal oxides formed during pyrolysis plays a decisive role. To evaluate this effect more precisely, thermodynamic data for the oxides (MOx) were used, which are shown in the Ellingham diagrams (Figure S1). Here, it becomes clear that an element is unstable and its oxide is stable at higher oxygen potentials. Therefore, the larger the negative value for ΔG, the more stable the oxides. Thus, the oxides of nickel, iron and cobalt are less stable and lead to the formation of metal [Citation19,Citation25]. As expected, the XRD results of the bimetallic samples (not shown here) mainly show the reflection of the crystalline metal, which is present in a dominant amount.
Figure 3. XRD patterns of monometallic allyl hydrido polycarbosilane precursor pyrolyzed at 600 and 800 °C.
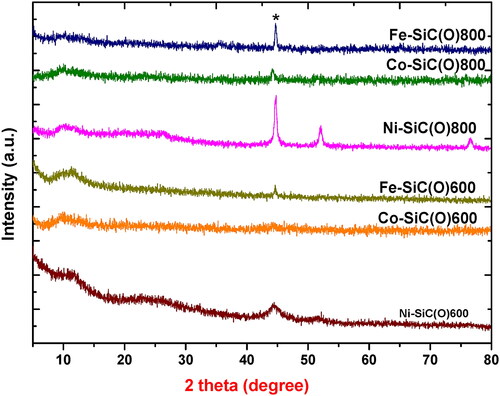
The sample pyrolyzed at 600 and 800 °C were also investigated with regard to their SSA determined using the adsorption isotherms according to BET. The SSA was found to strongly depend on metal modification, type of metal used and pyrolysis temperature (). Metal modification of the polycarbosilane tends to increase the SSA from ca. 5 m2/g for SMP-10 to ca. 14–155 m2/g at 600 °C for metal-containing SiC(O). However, when the pyrolysis temperature is further increased to 800 °C, the SSA decreases (7–52 m2/g).
Table 2. Specific surface area of the mono- and bi-metallic SMP-10 precursors as a function of pyrolysis temperature.
Furthermore, Ni and Co-SiC(O)600 ceramic nanocomposites (155 and 50 m2/g, respectively) had a larger SSA than the Fe-SiC(O)600 (14 m2/g). Due to the decomposition of the acetylacetonate at higher temperatures (600 °C), it increases the porosity and thus the SSA. However, the above-mentioned microporosity collapses as the thermolysis temperature increases (higher than ∼450 °C) [Citation26]. The degree of cross-linking plays a crucial role in preventing the collapse of formed porosity at higher temperatures. The results show that Ni-SiC(O)600 has a better degree of cross-linking than Co-SiC(O)600 and Fe-SiC(O)600, which in turn contributes to the production of higher SSA.
From the flat course of the isotherm of SiC(O)600, it is evident that it is a non-porous material (). In contrast to that, Ni and Co-containing samples pyrolyzed at 600 °C with higher cross-linking degree exhibit a Type I isotherm typical for microporous materials with high SSA. The decomposition of the acetylacetonate contributes to the porosity, but better cross-linking helps to maintain it up to high temperatures. With increasing temperature, the SSA decreases and the sample becomes non-porous (Figure S2).
Figure 4. The nitrogen adsorption/desorption isotherms of the mono- and bimetallic SMP-10 precursors pyrolyzed at 600 °C.
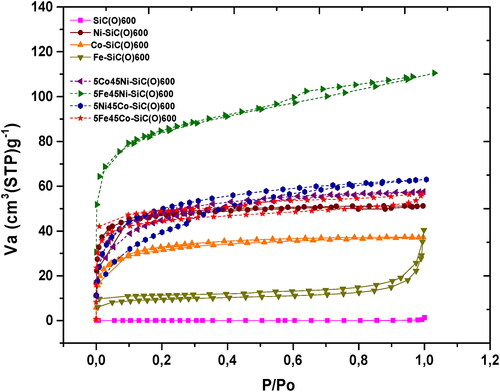
The presence of iron in a bimetallic sample leads to higher SSA compared to the monometallic, but also to most bimetallic samples. In case of Ni-SiC(O)600 addition of iron tends to increases the SSA from 155 to 290 m2/g whereas in case of Co-SiC(O)600 it increases from 50 to 170 m2/g. Due to the complete decomposition of iron acetylacetonate at lower temperatures, as shown by the TGA results, the porosity increases. However, in Fe-containing precursors, Ni and Co-acetylacetonate contribute to good cross-linking and thus prevent porosity breakdown in the bimetallic precursors. In contrast to the presence of a Type I isotherm for the microporous, monometallic Ni-SiC(O)600 and Co-SiC(O)600 samples, the bimetallic 5Fe45Ni-SiC(O)600 and 5Fe45Co-SiC(O)600 samples exhibit a typical type IV isotherm with a pronounced hysteresis loop in the range of 0.4–1.0 P/Po, which is characteristic of mesoporous materials. Furthermore, the strong uptake at a relative pressure (p/po) < 0.1 indicates the simultaneous presence of microporous structures. Therefore, ceramics obtained by pyrolysis of Fe/M-SMP (M = Ni or Co) at 600 °C contain both micropores and mesopores. While micropores are generated by smaller fragments (H2, SiH4, CH3SiH3, CO2, C2H4 and CH4) formed due to better cross-linking, mesopores are apparently initiated by larger acetylacetonate units formed during the sudden decomposition of iron acetylacetonate.
The mono- and bimetallic SiC(O)-based samples were also investigated with the help of TEM. shows that samples with homogeneously distributed nanoscale metal particles were produced. In the case of Ni-SiC(O)600 and Co-SiC(O)600, the metal particles have an average diameter of about 5 nm, while in Fe-SiC(O)600 they are about 7 nm. The bimetallic samples 5Co45Ni-SiC(O)600 and 5Ni45Co-SiC(O)600 contain metallic nanoparticles of approx. 4 and 5 nm, respectively. The presence of iron in bimetallic samples increases the average particle size from 5 to 7 nm. Increasing the pyrolysis temperature leads to an increase of the particle size (Figure S3).
Preliminary investigation of the catalytic properties
In order to analyze the applicability of the metal-containing ceramic nanocomposites for catalytic application, CO2 methanation measurements were performed. All materials produced were investigated with regard to CO2 conversion and CH4 selectivity in a temperature range of 200–500 °C (). In a first step, the influence of the different metals and pyrolysis temperatures (used during the ceramization process) on the catalytic activity was investigated. It is clearly observed that CO2 conversion and CH4 selectivity (except Co-SiC(O)600) increase with increasing temperature for all samples pyrolyzed at 600 °C and reach their maximum at a reaction temperature of 500 °C. The classification of metals for methanation catalysts according to their activity and selectivity for methane was found to be Ru > Fe > Ni > Co > Mo and Ni > Co > Fe > Ru, respectively [Citation8].
Figure 6. CO2 conversion and CH4 selectivity for monometallic (a, b) pyrolyzed at 600 °C and 800 °C and bimetallic (c, d) SiC(O) catalyst pyrolyzed at 600 °C.
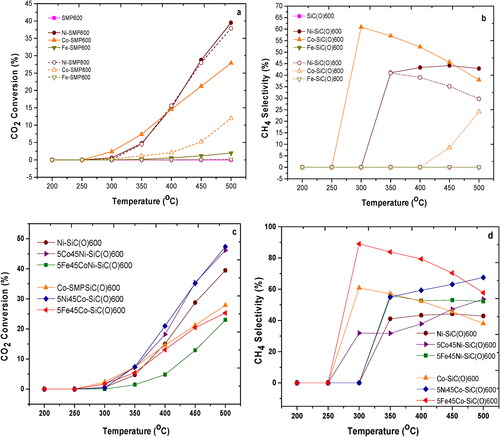
In this work, the highest conversion (∼40%) and selectivity (∼43%) at a measurement temperature of 500 °C were observed for Ni-SiC(O)600 while the values for the Co-SiC(O)600 sample were 28 and 38% and for Fe-SMP600 were 2 and 0%, respectively. Thus, in this study the classification of the metals is different in terms of conversion (Ni > Co > Fe), but the same in terms of selectivity (Ni > Co > Fe). The change in the classification with respect to conversion behavior can be explained by the bigger Fe particle size and very low SSA of Fe-SiC(O)600. It has been shown in literature that bigger Fe particle is responsible for olefin generation [Citation27]. However, a further increase of the pyrolysis temperature to 800 °C reduces the conversion and selectivity of M-SiC(O)800. This behavior can be explained mainly by the size of the metal particles and the SSA, since with increasing temperature the particle size of the metal particles increases while the SSA decreases. In case of the Ni-SiC(O)800 the conversion and selectivity decreases slightly as compared to Ni-SiC(O)600. The overall results show that the Ni-SiC(O)600 and Co-SiC(O)600 samples give better results compared to Fe-SiC(O)600. Maximum CO2 conversion was found to be 40% at 500 °C and CH4 selectivity was 61% at 300 °C for sample Ni-SiC(O)600 and Co-SiC(O)600. However, there is a possibility of further improvement by using a bimetallic system.
In this work, therefore, a preliminary investigation of bimetallic samples was carried out using Ni-SiC(O)/Co-SiC(O) as a base and pyrolyzed at 600 °C. The CO2 conversion and selectivity for the 5Co45Ni-SiC(O)600 bimetallic sample were 46 and 54%, while for 5Ni45Co-SiC(O)600 they were 47 and 68%, respectively. The results clearly show that the presence of Co and Ni in the respective nanocomposites helps to increase CO2 conversion and selectivity. However, the total metal loading was constant in Ni and Co-based samples (11.45%). In the case of the 5Co45Ni-SiC(O)600 samples, the addition of Co helped to increase the reducibility and dispersion of the Ni phase, which in turn increased the catalytic activity [Citation28]. In the case of the 5Ni45Co-SiC(O)600 sample, nickel also helps to increase the degree of cross-linking of the precursor, which in turn leads to an increase in the SSA from 50 to 181 m2/g. Compared to their monometallic counterparts (Ni and Co), the bimetallic 5Fe45M (M = Ni or Co) catalysts show a CO2 conversion of 24 and 25%, respectively. The results obtained are thus comparatively lower than for the monometallic counterparts Ni-SiC(O)600 and Co-SiC(O)600. This can be explained by the lower total metal loading (10.95%). However, the bimetallic 5Fe45M-SiC(O)600 (M = Ni or Co) catalysts show a high methane selectivity compared to the corresponding monometallic samples. For the bimetallic 5Fe45Ni-SiC(O)600 catalyst, it increases from 40 to 52%, while for the bimetallic 5Fe45Co-SiC(O)600 catalyst, it increases from 38 to 58% at a measurement temperature of 500 °C. Furthermore, 5Fe45M-SiC(O)600 (M = Ni or Co) catalysts show high methane selectivity at lower temperatures of 56% for 5Fe45Ni-SiC(O)600 and 89% for 5Fe45Co-SiC(O)600 catalysts at 300 and 350 °C, respectively. This has been attributed in similar bimetallic catalysts on Al2O3 or SiO2 support to the fact that the unreduced FexOy sites formed in-situ enhance CO2 chemisorption and activation [Citation29].
Conclusion
The novelty of the work includes 1) the production of monometallic SiC(O) nanocomposites with high SSA, 2) the development of novel bimetallic SiC(O) ceramic nanocomposites using polymer derived route and 3) investigation of the preliminary catalytic properties of the produced nanocomposites.
High surface area monometallic and bimetallic SiC(O) based ceramic nanocomposites were successfully prepared by the polymer-derived ceramics route using allylhydrido polycarbosilane as a preceramic polymer and metal acetylacetonate as a metal precursor. Nickel-containing precursors have been shown to be better cross-linked, which in turn results in higher ceramic yield and SSA compared to Co- and Fe-containing precursors. The tested synthesis route allows in-situ generation of metal particles in the SiC(O) ceramic, resulting in homogeneous distribution and controlled particle size. The introduction of a second metal (Ni, Co or Fe) into the Co- or Ni-containing precursors increases the SSA of the final nanocomposites.
In the methanation reaction, the highest conversion (∼40%) and selectivity (∼43%) were observed at a measurement temperature of 500 °C for monometallic Ni-SiC(O)600 compared to Co-SiC(O)600 samples (28 and 38%) and Fe-SiC(O)600 (2 and 0%). The additional presence of Co and Ni in bimetallic nanocomposites contributes to the increase in CO2 conversion (46 and 47%) and selectivity (54 and 68%) at 500 °C. Fe-containing bimetallic nanocomposites show a lower conversion but are characterized by high methane selectivity of 56 and 89% at already lower temperatures of 300 and 350 °C, respectively. For further work, 1) the addition of a third metal, as well as the increase of the metal load will be investigated in order to obtain even higher catalytic activity; 2) for practical application monolith catalysts are mostly suitable and the one of the advantages of the PDCs route is the easy shaping of the green body into different shapes with tunable porosity.
Supplemental Material
Download MS Word (924.4 KB)Acknowledgments
The authors also want to convey thanks to Dr. Thomas Kowalik Fraunhofer IFAM Bremen for support in measuring ATR-FTIR.
Disclosure statement
No potential conflict of interest was reported by the author(s).
Additional information
Funding
Notes on contributors
Sarabjeet Kaur
Sarabjeet Kaur, received her Ph.D. degree in Materials Science from Technical University of Darmstadt in 2016. She is currently working as Research Associate in UBC-Center for Ceramics Materials in cooperation with University of Bremen, Germany. Her main research fields are Materials chemistry, synthesis and characterization, Sol-gel synthesis, Polymer-derived ceramics, nanocomposites and processing of ceramics with tailored porosity.
Ana Luiza Silveira Fiates
Ana Luiza Silveira Fiates received her diploma in materials engineering from Universidade Federal de Santa Catarina, Brazil, in 2020. Since 2020 she is working at the Institute for Microsensors, -actuators and -systems (IMSAS) at University of Bremen as a research associate and PhD candidate. Her main research fields are Material’s synthesis and characterization, Sol-gel and supercritical drying techniques as well as materials for sensing applications.
Kurosch Rezwan
Kurosch Rezwan is Professor of Advanced Ceramics at the University of Bremen. He earned his degrees in Materials Science and Engineering from the ETH Zurich. After being a visiting research fellow at the University of California Santa Barbara and at the Imperial College of Science and Technology in London, he joined the University of Bremen in 2006. He was distinguished with the ETH HILTI award for innovation and excellence in science and engineering, the Starting Independent Research Grant by the European Research Council, the Fritz-Behrens award for outstanding scientists and the Wessels award for excellent and innovative industrial cooperation. He and his team's research focus on the design of advanced ceramic materials interfaces for biological environments and biotechnological processes.
Michaela Wilhelm
Michaela Wilhelm received her Ph.D. in inorganic chemistry from Carl-von-Ossietzky University of Oldenburg, Germany, in 2001. In 2002 she joined the Advanced Ceramics Group at the University of Bremen, Germany, where she currently works as Senior Scientist and Head of the Subgroup for Polymer-derived Ceramics. Her research focuses on the development of highly porous, multifunctional ceramics and hybrid materials based on organosilicon polymers for application in separation and energy conversion technologies and in catalysis.
References
- Le TA, Kim MS, Lee SH, et al. CO and CO 2 methanation over supported Ni catalysts. Catal Today. 2017;293–294:89–96.
- Stangeland K, Kalai D, Li H, et al. CO 2 methanation: the effect of catalysts and reaction conditions. Energy Proc. 2017;105:2022–2027.
- Takanabe K, Nagaoka K, Nariai K, et al. Titania-supported cobalt and nickel bimetallic catalysts for carbon dioxide reforming of methane. J Catal. 2005;232(2):268–275.
- Vannice MA. The catalytic synthesis of hydrocarbons from carbon monoxide and hydrogen. Catal Rev. 1976;14(1):153–159.
- Franken T, Heel A. Are Fe based catalysts an upcoming alternative to Ni in CO2 methanation at elevated pressure? J CO2 Util. 2020;39:101175.
- Guo X, Traitangwong A, Hu M, et al. Carbon dioxide methanation over Nickel-Based catalysts supported on various mesoporous material. Energy Fuels. 2018;32(3):3681–3689.
- Frontera P, Macario A, Ferraro M, et al. Supported catalysts for CO2 methanation: a review. Catalysts. 2017;7(12):59–28.
- Mills GA, Steffgen FW. Catalytic methanation. Catal Rev. 1974;8(1):159–210.
- Ledoux MJ, Pham-Huu C. Silicon carbide: a novel catalyst support for heterogeneous catalysis. CATTECH. 2001;5(4):226–246.
- Nguyen P, Edouard D, Nhut JM, et al. High thermal conductive β-SiC for selective oxidation of H2S: a new support for exothermal reactions. Appl Catal, B. 2007;76(3–4):300–310.
- Lacroix M, Dreibine L, de Tymowski B, et al. Silicon carbide foam composite containing cobalt as a highly selective and re-usable Fischer–tropsch synthesis catalyst. Appl Catal, A. 2011;397(1–2):62–72.
- Seifollahi Bazarjani M, Hojamberdiev M, Morita K, et al. Visible light photocatalysis with c-WO(3-x)/WO3 × H2O nanoheterostructures in situ formed in mesoporous polycarbosilane-siloxane polymer. J Am Chem Soc. 2013;135(11):4467–4475.
- Riedel R, Mera G, Hauser R, et al. Silicon-based polymer-derived ceramics: synthesis properties and applications-A review. Nippon Seramikkusu Kyokai Gakujutsu Ronbunshi. 2006;114(1330):425–444.
- Iwamoto Y. Precursors-derived ceramic membranes for high-temperature separation of hydrogen. J Ceram Soc Japan. 2007;115(1348):947–954.
- Adam M, Kocanis S, Fey T, et al. Hierarchically ordered foams derived from polysiloxanes with catalytically active coatings. J Eur Ceram Soc. 2014;34(7):1715–1725.
- Ionescu E, Kleebe HJ, Riedel R. Silicon-containing polymer-derived ceramic nanocomposites (PDC-NCs): preparative approaches and properties. Chem Soc Rev. 2012;41(15):5032–5052.
- Krawiec P, Kockrick E, Simon P, et al. Platinum-catalyzed template removal for the in situ synthesis of MCM-41 supported catalysts. Chem Mater. 2006;18(11):2663–2669.
- Kockrick E, Frind R, Rose M, et al. Platinum induced crosslinking of polycarbosilanes for the formation of highly porous CeO2/silicon oxycarbide catalysts. J Mater Chem. 2009;19(11):1543–1553.
- Kaur S, Cherkashinin G, Fasel C, et al. Single-source-precursor synthesis of novel V8C7/SiC(O)-based ceramic nanocomposites. J Eur Ceramic Soc. 2016;36(15):3553–3563.
- Kaur S, Mônego G, Rezwan K, et al. Synthesis of porous Ni/SiC(O)‐based nanocomposites: effect of nickel acetylacetonate and poly(ethylene glycol) methacrylate modification on specific surface area and porosity. Adv Eng Mater. 2020;22(4):1901036–1901038.
- Ren J, Qin X, Yang J-Z, et al. Methanation of carbon dioxide over Ni–M/ZrO2 (M = Fe, Co, Cu) catalysts: effect of addition of a second metal. Fuel Process Technol. 2015;137:204–211.
- Ocampo F, Louis B, Kiwi-Minsker L, et al. Effect of Ce/Zr composition and noble metal promotion on nickel based CexZr1 − xO2 catalysts for carbon dioxide methanation. Appl Catal A. 2011;392(1–2):36–44.
- Nagaoka K. Influence of the reduction temperature on catalytic activity of Co/TiO2 (anatase-type) for high pressure dry reforming of methane. Appl Catal A. 2003;255(1):13–21.
- Kaur S, Mera G, Riedel R, et al. Effect of boron incorporation on the phase composition and high-temperature behavior of polymer-derived silicon carbide. J Eur Ceram Soc. 2016;36(4):967–977.
- Ionescu E, Terzioglu C, Linck C, et al. Thermodynamic control of phase composition and crystallization of Metal-Modified silicon oxycarbides. J Am Ceram Soc. 2013;96(6):1899–1903.
- Schitco C, Bazarjani MS, Riedel R, et al. NH 3 -assisted synthesis of microporous silicon oxycarbonitride ceramics from preceramic polymers: a combined N 2 and CO 2 adsorption and small angle X-ray scattering study. J Mater Chem A. 2015;3(2):805–818.
- Torres Galvis HM, Bitter JH, Davidian T, et al. Iron particle size effects for direct production of lower olefins from synthesis gas. J Am Chem Soc. 2012;134(39):16207–16215.
- Alrafei B, Polaert I, Ledoux A, et al. Remarkably stable and efficient Ni and Ni-Co catalysts for CO2 methanation. Catal Today. 2020;346:23–33.
- Tsiotsias AI, Charisiou ND, Yentekakis IV, et al. Bimetallic Ni-based catalysts for CO2 methanation: a review. Nanomaterials (Basel). 2020;11(1):28–28.