Abstract
A new type of metal-free oxygen electrode has been proposed. Its distinguishing feature is applying as an active layer nanodispersed graphitic carbon nitride with electrocatalytic properties controlled by synthesis conditions. Optimal conditions of thermochemical synthesis of g-C3N4 by a heat treatment of a solid mixture of melamine precursor and nitrogen-rich urea additive have been defined. The obtained layered g-C3N4 has been characterized by XRD, selected area electron diffraction, TEM and FTIR. An investigation of the electrocatalytic characteristics of oxygen electrodes has been carried out in a fuel half-cell with alkaline electrolyte. The electrochemical characteristics of the obtained g-C3N4 material with melamine-to-urea ratio 1:2 have been shown to be close to those of platinum electrodes. Electrochemical studies have shown that the obtained carbon nitride is a promising material as metal-free catalyst for oxygen electrodes of fuel cells The long-term testing of produced materials confirmed that they were stable over six months.
Layered g-C3N4 was synthesized at different ratios of melamine to urea and its location during synthesis.
Electrochemical studies have shown that the obtained carbon nitride is a promising material as metal-free catalyst for oxygen electrodes of fuel cells.
It is shown that the best electrochemical characteristics of oxygen electrodes are obtained with an excess of nitrogen atoms in the sample.
HIGHLIGHTS
1. Introduction
The use of air/oxygen electrodes in devices generating electrical energy is a promising technology, since it does not create environmental problems and saves non-renewable natural resources. The air/oxygen electrodes in the fuel cells are the three-phase electrode-electrolyte-gas systems, where the processes of generating an electric current are localized at the phase boundaries. The air/oxygen electrode contains a catalytic active layer (a catalyst and the catalyst carrier), and hydrophobic layer.
Platinum metal catalysts are currently regarded as a most effective one for oxygen reduction, but there is a significant drawback - high cost. There is a huge amount of works aiming to investigate other effective catalysts. Another direction of works to create effective catalysts for air/oxygen electrodes is a search for new catalytically active materials and robust carriers. As such, carbon materials are widely known from the literature. Meanwhile, the activated carbon was the first chemically stable material used to create the oxygen electrodes. However, during the operation of activated carbon with a supported catalyst, the catalyst aggregated into large particles, which led to a decrease in the characteristics of such electrodes. In the literature, this process is called ‘catalyst aging.’ Therefore, at present time, multi-walled carbon nanotubes [Citation1,Citation2] and graphene-like structures [Citation3] are widely used as the catalyst support materials with the supported catalyst incorporated into defects in their structure. Such type of catalyst carrier results in creation of various types of bonds between the catalyst nanoparticles and the carbon carrier, which prevents the catalyst aggregation. Thus, it is possible to increase the service life of the air/oxygen electrode and to prevent the catalyst aging caused by its aggregation on the carrier surface. It has been shown that carbon materials doped with heteroatoms are good metal-free catalysts in the oxygen reduction reaction [Citation4]. To such materials belong nitrogen-doped carbon nanotubes and graphenes [Citation5,Citation6]. It was noted [Citation4] that doping with nitrogen is the most effective method for improving the electrocatalytic activity of carbon nanomaterials. Meanwhile, carbon nitride compounds with high N: C ratio can be considered as very promising metal-free next-generation electrocatalysts [Citation6]. Carbon nitrides reveals various crystalline forms/structures, e.g. such as tetrahedral carbon nitride (β-C3N4) and graphitic carbon nitride (g-C3N4) [Citation4]. The β-C3N4 material has high hardness and low compressibility, like diamond, while g-C3N4 is considered as being the most stable under environmental conditions [Citation7]. For g-C3N4, at least two structural isomers have been identified: the first, comprises the condensed melamine (1,3,5-triazine-2,4,6-triamine) units containing an array of periodically repeating single carbon vacancies; the other consists of a compressed melamine (2,5,8,-triamino-tri-s-triazine) subunits containing larger periodic vacancies in the lattice [Citation8]. Carbon nitride materials are also known as effective photocatalysts in the visible range [Citation9].
Two-layer oxygen electrodes are prepared by pressing a hydrophobic layer and a catalytic active layer on a nickel grid (an electric current collector). In such a composite electrode, a triple contact zone electrode-electrolyte-oxygen is formed. The amount of current generated at such electrode depends on the triple contact zone area, as well as on the interaction between the catalysts and the catalyst carrier which accelerate the electrocatalytic reduction of oxygen [Citation10]. In our case, the active layer consists only of g-C3N4 pressed onto a hydrophobic layer consisting of conductive carbon black with polytetrafluoroethylene. An essential distinction of our electrode is the use of a g-C3N4--based nanocomposite as catalytic material. Due to the interaction of g-C3N4 with a hydrophobic layer, a nanocomposite is obtained which increases the triple contact zone surface area where an electric current is generated. Nanocomposites are multiphase or hybrid materials which display markedly different properties from their bulk components. Nanocomposites differ from conventional composite materials by the nano-scale dimensions of the filler phase and exceptionally high surface to volume ratio of this phase. As a result, they often possess unique mechanical, thermal, electrical, optical or catalytic properties which are controlled by factors such as local chemistry, mobility, morphology, or crystallinity. In addition, nanocomposites often offer a combination of several properties, making them even more attractive as multifunctional materials for the future, with potential applications in aerospace, healthcare, energy materials, sensors and other systems. The authors of [Citation11] reported the effect of the nanocomposite composition on the performance of lithium-ion batteries. The authors report the effect of the nanocomposite composition on the performance of lithium-ion batteries and a role of interaction between the composite components on the electrical performance of lithium batteries.
The aim of the present work was to develop a carbon nitride-based hybrid material and to study its application potential as a metal-free catalyst for oxygen electrodes of fuel cells depending on the synthesis conditions.
2. Experimental
All chemical reagents were purchased from Aldrich (Gillingham, UK). A thermochemical synthesis by a heat treatment of a solid mixture of precursors was used for the preparation of g-C3N4 material. This method consists in heating up the mixture of melamine precursor and nitrogen-rich urea CO(NH2)2, additive in a lid-covered crucible to a temperature of 550 °C for 4 h. The synthesis technique was described in [Citation12,Citation13]. The heating rate was 2 °C/min. For the synthesis, we have employed different urea-to-melamine ratios as well as different arrangement of the chemicals in the reaction crucible, such as: i) melamine powder over that of urea, and ii) both powders uniformly mixed. The resulting materials were purified from impurities by boiling in concentrated nitric acid for half an hour. For the solutions preparation and subsequent washing of the samples, we used bidistilled water. Depending on melamine-to-urea ratio and the reagents arrangement, the obtained C3N4 samples were denoted as follows: I- melamine-to-urea weight ratio 1: 56.5, melamine on top; II – melamine-to-urea weight ratio 1:2, melamine on top; III – melamine-to-urea weight ratio 1:1, melamine and urea uniformly mixed; IV- melamine-to-urea ratio 1:1, melamine on top. It is known that during the thermal decomposition of urea CO(NH2)2, a gaseous ammonia is released, which creates a nitrogen-rich atmosphere in a tightly closed reaction crucible, so we assumed that during the reaction, an excess urea would release more nitrogen resulting in a formation of nitrogen doped carbon nitride C3N4. So, the selected 4 samples were chosen to study the effect of the nitrogen amount released from urea additive during the heat treatment of the reaction mixture as well as the reagents arrangement in the reaction cubicle on electrocatalytic performance of synthesized C3N4 in oxygen electrodes.
To compare the electrocatalytic properties of the obtained C3N4 materials in the oxygen reduction reaction, the current-voltage characteristics of the oxygen electrode were studied. A comparison of the properties of the obtained materials was carried out by estimating the magnitude of the current generated at polarization of 350 mV. An operative two-layer oxygen electrode was prepared by pressing its components onto a nickel grid. The hydrophobic layer contained 0.07 g/cm2 of conductive carbon black with 25% polytetrafluoroethylene. An active layer of the oxygen electrode comprised 0.014 g/cm2 of the prepared g-C3N4 material with 5% polytetrafluoroethylene. Electrochemical load characteristics were recorded in galvanostatic mode. U-shaped electrolyzer with an alkaline electrolyte served as a source of oxygen. Oxygen was supplied to the gas electrodes under an overpressure of 0.01 MPa. Before measurements, the oxygen electrode was purged with oxygen for an hour. The studies were carried out in a fuel half-cell, with a zinc electrode used as an anode. The electrolyte was a 6 M potassium hydroxide solution in bidistilled water. The fuel half-cell for the study of the obtained materials as an oxygen electrode was described in [Citation14]. The scheme of the fuel half-cell is shown in .
Figure 1. Mock-up of the fuel half-cell. 1 - cell housing: 2 - pressure clutch; 3 – metal current lead and tube for oxygen supply; 4 - metal grid of the oxygen electrode; 5 - hydrophobic layer of oxygen electrode; 6 - the active layer of the oxygen electrode from the g-C3N4 particles; 7 - polytetrafluoroethylene gasket; 8 - reference electrode; 9 - zinc anode.
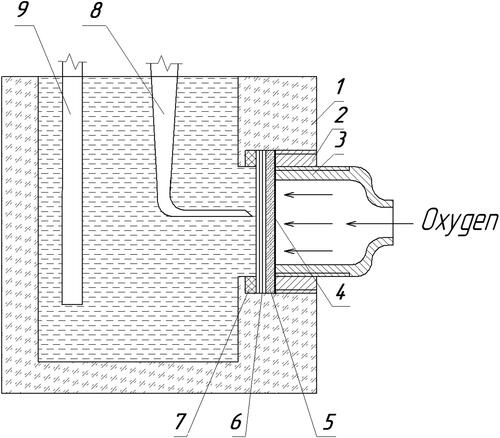
To compare the parameters of the investigated electrodes, the reference electrodes made of the active layer of multiwalled carbon nanotubes (MWCNT) with supported platinum black in an amount of 10 wt.%, electrochemically deposited from aqueous solution consisting of 3% H2PtCl6 with 0.2% lead acetate (II) at 1 V voltage over 2.5 min. The electric current direction was changed every 30 s.
The morphology of the prepared C3N4 nanostructures has been studied using a JEM-100 CXII electron microscope. X-ray diffraction measurements have been carried out with a DRON-4 X-ray diffractometer with CuKα radiation. The samples for transmission electron microscopy (TEM) were prepared by dissolving the prepared C3N4 material in асetone during 30 min followed by depositing a drop of the solution onto a copper mesh and drying the samples for 5 min.
The porous structure of prepared materials was studied by low temperature nitrogen adsorption. Nitrogen adsorption/desorption isotherms were recorded at −196 °C using a NOVA 2200 surface gas analyzer (Quantachrome). The specific surface area was calculated by the BET method.
FT-IR transmission spectra were recorded using an INVENIO R (Bruker) spectrometer in the range of 650–3800 cm−1. The samples of g- C3N4 were prepared in the form of tablets with KBr powder. The spectra were baseline corrected; the frequencies were determined by the second derivative method using the Opus 8.2 software.
Electronic absorption spectra were recorded with a Perkin Elmer UV/vis Lambda 35 spectrophotometer. PL excitation and emission spectra were recorded in the region of 300 − 700 nm with a Perkin Elmer LS 55 instrument, the light source was a xenon lamp (Xe). Samples of the synthesized materials were ground in agate mortar to the state of finely dispersed powder. The 0.05 g of the prepared powder was applied onto a 30 μm thick quartz slide with a spatula and mixed with several drops of immersion liquid (98% glycerin). Then this emulsion was evenly distributed over the surface of the quartz window and pressed against the second quartz one until a vitreous transparent mass was formed. All measurements of the spectra were made relative to quartz windows with a layer of immersion liquid. Optical absorption spectra were used for the band gap calculation.
The study of the polarization curves has been carried out using a standard three-electrode system with a potentiostat IPS-Pro (Volta). The working electrodes were prepared by pressing 0.1 g of the synthesized g-C3N4 material onto a nickel grid. The electrode area was 2 × 1.2 cm2. The auxiliary electrode was a platinum plate with an area of 6 cm2. An Ag⁄AgCl electrode attached via a salt bridge was used as a reference in these experiments. Catalytic activities of the electrocatalysts were evaluated by cyclic voltammetry in an oxygen аnd nitrogen saturated aqueous solution of 1 M KOH at room temperature. The saturation of the solutions with oxygen before the study was carried out for half an hour. The potential sweep rate was 10 mV/s. The interval from −1 V to +0.6 V relative to Ag⁄AgCl reference electrode was investigated.
3. Results and discussion
A finely crystalline yellow g-C3N4 powder was obtained in thermochemical synthesis as described above. shows X-ray diffraction patterns of different g-C3N4 samples I - IV. The X-ray diffraction patterns of the samples III and IV of the same composition with different arrangement of the reaction mixture in the crucible were identical.
Figure 2. X-ray diffraction patterns of the prepared carbon nitride samples: I (curve 1); III (curve 2); II (curve 3); IV (curve 4).
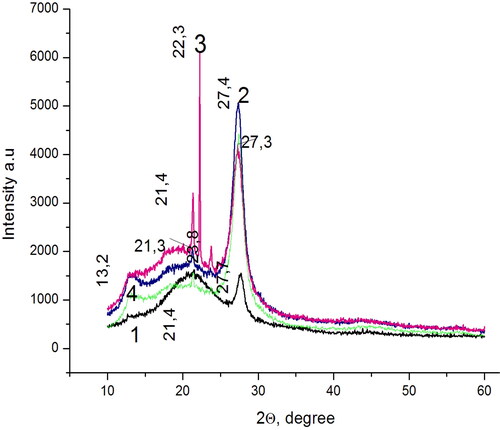
A small peak observed at 2θ ≈ 13.2° for all samples can be attributed to the (100) face of g-C3N4, and the corresponding distance between structural heptazine blocks within individual carbon nitride monolayers makes up 0.67 nm (). The diffraction peak at 2θ ≈ 27.3° and other close to this, which are also displayed by all four samples correspond to the interplanar distance between the monolayers d = 0.326 nm of the (002) face of g-C3N4 [Citation15]. This value is in the range typical for g-C3N4 from 0.319 to 0.326 nm. Other small (100) peak around 2θ ≈ 21.3° can be ascribed to the in-plane repeated units of fully polymerized triazine. The peak at 2θ ≈ 22.3° (which is most pronounced in sample II) is responsible for carbonated urea residues [Citation16], and the peak at 23.8° is responsible for carbonated melamine residues [Citation17]. The resulting material in its structure corresponds to g-C3N4 [Citation15].
shows a TEM image of a single larger particle of the synthesized g-C3N4 sample II (of about 1 µm in size) consisting of the particles of different sizes (submicron and less) and shapes. The lighter areas of the image correspond to a smaller thickness areas (tens of nm), and the darker ones to a larger thickness.
Figure 3. The TEM image of a rather large g-C3N4 particle (of about 1 µm) demonstrating the complicated shape of the prepared particles of II and their high transparency to electron beam. The SAED pattern in the inset shows two very weakly expressed wide (similar to amorphous) diffraction rings, with two pairs of symmetric reflections and a separate reflection. These bright reflections are located in the middle of weak diffraction rings indicating that the large crystalline particles are of the same g-C3N4 origin as the small ones.
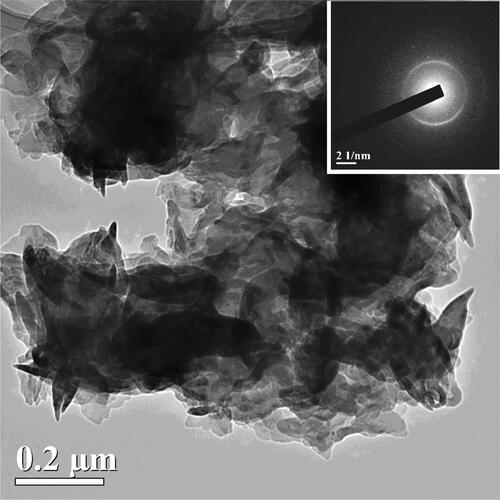
The SAED pattern (see inset) shows two very weakly expressed wide (similar to amorphous) diffraction rings, with two pairs of symmetric reflections and a separate reflection clearly seen. It should be noted that these bright reflections are located in the middle of weak diffraction rings. The position of bright reflections means that the large crystalline particles consist of the same g-C3N4 material as the small ones. All the prepared C3N4 samples are heterogeneous and have the similar surface area that is why we present the data for one sample only.
In , the FT-IR spectra of the prepared C3N4 samples are presented. In the C3N4 IR absorption spectra, the vibrations of C-N and C = N bonds are registered, as well as vibrations of the triazine ring as a whole [Citation18,Citation19].
Figure 4. IR spectra of the prepared C3N4 samples I-IV in the regions of: (a) 3800–2600 and (b) 1800–700 cm−1. For comparison, IR spectrum of bare KBr disk is shown (blue curve).
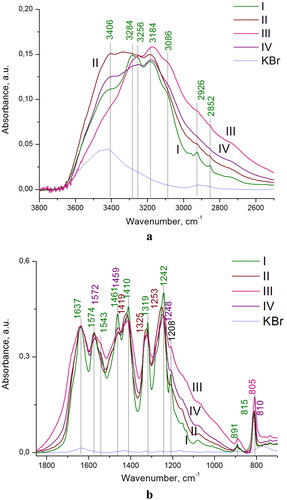
The breathing modes of triazine rings were found at 815 cm−1 and 891 cm−1. The bands observed in the region of 1207-1640 cm−1 refer to C-N and C = N stretching vibrations in heterocycles. In particular, the bands centered at 1461, 1410, 1319 and 1242 cm−1 can be assigned to C-N, and those at 1543, 1574, and 1637 cm−1 to C = N stretching vibrations (). The set of bands observed in the region of 3600–2400 cm−1 can be assigned to hydrogen-bonded N-H and O-H groups, the bands centered at 2926, and 2852 cm−1 to CH2 stretching, and the band at 3086 cm−1 to aromatic CH (). The appearance of these CH stretching vibrations bands is associated with the presence of defects in the structure, vacancies, in place of breaks of nets and boundaries of the particles. In the case of g-C3N4 sample I (), the IR spectra display strong absorption bands belonging to triazine rings centered at 1543, 891, 815 cm−1 which are not observed in others C3N4 samples.
shows the results of the band gap calculation for the obtained C3N4 samples [Citation20].
Figure 5. Optical absorption spectra of g-C3N4 samples: I – curve 1; II –curve 2; IV –curve 3; III – curve 4. As the nitrogen content in the obtained materials decreases in order of I < II < III = IV, the band gap increases in the same order due to a decreased number of the defects states. The upper location of melamine precursor over the urea powder during the synthesis of III results in the production of nitrogen-doped С3N4, showing the largest band gap of 2.4 eV for the 1:1 components weight ratio.
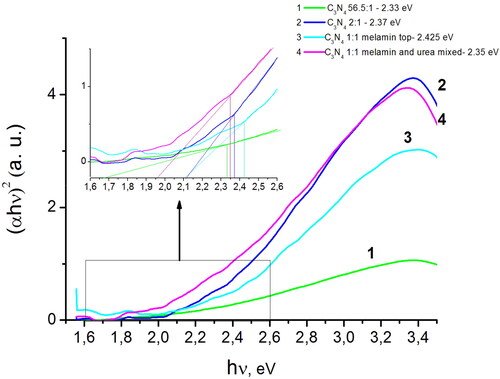
In order to eliminate the contribution of impurities, unreacted starting materials, and intermediate synthesis products, the band gap was determined according to the procedure described in [Citation21]. As can be seen from the , the band gap depends on the weight ratio of the components in the reaction mixture and their arrangement during the synthesis, namely, it is the largest (2.4 eV) for the sample III. Consider the chemical formulas of urea, (NH2)2CO, and melamine, C3H6N6, melamine contains more nitrogen than urea, and therefore, when melamine powder is placed over that of urea, a material with lower nitrogen content is synthesized. Due to the fact that during melamine decomposition, a larger amount of ammonia is released in a reaction, which leads to nitrogen consumption in the reaction zone. Therefore, the nitrogen content in the obtained material decreases and the band gap increases due to a decreased number of defect states. In [Citation22], the authors also showed that the narrowing of the band gap is caused by changes in the electronic structure due to an increase content of the N in g-C3N4. The amount of N in the g-C3N4 depends on the used nitrogen-rich precursors (such as melamine and urea and others) and the methods of synthesis. Since the band gap and properties of nitrogen-containing carbon materials change from semiconductor to metallic ones with an increase in nitrogen content, as proved by Yang et al. [Citation23], we assume that the upper placement of melamine powder during the synthesis leads to the formation of nitrogen-depleted С3N4, as is the case in sample III with the band gap ≈ 2.4 eV compared to I, II, IV - in which the band gap is 2.3 eV. Therefore, it can be assumed that in samples I, and II there is an increasing nitrogen content which will improve the electrocatalytic properties of the prepared materials in the oxygen reduction reaction, which was confirmed by the following electrochemical studies.
In , the electric current density at the working electrode is plotted versus the applied voltage (cyclic voltamperogram) for the synthesized C3N4 samples under oxygen flow.
Figure 6. Cyclic voltammograms with oxygen flow for the g-C3N4 samples: III –curve 1; IV – curve 2; II – curve 3; I –curve 4.
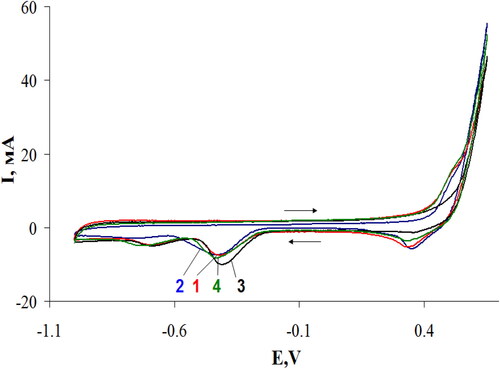
As can be seen from , curve 3, the peaks for the sample II are shifted to the positive side, which indicates a higher catalytic activity of sample II in the oxygen reduction reaction compared to other samples. This increase in catalytic activity can be explained by the enrichment of g-C3N4 with nitrogen, which leads to the unique properties of this composite in the reaction of oxygen reduction. The oxygen electrodes were made of the synthesized materials. For the comparison, the reference oxygen electrodes made of initial MWCNTs, the synthesized C3N4 and of carbon nanotubes with supported platinum were investigated in a fuel half-cell with an alkaline electrolyte and a zinc anode. The specific surface area calculated by the BET method of all investigated materials was 130–150 m2g−1. Therefore, using the same weighed portion of the catalytic material, the electrocatalytic characteristics of the oxygen electrodes can be estimated from the electric current density at the same potential. The higher is the current density at the electrode at the same potential, the better are the electrocatalytic characteristics of the test material in the oxygen reduction reaction. In the introduction, we stated that: It has been shown that carbon materials doped with heteroatoms are good metal-free catalysts in the oxygen reduction reaction [Citation4]. To such materials e.g. belong carbon nanotubes and graphenes doped with nitrogen [Citation5,Citation6]. It was noted in [Citation4] that doping with nitrogen is the most effective method for improving the electrocatalytic activity of carbon nanomaterials. Therefore, carbon nitrides with high nitrogen content can be considered as very promising metal-free electrocatalysts [Citation6]. Therefore, we explain the increase in catalytic activity by increasing the amount of doped nitrogen. The results of the studies of the current density as a function of electrode potential are shown in .
Figure 7. Current-voltage characteristics of the oxygen electrodes with an active layer made of different electrode materials: C3N4 (IV) - curve 1; C3N4 (III) – curve 2; C3N4 (I) – curve 3; C3N4 (II) – curve 4; and MWCNTs with 10 wt.% Pt - curve 5.
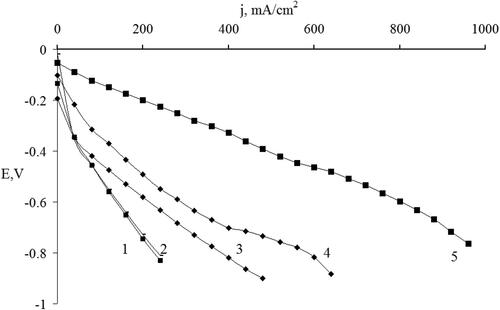
As can be seen from , the location of melamine in the crucible does not affect the electrochemical characteristics of the electrodes (, curves 1 and 2). The best I-V characteristics are obtained for C3N4 sample II with melamine-to-urea ratio 1:2 (, curve 4). Thus, it turns out that by the synthesis requires an excess of urea, and in our opinion, the most optimal melamine-to-urea ratio is obtained of 1:2. The correlation of catalytic activity depending on the composition was explained by the enrichment of the synthesized samples of g-C3N4 by nitrogen. This increase in catalytic activity can be explained by the enrichment of g-C3N4 with nitrogen, which leads to the unique properties of this composite in the reaction of oxygen reduction. Thus, if we compare the magnitudes of the electric current at the same potential value for example at 0.4 V. In , these samples are arranged in the following sequence according to the magnitude of the generated current: sample IV - curve 1; sample III - curve 2; sample I - curve 3; sample II - curve 4; and MWCNTs with deposited Pt curve 5. This dependence can be explained by the enrichment of g-C3N4 with nitrogen, with the formation of hybrid composites with different structures, which was shown by the above physicochemical studies. This leads to the unique properties of such a composite in the reaction of oxygen reduction. This makes it possible to obtain carbon nitride samples enriched with nitrogen, which is confirmed by the presence of XRD peak at 2θ ≈ 22.3° which is responsible for carbonated urea residues in sample II (, curve 3).
This is also confirmed by the results of cyclic voltammetry (). The current-voltage characteristics of the C3N4 electrodes () show that the samples of the synthesized C3N4 demonstrate a good electrical conductivity. The electrochemical studies have shown that the obtained g-C3N4 material is a promising candidate as a metal-free catalyst for oxygen electrodes of fuel cells with characteristics close to those of Pt electrodes. Long-term experiments were also carried out on the stability of the oxygen electrodes made of the g-C3N4. During cell operation, the alkaline electrolyte and the zinc anode were periodically replaced. The long-term testing results are shown in .
Figure 8. Results of the long-term testing of a two-layer oxygen electrode with an active layer consisting of 0.014 g cm−2 of C3N4 (II) coupled with a zinc anode under constant operating current density at the oxygen electrode of 200 mA cm−2.
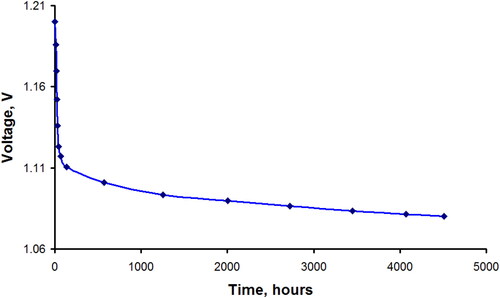
It is worth to mention that the produced materials were stable over six months under testing at 200 mA·cm−2 at the oxygen electrodes. The dependence of the potential over time at the current density of 200 mA·cm−2 shows that the structure of this composite does not change during the operation time of the oxygen electrode. Calculated electrochemical characteristics of the oxygen electrode of fuel half-cell based on g-C3N4 are 220 mW cm−2 at the polarization 250 mV at room temperature. These calculations were obtained from the characteristics of the fuel half-cell U = 1.11 V at a current density of 220 mA cm−2 according to . This data is comparable to 623 mW cm−2 for oxygen electrode based on carbon nanotubes containing 0.15 mg cm−2 Pt and working with pressure of 3 atm and temperature of 70 °C [Citation2]. Our electrodes worked at room temperature and an overpressure of 0.01 MPa, so these results are better, since the characteristics increase several times with an increase in temperature and pressure.
4. Conclusions
A new type of metal-free oxygen electrode for fuel cells has been developed. Its distinguishing feature is applying as an active layer nanodispersed g-C3N4 with improved electronic and electrocatalytic properties controlled by synthesis conditions.
The thermochemically synthesized g-C3N4 samples have a porous layered structure with interspersed nanocrystals, which is confirmed by X-ray diffractions, FTIR spectroscopy and TEM analysis. The electrochemical studies have shown that the obtained g-C3N4 is a promising candidate as a metal-free catalyst for oxygen electrodes of fuel cells, and their characteristics are close to those of platinum electrodes.
Disclosure statement
No potential conflict of interest was reported by the authors.
Additional information
Funding
Notes on contributors
M. O. Danilov
M. O. Danilov, candidate of science in electrochemistry, working in Vernadsky Institute of general and inorganic chemistry of NASU, Department of electrochemistry and photoelectrochemistry of nonmetallic systems, research interest in electrochemistry, materials chemistry and nanotechnology.
G. I. Dovbeshko
G. I. Dovbeshko, Professor, doctor of sciences, working in Institute of Physics of NASU, Department of Physics of Biological Systems, has many years of experience in the fields of biophysics and solid state physics, soft matter. She is an expert in FTIR, SEIRA, UV, Raman, SERS, CARS, NMR spectroscopy, Langmuir-Blodgett technique, AFM microscopy, confocal microscopy.
I. A. Rusetskyi
I. A. Rusetskyi, candidate of science in electrochemistry, working in Vernadsky Institute of general and inorganic chemistry of NASU, Department of electrochemistry and photoelectrochemistry of nonmetallic systems, he is highly qualified specialist in the field of electrochemistry and photoelectrochemistry. He has many years of experience in the field of alternative energy-converting systems, the study of photoelectrochemical properties of semiconductor heterostructures, composites, nanomaterials, as well as in the study of charge transfer processes at the semiconductor-electrolyte interface.
V. N. Bykov
V. N. Bykov, Candidate of Physical and Mathematical Sciences, works at the Institute of Physics, Department of Physical Electronics, is a specialist in electron microscopy and X-ray structural analysis of metal and semiconductors, research interest in the field of solid state physics, materials science and condensed matter physics. His skills and expertise are thin films and nanotechnology, nanomaterials, SEM analysis, XRD analysist, TEM image analyses, EDX, particle size analysis.
O. P. Gnatyuk
O. P. Gnatyuk, doctor of sciences, working in Institute of Physics of NASU, Department of Physics of Biological Systems, has many years of experience in the field of vibrational spectroscopy of biomolecules, cells and tissues, as well as the study of their interaction with antitumor drugs and nanoparticles.
S. S. Fomanyuk
S. S. Fomanyuk, candidate of science in electrochemistry, working in Vernadsky Institute of general and inorganic chemistry of NASU, Department of electrochemistry and photoelectrochemistry of nonmetallic systems, research interest in the field of electrochemistry and photoelectrochemistry. Area of expertise and research interests includes electrochemical synthesis of oxide films of tungsten, molybdenum, titanium, niobium, and nickel, bismuth tungstates and vanadates.
G. Ya. Kolbasov
G. Ya. Kolbasov, Professor, doctor of sciences, Head of Department of electrochemistry and photoelectrochemistry of nonmetallic systems of the Vernadsky Institute of General and Inorganic Cemistry of NASU, has many years of experience in the fields of electrochemistry and photoelectrochemistry of semiconductors, nanomaterials and nanotechnologies, electrochemical and optical sensors, photoelectrochemical converters of solar energy.
References
- Danilov MO, Melezhyk AV. Carbon nanotubes modified with catalyst—promising material for fuel cells. J. Power Sourc. 2006;163(1):376–381.
- Wang X, Waje M, Yan Y. CNT-based electrodes with high efficiency for PEMFCs. Electrochem. Solid-State Lett. 2005;8(1):A42.
- Shao Y, Zhang S, Wang C, et al. Highly durable graphene nanoplatelets supported Pt nanocatalysts for oxygen reduction. J. Power Sourc. 2010;195(15):4600–4605.
- Wang M, Wu Z, Dai L. Graphitic carbon nitrides supported by nitrogen-doped graphene as efficient metal-free electrocatalysts for oxygen reduction. Electroanal Chem. 2015;753:16–20.
- Yu D, Zhang Q, Dai L. Highly efficient metal-free growth of nitrogen-doped single-walled carbon nanotubes on plasma-etched substrates for oxygen reduction. J Am Chem Soc. 2010;132(43):15127–15129.
- Qu L, Liu Y, Baek J-B, et al. Nitrogen-doped graphene as efficient metal-free electrocatalyst for oxygen reduction in fuel cells. ACS Nano. 2010;4(3):1321–1326.
- Liu AY, Cohen ML. Prediction of new low compressibility solids. Science. 1989;245(4920):841–842.
- Lyth SM, Nabae Y, Moriya S, et al. Carbon Nitride as a nonprecious catalyst for electrochemical oxygen reduction. J. Phys. Chem. C. 2009;113(47):20148–20151.
- Wang X, Maeda K, Thomas A, et al. A metal-free polymeric photocatalyst for hydrogen production from water under visible light. Nat Mater. 2009;8(1):76–80.
- Danilov MO, Ya. Kolbasov G. Electrochemical method for the preparation nanocomposites based on carbon nanotubes and chromium oxides for oxygen electrodes. J Solid State Electrochem. 2010;14(12):2169–2172.
- Liang C-L, Liu Y, Bao R-Y, et al. Sodium ascorbate assisted uniform distribution of Fe 3 O 4 nanoparticles on rGO surface: improved cycling and rate performance of rGO/Fe 3 O 4 anode material for lithium ion batteries. Nanocomposites. 2015;1(3):170–175.
- Dante RC, Martín-Ramos P, Correa-Guimaraes A, et al. Synthesis of graphitic carbon nitride by reaction of melamine and uric acid. Mater Chem Phys. 2011;130(3):1094–1102.
- Danilov MO, Dovbeshko GI, Rusetskii IA, et al. Carbon Nitride is a Non-Metallic catalyst for oxygen electrodes for fuel cells. ECS Trans. 2021;105(1):87–96.
- Danilov MO, Rusetskii IA, Dovbeshko GI, et al. Electrochemical Synthesis partially unzipped Multi-Walled carbon nanotubes as electrode materials for fuel cells. ECS Trans. 2019;95(1):273–282.
- Xu L, Li H, Xia J, et al. Graphitic carbon nitride nanosheet supported high loading silver nanoparticle catalysts for the oxygen reduction reaction. Mater Lett. 2014;128:349–353.
- Madhurambal G, Mariappan M. Growth and characterization of urea-thiourea non-linear optical organic mixed crystal. Indian J Pure Appl Phys. 2010;48:264. http://nopr.niscpr.res.in/bitstream/123456789/7645/1/IJPAP%2048%284%29%20264-270.pdf
- Ma HA, Jia XP, Chen LX, et al. High-pressure pyrolysis study of C 3 N 6 H 6 : a route to preparing bulk C 3 N 4. J. Phys.: Condens. Matter. 2002;14(44):11269–11273.
- Li W, Chen Q, Zhong Q. One-pot fabrication of mesoporous g-C3N4/NiS co-catalyst counter electrodes for quantum-dot-sensitized solar cells. J Mater Sci. 2020;55(24):10712–10724.
- Gao W, Zhao Y, Mao Z, et al. Enhanced visible light photocatalytic activity for g-C3N4/SnO2: sb composites induced by Sb doping. J Mater Sci. 2018;53(13):9473–9485.
- Zanatta AR. Revisiting the optical bandgap of semiconductors and the proposal of a unified methodology to its determination. Sci Rep. 2019;9(1):11225.
- Makuła P, Pacia M, Macyk W. How to correctly determine the band gap energy of modified semiconductor photocatalysts based on UV-Vis spectra. J Phys Chem Lett. 2018;9(23):6814–6817.
- Starukh H, Praus P. Doping of graphitic carbon nitride with Non-Metal elements and its applications in photocatalysis. Catalysts. 2020;10(10):1119.
- Yang Z, Hu K, Meng X, et al. Tuning the band gap and the nitrogen content in carbon nitride materials by high temperature treatment at high pressure. Carbon. 2018;130:170–177.