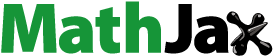
Abstract
In the present study, MnO2 nanoparticles were synthesized using Citrus limetta peels extract and functionalized by chitosan polymer. Surface morphology analysis of chitosan functionalized MnO2 nanoparticles was carried out using a scanning electron microscope (SEM) and transmission electron microscope (TEM), which revealed that the synthesized nanoparticles were spherical, with a size range of 14–24 nm. Energy dispersive X-ray analysis and elemental mapping were used to observe Mn, O, C, H, and N. Fourier-transform infrared spectroscopy confirmed the presence of hydroxyl, carboxyl, and amino groups on the surface of the nanoparticles. The kinetics and isotherms were compared and it was found that the pseudo-second-order and Langmuir isotherm were the best fit, with R2 values of 0.99. The thermodynamic study demonstrated that the adsorption was endothermic and spontaneous. These findings indicate that chitosan functionalized nanoparticles have a better Pb(II) removal efficiency (94.40%), making them an eco-friendly and cost-effective alternative for wastewater treatment.
Chitosan functionalized nanoadsorbent was synthesized through green route.
Sorption mechanism explored through isotherm, kinetics, and thermodynamic models.
Synthesized adsorbent showed high Pb(II) removal capacity.
Highlights
1. Introduction
Heavy metal contamination in the water is caused by several industrial and other anthropogenic activities. The researchers have given top priority to metallic pollutants due to their high toxicity [Citation1]. These substances occur naturally in ecosystems, albeit in trace amounts [Citation2]. However, some metal ions exhibit higher toxicity levels even at low concentrations [Citation3,Citation4]. The primary routes of exposure to Pb(II) are through contaminated food, water, and Pb(II)-contaminated dust in the air. Pb(II) toxicity is commonly associated with liver damage, kidney malfunction, cardiac failure, and neurological impairment [Citation5]. The initial symptoms of Pb(II) toxicity include headaches, memory loss, and dullness [Citation6]. Furthermore, Pb (II) also disrupts the synthesis of RBCs, leading to anaemia. Pb(II) is also classified as a potential carcinogen that causes several types of cancer [Citation7]. The World Health Organization (WHO) has issued a permissible limit of 0.01 mg/L of Pb(II) in drinking water [Citation8].
Therefore, there is an urgent need to eliminate the contamination of Pb(II) from wastewater [Citation9]. Several technologies such as reverse osmosis, membrane filtration, ion exchange, solvent extraction, precipitation, flocculation, electro-dialysis, and coagulation are used for the extraction of Pb(II) from aqueous medium [Citation10]. However, these methods are expensive, partially ineffective at removing trace amounts of Pb(II) in wastewater, and generate secondary chemical sludge [Citation11]. On the other hand, biological methods such as bioaccumulation, bio-conversion/bio-transformation are cost-effective and eco-friendly and can be considered as better alternative of conventional methods [Citation12].
Biosorption is widely recognized as an environmentally safe and cost-effective approach for eliminating Pb(II) [Citation13]. Various biosorbents, such as wheat husk, fruit waste, rice husk, microbial biomass, plant residue, and nanomaterials, have been frequently employed for the remediation of Pb(II) and other heavy metal ions [Citation14]. Nanomaterials possess small size and large surface area, rendering them distinct from other biosorbents and enabling them to be effective biosorbents for Pb(II) extraction from wastewater [Citation15]. Numerous types of nanomaterials, comprising silver, iron, gold, grapheme, and other polymers, have been synthesized for the heavy metal adsorption. Among these nanomaterials, manganese dioxide (MnO2) is deemed to be environmentally safe and effective for Pb(II) removal [Citation16]. The utilization of plant extracts in the production of MnO2 nanoparticles is a highly effective and ecologically sustainable approach, owing to its cost-effectiveness and superior efficacy as a nanoadsorbent. This method stands out as a more favourable option when compared to the physiochemical methods utilized in the production of other nanoparticles [Citation17]. Turmeric powder-derived curcumin extract (CE) acts as a stabilizing agent that provides stability and prevents the aggregation of nanomaterials in water [Citation18]. Surface modification of adsorbents using chitosan biopolymers enhances the effectiveness of the MnO2 nanoparticles. Chitosan is typically derived from the arthropods, such as crabs and fungi [Citation19]. Its structure contains various functional groups that play vital role in Pb(II) adsorption [Citation20,Citation21].
This study aims at the development of a low-cost, reusable, and efficient chitosan functionalized MnO2 nanoadsorbent for Pb(II). MnO2 nanoparticles were synthesized by green route by Citrus limetta peels extract (CLPE) and turmeric powder-derived CE. The nanoparticles were functionalized with chitosan. The effectiveness of chitosan-functionalized MnO2 nanoparticles (Ch-MNPs) was evaluated in terms of Pb(II) removal. The adsorption mechanisms of Ch-MNPs were assessed through goodness-of-fit in kinetic, thermodynamic, and isotherm models.
2. Material and methods
2.1. Synthesis of Ch-MNPs
CLPE was derived from the peels of the Citrus limetta fruit. About 3 g of turmeric powder was mixed with 100 mL of ethanol and boiled for 5 min to prepare the CE. The resultant CE was obtained by centrifugation at 6000 rpm for 10 min, followed by filtration through Whatman filter paper. About 100 mL manganese acetate of 0.10 mM strength was then added to 100 mL of CLPE in the presence of 20 mL of CE as a stabiliser for MnO2 nanoparticles. NaOH and HCl were added to the mixture to maintain a pH of 8. The mixture was subjected to constant stirring for 120 min at 37 °C, after which it was centrifuged at 6000 rpm. A mixture of ethanol and deionized water was used to wash precipitated MnO2 nanoparticles. The nanoparticles were coated with chitosan after they had been prepared. An acetic acid (w/v) solution containing 0.1% chitosan was prepared. The mixture of 5 g of MnO2 nanoparticles in 0.1% chitosan solution was mixed for 2 h. The resultant Ch-MNPs were centrifuged, washed, oven dried, and finally stored in air tight containers in dark for further experiments.
2.2. Characterization of Ch-MNPs
The surface morphology of Ch-MNPs was studied through scanning electron microscope (SEM), ZEISS MA15/18 make, USA. Before and after Pb(II) adsorption, the elemental composition of Ch-MNPs was determined using an energy dispersive X-ray analyzer (EDAX Inc. make, USA). An NICOLET spectrophotometer (iS5) was used to determine the surface functional groups by measuring Fourier Transformation Infrared (FTIR) spectra. Additionally, the morphological characteristics, encompassing the dimensions, contours, and crystalline features of the Ch-MNPs, were successfully determined via the utilization of High-Resolution-Transmission Electron Microscopy (HR-TEM), FEI Company, make USA.
2.3. Pb(II) adsorption
We conducted the adsorption of Pb(II) in a 100 mg/L solution containing 50 mL of working solution. The pH was kept constant at 6. The experiment evaluated the impact of initial Pb(II) concentration (ranging from 50 to 200 mg/L), Ch-MNP dose (ranging from 0.5 t0 2.5 g/L), pH levels (ranging from 2 to 10), temperature (ranging from 20 to 50 °C), contact time (ranging from 60 to 300 min), and agitation rate (120–240 rpm). The samples were centrifuged at 5000 rpm for 15 min after being collected from the flask.
Pb(II) removal percentage and uptake capacity (qe) of Ch-MNPs were calculated from EquationEquations (1)(1)
(1) and Equation(2)
(2)
(2)
(1)
(1)
(2)
(2)
where initial and equilibrium concentrations of Pb(II) (mg/L) were denoted as Ci and Ce, respectively. V and W represented the volume of Pb(II) ion solution (L) and the weight of Ch-MNPs (g). The concentration of Pb(II) was measured by an inductively coupled plasma optical emission spectrometer (ICP-OES) (Perkin Elmer Optima 7000 DV make, USA).
2.4. Isotherm models
Langmuir, Freundlich, Temkin, and Halsey isotherms were tested to evaluate goodness-of-fit of the experimental data. These isotherms also provided a deep insight into the mechanism of Pb(II) adsorption over the surface of nanoparticles.
2.4.1. Langmuir isotherm
The Langmuir isotherm assumes that metal ions are adsorbed uniformly, forming a monolayer. It has been mathematically represented in EquationEquation (3)(3)
(3)
(3)
(3)
the maximum adsorption capacity was denoted by
(mg/g), and the isotherm constant b (L/mg) can be determined by calculating the slope and intercept of the graph obtained by plotting
vs. Ce.
2.4.2. Freundlich isotherm
Friendlich isotherm assumes that adsorbent has heterogeneous surface along with multilayer coverage. Freundlich’s isotherm can be expressed as:
(4)
(4)
where kf (mg/g) and n were isotherm constants and determined by evaluating the slope and intercept of the plot between log
vs. log
2.4.3. Temkin isotherm
In Temkin isotherms, all molecules in a single layer are considered to be heated by adsorption. As the uptake capacity increases, the relationship decreases linearly rather than logarithmically. EquationEquation (5)(5)
(5) provides linearized form of the Temkin isotherm
(5)
(5)
where R was universal gas constant (8.341 J mol−1 K−1), T was temperature (ᵒK), AT and bT were model constants. Specifically, AT and bT were related to heat of adsorption (L/g) and the maximum binding energy (kJ/mol) respectively. A plot between qe and lnCe was used to determine AT and bT values.
2.4.4. Halsey isotherm
This Halsey isotherm indicates how multilayer adsorption develops at a considerable distance from the surface of the adsorbent due to the four-layer adsorption. Equation (6) illustrates the Halsey isotherm and its characteristics
(6)
(6)
The constants ( and
) for the Halsey isotherm can be expressed in the following way. These constants can be calculated from the slope and intercept of the plot. It is possible to calculate these constants by slopes and intercepts of the plot between
and
2.5. Kinetics
Adsorption kinetics shows the mechanism of Pb(II) adsorption onto the adsorbent surface. Systems for wastewater treatment can be designed, developed, and scaled up using it [Citation22]. In this study, pseudo-first-order, pseudo-second-order, and Elovich kinetic models have been investigated to understand the adsorption kinetics of Pb(II) ions onto Ch-MNP surfaces.
2.5.1. Pseudo-first-order
The pseudo-first-order kinetics takes into account the reversible binding of ligands to an adsorbent surface. The linear form of pseudo-first-order kinetics is shown in EquationEquation (7)(6)
(6)
(7)
(7)
where ks is the equilibrium constant, and it can be calculated based on the slope of
) vs. time (t). Adsorption capacities (mg/g) at time t and equilibrium were denoted by qt and qe, respectively.
2.5.2. Pseudo-second-order kinetics
The pseudo-second-order kinetics postulates that surface sorption acts as the rate-determining factor, which includes chemisorption. This mode has been shown in EquationEquation (8)(7)
(7)
(8)
(8)
where
is the equilibrium constant
(9)
(9)
Here, and h are constants and these constant can be calculated from the plot between t/qt vs. t.
2.5.3. Elovich model
The underlying assumption of this model considers that the solid surface of the adsorbent is characterized by energetic heterogeneity. A chemisorption rate on the energetically heterogeneous adsorbent surface can also be explained by this theoretical framework. The kinetic model of Elovich can be seen in EquationEquation (10)(9)
(9)
(10)
(10)
where α (μg/g/min) and β (g/μg) were the initial adsorption rate and the extent of surface coverage.
2.6. Thermodynamics
The investigation of adsorption’s nature and thermodynamic viability was conducted through observing the alterations in Gibbs free energy (ΔG°), enthalpy (ΔH°), and entropy (ΔS°). The calculation of the thermodynamic parameters was executed by EquationEquations (11)(10)
(10) , Equation(12)
(11)
(11) , and Equation(13)
(12)
(12)
(11)
(11)
(12)
(12)
(13)
(13)
In the aforementioned equation, the equilibrium concentration Cae (mg/L) is the concentration at equilibrium, Ce (mg/L) denotes the equilibrium Pb (II) ions concentration in the bulk solution, T represents the temperature of the reaction in Kelvin, and R signifies the universal constant (8.314 J mol−1 K−1). The values for ΔS° and ΔH° were calculated through the use of the intercept and slope of the graph depicting the natural logarithm of kc vs. 1/T.
2.7. Desorption of Pb(II) and regeneration of Ch-MNPs
A stripping agent containing 0.10 M HNO3 was used in the desorption experiment. A quantity of 0.10 g of Pb(II) ion-loaded dried biosorbent was combined with 50 mL of 0.10 M HNO3 and left to incubate at ambient temperature for one hour. After this incubation period, the remaining concentration of Pb(II) in the solution was measured. Desorption of Pb(II) from the biosorbent into the solution was calculated from EquationEquation (14)(13)
(13)
(14)
(14)
CD indicates the Pb(II) concentration desorbed into the solution and VD represents the volume of the desorbed solution in Litres (L). Additionally, m signifies the mass (g) of Ch-MNPs, and qe (mg/g) denotes the adsorption capacity. To assess the reusability of the biosorbent, five cycles of adsorption–desorption were conducted. A thorough wash with distilled water was performed following each cycle, and the adsorbent was then dried in an oven at 105 °C.
2.8. Statistical data analysis
The adsorption experiments were conducted in triplicate (n = 3) and the mean values were utilized for the creation of graphical representations. Furthermore, the deviations (±standard deviation) were determined and depicted in the graphs as error bars.
3. Result and discussion
3.1. FTIR analysis
FTIR analysis of Chitosan, MNPs, Ch-MNPs, and Pb (II) ions loaded Ch-MNPs is represented in .
Figure 1. FTIR spectra of chitosan, MNPs, Ch-MNPs, and Pb (II) loaded Ch-MNPs [Citation16].
![Figure 1. FTIR spectra of chitosan, MNPs, Ch-MNPs, and Pb (II) loaded Ch-MNPs [Citation16].](/cms/asset/4b716f92-2da7-4fcb-87d0-de199166e76b/ynan_a_2347804_f0001_c.jpg)
FTIR spectra at 3700–3584 cm−1 indicated the O–H stretching. FTIR spectra at 3359, 3228, and 3344 cm−1 were detected on Chitosan, MNPs, Ch-MNPs, and Ch-MNPs-Pb(II) indicated to –NH2 and O–H groups. The FTIR spectra at 1716 cm−1 at all samples indicated to the C = C [Citation23]. The peak at 798 and 897 cm−1 on Chitosan, MNPs, and Ch-MNPs indicated to –OH group. FTIR spectra at 1026 cm−1 indicated the C–O [Citation11]. There are slight variations in the FTIR of chitosan, MNPs, and Ch-MNPs were reported. Changes in the FTIR spectra were indicated by the Pb(II) adsorption ions onto Ch-CMNPs. The change in the FTIR of spectra in Pb(II) loaded Ch-MNPs was reported between the ranges of 3700 and 3584 cm−1. This indicates the O–H stretching involved in the Pb(II) adsorption. Moreover, the FTIR spectra at 700–1200 cm−1 were also disappeared in Pb(II) loaded Ch-CMPs which indicates that surface hydroxyl (O–H) involved in the Pb(II) adsorption. Samrot et al. [Citation24] investigated heavy metal adsorption onto chitosan-functionalized iron nanoparticles and observed similar kinds of results.
3.2. HR-TEM
The analysis of Ch-MNPs via HR-TEM was examined and subsequently presented in .
Figure 2. HR-TEM analysis represented the size and shape of Ch-MNPs (a–b). Arrows indicate the lattice fringes (b), (c) SAED pattern [Citation16].
![Figure 2. HR-TEM analysis represented the size and shape of Ch-MNPs (a–b). Arrows indicate the lattice fringes (b), (c) SAED pattern [Citation16].](/cms/asset/db0b60bf-63c4-4044-a931-54b85b91bf1e/ynan_a_2347804_f0002_c.jpg)
Nanoparticles synthesized were spherical and uniform in size, according to HR-TEM images (). The Ch-MNPs were small in size about 14–23 nm . The parallel lattice fringes on the Ch-MNPs were observed which confirmed the crystalline and uniform structure of Ch-MNPs [Citation25]. Through diffraction rings formed by open crystals in the selected area of the SAED pattern (), it was confirmed that the nanoparticles were crystalline. Because nanoparticles have crystallinity, they can be cycled multiple times without degrading significantly, which enables nanoparticles to be used as an adsorption-desorption catalyst in numerous applications [Citation26]. Guo et al. [Citation27] synthesized MnO2 synthesized particles and observed similar kinds of properties of MnO2 nanoparticles.
3.3. HR-SEM
The surface property including morphology and shape of Ch-MNPs was analyzed via HR-SEM. The surface properties of Ch-MNPs are critical for heavy metal adsorption, as demonstrated by Guo et al. [Citation27] and Buccolieri et al. [Citation28]. depicts the HR-SEM images of Ch-MNPs.
Figure 3. HR-SEM micrographs of Ch-MNPs before (a) and after Pb (II) adsorption (b) [Citation16].
![Figure 3. HR-SEM micrographs of Ch-MNPs before (a) and after Pb (II) adsorption (b) [Citation16].](/cms/asset/161da66f-a246-4ba7-a245-4b81d2b144ca/ynan_a_2347804_f0003_b.jpg)
The analysis of a and b revealed that Ch-MNPs exhibited uniform aggregation and spherical shape. a shows the rough surface of Ch-MNPs. No alteration in the nanoparticle morphology was observed pre- () and post-Pb(II) adsorption (). The small size, large surface area, and rough surface of Ch-MNPs indicated towards a preferential adsorption of Pb(II). Due to their rough surfaces and small sizes, Ch-MNPs provided an increased surface area for Pb(II) adsorption [Citation29]. The chitosan coating rendered the nanoparticle surface porous and uneven in morphology [Citation30,Citation31].
3.4. EDX analysis and elemental mapping
The analysis utilizing EDX was conducted to validate the existence of Pb(II) which persisted in its bound state after the adsorption process. Furthermore, the fundamental elements that carried out a significant function in the synthesis of Ch-MNPs were also verified. A comparison of Ch-MNP analyses before and after Pb(II) adsorption is presented in .
Figure 4. EDX analysis (a) and elemental mapping representing the distribution of all elements (b), carbon (c), nitrogen (d), oxygen (e), manganese (f), and Pb (g).
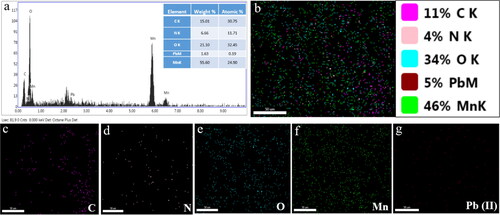
The elemental analysis depicted in demonstrated that Mn and O were the primary elements in the Ch-MNPs. This observation can be attributed to the successful synthesis of MnO2 nanoparticles [Citation32,Citation33]. The presence of C was also detected, albeit in minor amounts, which was attributed to the chitosan coating on the MnO2 nanoparticles. Moreover, the EDX analysis of Ch-MNPs further confirmed the existence of Pb(II) on the surface of these nanoparticles.
In the elemental mapping, Mn (55%) and O (23%) were the most abundant components (). The presence of C (), N () and O () confirmed the chitosan coating on MnO2 nanoparticles. It was also confirmed by elemental mapping () that the Pb(II) ions are biosorbed onto the surfaces of Ch-MNPs due to the homogeneous distribution. Tho et al. [Citation34] performed adsorption of multiple heavy metals (As+3, Pb+2, Cd+2, and Cr+6) on ZnO nanoparticle-coated biochar. According to the authors, modified biochar is a good adsorbent for removal of heavy metal ions.
3.5. Adsorption study
3.5.1. Optimization of process parameters
In the present study, various parameters such as pH, temperature, initial metal ion concentration, Ch-MNP dosage, contact time, and agitation were examined in order to study the impact of all these variables on the removal of Pb(II). Pb(II) removal is shown in as a function of these parameters.
Figure 5. Effect of pH (a), initial Pb(II) concentration (b), Ch-MNPs dosage (c), temperature (d), contact time (e), and agitation rate (f) for Pb(II) adsorption onto Ch-MNPs.
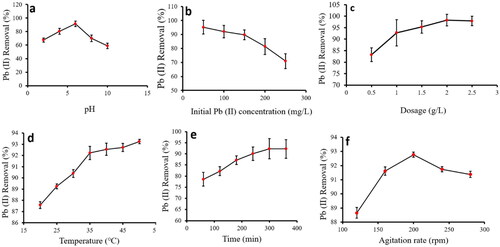
It was observed that the pH had a significant effect on the removal of lead (II) from the solution () within the range of 2–10. Increasing pH up to 6 resulted in a higher removal of Pb(II), with pH 6 resulting in the highest removal (a). When the pH is increased beyond 6, the amount of Pb(II) being removed reduced. Due to intense competition between hydrogen ions in the liquid medium and metal ions, both Pb(II) removal at acidic pH levels (2–5) and peak removal at neutral pH levels (6–7) were low. The highly charged atmosphere makes it very difficult for hydrogen ions to acquire active sites. As a result, Pb(II) adsorption onto Ch-MNPs tends to decrease [Citation35]. However, when the pH of the solution increases the number of free hydrogen ions in the solution declines, so the competition for active sites declines, and more number of Pb(II) ions were more likely to be adsorbed to the surface. Researchers have reported that Pb(II) removal by chitosan-coated MnO2 nanoparticles was maximally effective at pH 6, and that removal decreased as pH increased [Citation35].
It has been demonstrated by that using a range of initial metal ion concentrations from 50 to 250 mg/L, Ch-MNPs retain less heavy metal than their counterparts in an environment containing Pb(II) ions. In this study, it was observed that the best elimination of Pb(II) was achieved at a concentration of 50 mg/L of Pb(II). It has been observed that a reduction in the proportion of heavy metal ions being eliminated occurs at concentrations above 50 mg/L. This decline in removal percentage above 50 mg/L was attributed to the lack of availability of active sites on the surface of the Ch-MNPs. Farghali et al. [Citation36] examined the removal of lead (II) by copper oxide nanostructures. Authors found that as the concentration of lead (II) increased the lead (II) removal got decreased.
The effect of Ch-MNPs dosages on the removal of Pb(II) is shown in c. The efficacy of heavy metal ion elimination was observed to increase proportionally with the augmentation in the dose of Ch-MNPs, ultimately culminating in the attainment of maximum heavy metal removal at 2.5 g/L. The increase in heavy metal removal with the increase in Ch-MNPs dosage was due to rise in the number of active sites available for adsorption [Citation37]. Gaur et al. [Citation38] investigated the behaviour of Pb(II) adsorption using soya bean waste and observed that the removal of Pb(II) was influenced by the increase in adsorbent dosage.
The removal of Pb(II) using Ch-MNPs was investigated over a temperature range of 20–50 °C. The results demonstrated that the removal efficiency of Pb(II) increased rapidly up to 35 °C, after which it slowed down between 35 and 50 °C (d). The highest elimination of Pb(II) was achieved at a temperature of 50 °C. The increase in Pb(II) removal with the upsurge in solution temperature suggested that the adsorption is endothermic. There is a possibility that the enhanced adsorption of Pb(II) ions at the higher temperature might be due to the activation of the surface of Ch-CMNPs [Citation39]. Using magnetite nanoparticles, Fato et al. [Citation40] removed heavy metal ions, including Pb(II), from river water. Authors observed an increase in metal ion removal with higher solution temperature, up to a maximum of 45 °C.
The current inquiry embarked upon an exploration of the analysis of the influence of the duration of contact within the span of 60–360 min. At the 300-min, the maximum elimination of Pb(II) (as seen in ) was achieved. Beyond this point, there was a conspicuous absence of any significant increase in the adsorption. This phenomenon was attributed to the saturation of all active sites on the surface of Ch-MNPs at 300 min, resulting in no or negligible adsorption thereafter. The Pb(II) removal increased with swelling in agitation time mediated by activated carbon made up from maize tassels for Pb(II) removal [Citation41].
We evaluated the effects of agitation on heavy metal adsorption on Ch-MNPs under various agitation rates (120–280 rpm) during Pb(II) adsorption (f). As agitation rate increased up to 200 rpm, Pb(II) removal increased, but after that, there was no increase. As turbulence increased in the aqueous phase, Ch-MNPs were more likely to collide with metal ions and the boundary layer surrounding them was thinner, leading to this increase in turbulence [Citation42].
3.5.2. Isotherm
Isotherm study based on initial concentrations of metal ions of 50–200 mg/L and temperatures of 20–50 °C, Pb(II) adsorption was investigated. shows Pb(II) adsorption data fitted with Langmuir, Freundlich, Temkin, and Halsey isotherms.
Figure 6. Langmuir (a), Freundlich (b), Temkin (c), and Halsey (d) isotherms of Pb(II) adsorption on Ch-MNPs.
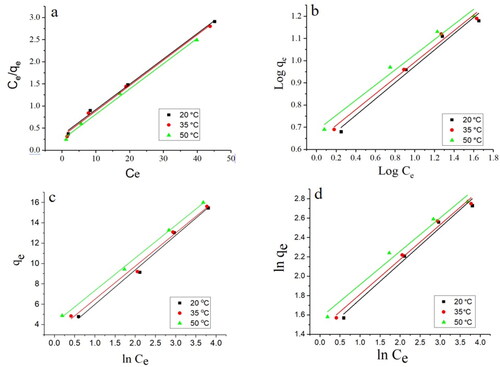
The isotherm parameters were calculated from the graphs of isotherm models and tabulated in .
Table 1. The isotherm parameters of Pb(II) adsorption at various temperature.
The superior R2 values and inferior residual sum of squares (RSS) of Pb(II) served as evidence that the Langmuir isotherm model was the most appropriate and best fitted in the adsorption data as compared to Freundlich, Temkin, and Halsey isotherms models. This model is based on Langmuir’s isotherm, which that predicts a rapid dissipation of intermolecular interactions between adsorbate molecules over time, resulting in a monolayer of adsorbate molecules covering surfaces of adsorbent particles [Citation43]. The Langmuir isotherm was also found to be suitable, and the adsorbed molecules were distributed homogeneously on the surface [Citation43]. It was concluded from the study that Pb(II) ions exhibited monolayer adsorption on Ch-MNP surfaces. In addition, the Ch-MNP surfaces showed homogeneity of structure, with adsorption sites that were identical and equivalent in size.
According to [Citation44], Langmuir isotherm was the best fit for the adsorption of Pb (II) from wastewater using hydroxyapatite nanoparticles. As compared to Freundlich isotherms [Citation45], found the Langmuir isotherm to be the best fit to the Pb(II) adsorption by nanoadsorbents based on silica.
3.5.3. Kinetics
In the present study, an investigation was carried out on the adsorption of Pb(II) utilizing various kinetic models, namely the pseudo-first-order, pseudo-second-order, and Elovich kinetic models. The outcomes of the aforementioned study are shown in .
The present investigation involved the calculation of several kinetic parameters for Pb(II) adsorption onto Ch-MNPS. The kinetic parameters, namely Ks, qe, k′2, qe′, α, β, and R2, were estimated by analysing the slope and intercept of various kinetic models, and the calculated values are comprehensively tabulated in .
Table 2. Kinetics of Pb(II) adsorption.
It has been demonstrated in that the pseudo-second-order kinetic model best fits the adsorption data in Pb(II) based on the higher R2 and lower RSS values, as shown in . Results indicated towards chemisorption. An examination of the adsorption of Pb(II) to copper oxide nanoparticles was carried out by [Citation46] who examined the kinetics of the adsorption using copper oxide nanoparticles. For fitting the adsorption data accurately, pseudo-second-order kinetics was found to be the most accurate model. Ceria nanoparticles also exhibited adsorption potential for lead (II) and mercury (II), as studied by Sharma et al. [Citation47]. Based on the experimental results, the pseudo-second-order kinetic model was found to be the best fit.
3.5.4. Thermodynamics
In order to ascertain the thermodynamic parameters of interest, namely ΔS° and ΔH°, a plot was generated correlating lnkc with 1/T, from which the intercept and slope were utilized to determine thermodynamic parameters ().
The thermodynamic parameters are calculated and listed in .
Table 3. Thermodynamic data for adsorption of Pb(II) on to Ch-MNPs.
The thermodynamic parameters of Pb(II) showed negative ΔG°, positive ΔH° and ΔS°. The negative ΔG and a positive ΔH° showed spontaneous endothermic adsorption of Pb(II). Additionally, ΔG° became more negative with an increase in temperature from 20 to 50 °C, which revealed the favourable adsorption of Pb(II) at higher temperatures. The positive ΔS° revealed increased randomness at Pb(II) solution Ch-MNPs interface [Citation45]. conducted a study on the adsorption of Pb(II) using nano-silica spheres. Authors found that ΔG° was negative and ΔH° was positive, indicating endothermic and spontaneous adsorption. A study demonstrated spontaneous adsorption of Pb(II) by SnO2 and WO3 nanoparticles.
3.6. Comparison Pb(II) removal capacity
The Pb(II) removal efficacy of Ch-MNPs has been compared with other nanomaterials in .
Table 4. Comparison of Pb(II) removal capacity of Ch-MNPs with other nanoparticles.
Although the adsorption capacities have been obtained under diverse experimental conditions and various adsorption mechanisms, they provide valuable perspectives into the selection of nanoparticles for ongoing industrial processes at a significant magnitude. It is proved that the Ch-MNPs exhibit a Pb(II) removal capacity that is either superior or equal to the majority of the adsorbent listed in .
3.7. Desorption of Pb(II) ions and regeneration of Ch-MNPs
The utilization of adsorbent in frequent cycles of adsorption–desorption is deemed more feasible and advantageous in practicality. About 0.1 N nitric acid was used as an eluent in the revival process of Ch-MNPs. shows a schematic representation of the adsorption and desorption cycles.
The data presented in indicated that the percentage of adsorption of Pb(II) decreased significantly from 92.77% to 45.56% throughout five adsorption–desorption cycles. The reduction in performance can be ascribed to the diminution of mass and inactivation of active sites of Ch-MNPs due to iterative cleansing and elution procedures, as well as the inability of the eluting reagent to completely desorb all the adsorbed Pb(II) ions. Despite the observed high percentage of desorption of Pb(II) ranging from 91.45% to 46.43% in the complete hysteresis loop, it was not found to be complete, as the strong binding of heavy metal ions with Ch-MNPs hindered the process by preventing complete detachment. Following three cycles of desorption efficiency of Cr(VI) and Pb(II) on groundnut husk, Bayuo et al. [Citation53] reported a reduction of 20.0% and 26.70%, respectively. Additionally, Pandey et al. [Citation54] observed a decrease in desorption of up to 3%, 14%, and 15% of Cr(VI), Pb(II), and Cu(II) on calcium alginate. The findings of the desorption investigation indicate that the Ch-MNPs exhibit a commendable capacity for rejuvenation and extended durability across multiple rounds of adsorption–desorption [Citation55–57].
4. Recommendations for future studies
The present study has demonstrated intriguing outcomes regarding the elimination of Pb(II) by Ch-MNPs from the liquid phase. In the future, it is possible to further investigate Ch-MNPs’ ability to remove other hazardous metal ions, including As, Cd, Ni, Hg, and Cu. Developing a cost-effective, energy-efficient, and environmentally friendly wastewater treatment system can also be achieved through the use of advanced technical tools for assessment, such as life cycle assessment, exergo-environmental studies, and exergo-economic assessments.
5. Conclusion
The present study involved the utilization of C. limetta peel powder and CE for the synthesis of Ch-MNPs. It was observed that Ch-MNPs exhibited a rough surface, a spherical shape, modest dimensions ranging from 14 to 25 nm and distinct crystallization characteristics. To confirm carboxyl, hydroxyl, and amino group presence, FTIR analysis was performed. Furthermore, EDX analysis and elemental mapping were employed to validate the existence of various elements such as Mn, O, C, H, and N. EDX and elemental mapping were used to determine the adsorption of Pb(II) ions. Pseudo-second-order kinetic model (R2 = 0.99) and Langmuir isotherm (R2 = 0.99) were found to be more accurate in fitting the Pb(II) adsorption on Ch-MNPs. The Pb(II) adsorption was spontaneous and endothermic. Ch-MNPs exhibited superior adsorption capacities and proved to be an effective and potent scavenger of Pb(II). In the present study, the removal of 92.77% of Pb(II) from water was achieved via the utilization of Ch-MNPs.
CRediT authorship contribution statement
Veer Singh: Performed experiments, Writing original draft preparation including figures and Conceptualization. Nidhi Singh and Sachchidanand Rai, Vivek K. Chaturvedi and Santosh K. Singh: Reviewing and Editing. Vishal Mishra, Emanuel Vamanu and Ashish Kumar: Supervision, Writing- Reviewing and Editing.
ynan_a_2347804_sm3049.docx
Download MS Word (13.1 KB)Acknowledgements
It is our sincere gratitude to the IIT (BHU), Varanasi and ICMR-RMRIMS, Patna for providing the necessary facilities for this study. Dr. Veer Singh thanks the Department of Health Research (DHR) for granting the Young Scientist Award (File No.R.12014/37/2022-HR).
Disclosure statement
No potential conflict of interest was reported by the author(s).
Conflicts of interest
The authors report no conflicts of interest.
References
- Date M, Patyal V, Jaspal D, et al. Zero liquid discharge technology for recovery, reuse, and reclamation of wastewater: a critical review. J Water Process Eng. 2022;49:103129. doi: 10.1016/j.jwpe.2022.103129.
- Date M, Jaspal D. Catalytic degradation of ammonia and nitrate from wastewater a critical review. Adv Environ Technol. 2022;8(3):239–253.
- Date M, Jaspal D, Patel UR. Synthesis and performance of bimetallic poly vinyl alcohol beads in electrocatalytic denitrification. Mater Today: Proc. 2022;71:186–190. doi: 10.1016/j.matpr.2022.08.403.
- Herawati N, Suzuki S, Hayashi K, et al. Cadmium, copper and zinc levels in rice and soil of Japan, Indonesia and China by soil type. Bull Environ Contam Toxicol. 2000;64(1):33–39. doi: 10.1007/s001289910006.
- Flora SJS, Flora GJS, Saxena G. Environmental occurrence, health effects and management of lead poisoning. In: Cascas SB, Sordo J, editors. Lead: chemistry, analytical aspects, environmental impacts and health effects. Netherlands: Elsevier Publication; 2006. p. 158–228.
- Centers for Disease Control and Prevention (CDC). Managing elevated blood lead levels among young children: recommendations from the advisory committee on childhood lead poisoning prevention. 2001. [cited 2020 Mar]. Available from: https://www.cdc.gov/nceh/lead/casemanagement/managingEBLLs.pdf
- Järup L. Hazards of heavy metal contamination. Br Med Bull. 2003;68(1):167–182. doi: 10.1093/bmb/ldg032.
- Ayeni O. Assessment of heavy metals in wastewater obtained from an industrial area in Ibadan, Nigeria. RMZ – Mater Geoenviron. 2014;61:19–24.
- Li R, Hu D, Hu K, et al. Coupling adsorption-photocatalytic reduction of Cr(VI) by metal-free N-doped carbon. Sci Total Environ. 2020;704:135284. doi: 10.1016/j.scitotenv.2019.135284.
- Ali EH, Hashe M. Removal efficiency of the heavy metals Zn(II), Pb(II) and Cd(II) by Saprolegnia delica and Trichoderma viride at different pH values and temperature degrees. Mycobiology. 2007;35(3):135–144. doi: 10.4489/MYCO.2007.35.3.135.
- Fang C, Achal V. The potential of microbial fuel cells for remediation of heavy metals from soil and water-review of application. Microorganisms. 2019;7(12):697–709. doi: 10.3390/microorganisms7120697.
- Igiri BE, Okoduwa SIR, Idoko GO, et al. Toxicity and bioremediation of heavy metals contaminated ecosystem from tannery wastewater: a review. J Toxicol. 2018;2018:2568038. doi: 10.1155/2018/2568038.
- Jobby R, Jha P, Yadav AK, et al. Biosorption and biotransformation of hexavalent chromium [Cr(VI)]: a comprehensive review. Chemosphere. 2018;207:255–266. doi: 10.1016/j.chemosphere.2018.05.050.
- Gadd GM. Geomycology: biogeochemical transformations of rocks, minerals, metals and radionuclides by fungi, bioweathering and bioremediation. Mycol Res. 2007;111(Pt 1):3–49. doi: 10.1016/j.mycres.2006.12.001.
- Zhang R, Leiviskä T. Surface modification of pine bark with quaternary ammonium groups and its use for vanadium removal. Chem Eng J. 2020;385:123967–123977. doi: 10.1016/j.cej.2019.123967.
- Singh V, Singh J, Mishra V. Sorption kinetics of an eco-friendly and sustainable Cr(VI) ion scavenger in a batch reactor. J Environ Chem Eng. 2021;9(2):105125. doi: 10.1016/j.jece.2021.105125.
- Gnanasangeetha D, Saralathambavani D. One pot synthesis of zinc oxide nanoparticles via chemical and green method. J Mater Sci Res. 2013;1:1–8.
- Souri M, Hoseinpour V, Ghaemi N, et al. Procedure optimization for green synthesis of manganese dioxide nanoparticles by Yucca gloriosa leaf extract. Int Nano Lett. 2019;9(1):73–81. doi: 10.1007/s40089-018-0257-z.
- Kumar MN. A review of chitin and chitosan applications. React Funct Polym. 2000;30:1–27.
- Kaveeshwar AR, Sanders M, Ponnusamy SK, et al. Chitosan as a biosorbent for adsorption of iron (II) from fracking wastewater. Polym Adv Technol. 2018;29(2):961–969. doi: 10.1002/pat.4207.
- Youssef AM, Abdel-Aziz ME, El-Sayed ESA, et al. Morphological, electrical & antibacterial properties of trilayered Cs/PAA/PPy bionanocomposites hydrogel based on Fe3O4-NPs. Carbohydr Polym. 2018;196:483–493. doi: 10.1016/j.carbpol.2018.05.065.
- Torrik E, Soleimani M, Ravanchi MT. Application of kinetic models for heavy metal adsorption in the single and multicomponent adsorption system. Int J Environ Res. 2019;13(5):813–828. doi: 10.1007/s41742-019-00219-3.
- Tian W, Wang D, Fan H, et al. A plasma biochemical analysis of acute lead poisoning in a rat model by chemometrics-based fourier transform infrared spectroscopy: an exploratory study. Front Chem. 2018;6:261–268. doi: 10.3389/fchem.2018.00261.
- Samrot AV, Shobana N, Sruthi PD, et al. Utilization of chitosan-coated superparamagnetic iron oxide nanoparticles for chromium removal. Appl Water Sci. 2018;8(7):192. doi: 10.1007/s13201-018-0841-4.
- Ye X, Daraio C, Wang C, et al. Room temperature solvent-free synthesis of monodisperse magnetite nanocrystals. J Nanosci Nanotechnol. 2006;6(3):852–856. doi: 10.1166/jnn.2006.135.
- Cheng G, Walker ARH. Transmission electron microscopy characterization of colloidal copper nanoparticles and their chemical reactivity. Anal Bioanal Chem. 2010;396(3):1057–1069. doi: 10.1007/s00216-009-3203-0.
- Guo Y, Guo H, Wang Y, et al. Designed hierarchical MnO2 microspheres assembled from nanofilms for removal of heavy metal ions. RSC Adv. 2014;4(27):14048–14054. doi: 10.1039/C4RA01044B.
- Buccolieri A, Serra A, Maruccio G, et al. Synthesis and characterization of mixed iron–manganese oxide nanoparticles and their application for efficient nickel ion removal from aqueous samples. J Anal Methods Chem. 2017;2017:9476065–9476069. doi: 10.1155/2017/9476065.
- Sarma GK, Gupta SS, Bhattacharyya KG. Nanomaterials as versatile adsorbents for heavy metal ions in water: a review. Environ Sci Pollut Res Int. 2019;26(7):6245–6278. doi: 10.1007/s11356-018-04093-y.
- Hu P, Wang J, Huang R. Simultaneous removal of Cr(VI) and Amido black 10B (AB10B) from aqueous solutions using quaternized chitosan coated bentonite. Int J Biol Macromol. 2016;92:694–701. doi: 10.1016/j.ijbiomac.2016.07.085.
- Liu L, Fan S. Removal of cadmium in aqueous solution using wheat straw biochar: effect of minerals and mechanism. Environ Sci Pollut Res Int. 2018;25(9):8688–8700. doi: 10.1007/s11356-017-1189-2.
- Jaganyi D, Altaf M, Wekesa I. Synthesis and characterization of whisker-shaped MnO2 nanostructure at room temperature. Appl Nanosci. 2013;3(4):329–333. doi: 10.1007/s13204-012-0135-3.
- Cherian E, Rajan A, Baskar G. Synthesis of manganese dioxide nanoparticles using co-precipitation method and its antimicrobial activity. Int J Mod Sci Technol. 2016;1:17–22.
- Tho PT, Van HT, Nguyen LH, et al. Enhanced simultaneous adsorption of As(III), Cd(II), Pb(II) and Cr(VI) ions from aqueous solution using cassava root husk-derived biochar loaded with ZnO nanoparticles. RSC Adv. 2021;11(31):18881–18897. doi: 10.1039/d1ra01599k.
- Mahmoud ME, Ibrahim GAA, Abdelwahab MS. Manganese dioxide nanoparticles decorated with chitosan for effective removal of lead and lanthanum ions from wastewater by microwave sorption technique. Mater Sci Eng. 2021;267:115091. doi: 10.1016/j.mseb.2021.115091.
- Farghali AA, Bahgat M, Allah AE, et al. Adsorption of Pb(II) ions from aqueous solutions using copper oxide nanostructures. J Basic Appl Sci. 2013;2(2):61–71. doi: 10.1016/j.bjbas.2013.01.001.
- Cardoso P, Erwin TL, Borges AV, et al. The seven impediments in invertebrate conservation and how to overcome them. Biol Conserv. 2011;144(11):2647–2655. doi: 10.1016/j.biocon.2011.07.024.
- Gaur N, Kukreja A, Yadav M, et al. Adsorptive removal of lead and arsenic from aqueous solution using soya bean as a novel biosorbent: equilibrium isotherm and thermal stability studies. Appl Water Sci. 2018;8(4):98. doi: 10.1007/s13201-018-0743-5.
- Senthilkumaar S, Kalaamani P, Porkodi K, et al. Adsorption of dissolved from aqueous phase on to activated carbon prepared from agricultural waste. Bioresour Technol. 2006;97(14):1618–1625. doi: 10.1016/j.biortech.2005.08.001.
- Fato FP, Li DW, Zhao LJ, et al. Simultaneous removal of multiple heavy metal ions from river water using ultrafine mesoporous magnetite nanoparticles. ACS Omega. 2019;4(4):7543–7549. doi: 10.1021/acsomega.9b00731.
- Moyo M, Chikazaza L, Nyamunda BC, et al. Adsorption batch studies on the removal of Pb(II) using maize tassel based activated carbon. J Chem. 2013;2013:1–8. doi: 10.1155/2013/508934.
- Parvathi K, Nagendran R. Biosorption of chromium from effluent generated in chrome‐electroplating unit using Saccharomyces cerevisiae. Sep Sci Technol. 2007;42(3):625–638. doi: 10.1080/01496390601070158.
- Ossman ME, Mansour MS. Removal of Cd(II) ion from wastewater by adsorption onto treated old newspaper: kinetic modeling and isotherm studies. Int J Ind Chem. 2013;4(1):13–19. doi: 10.1186/2228-5547-4-13.
- Safatian F, Doago Z, Torabbeigi M, et al. Lead ion removal from water by hydroxyapatite nanostructures synthesized from egg sells with microwave irradiation. Appl Water Sci. 2019;9(4):1–6. doi: 10.1007/s13201-019-0979-8.
- Manyangadze M, Chikuruwo NMH, Narsaiah TB, et al. Adsorption of lead ions from wastewater using nano silica spheres synthesized on calcium carbonate templates. Heliyon. 2020;6(11):e05309. doi: 10.1016/j.heliyon.2020.e05309.
- Zaidi R, Khan SU, Azam A, et al. A study on effective adsorption of lead from an aqueous solution using copper oxide nanoparticles. IOP Conf Ser: Mater Sci Eng. 2021;1058(1):012074. doi: 10.1088/1757-899X/1058/1/012074.
- Sharma R, Raghav S, Nair M, et al. Kinetics and adsorption studies of mercury and lead by ceria nanoparticles entrapped in tamarind powder. ACS Omega. 2018;3(11):14606–14619. doi: 10.1021/acsomega.8b01874.
- Kabbashi NA, Nour AH, Muyibi SA, et al. Development of a wastewater treatment system for chromium removal using chitosan. Int J Chem Technol. 2009;1(2):44–51. doi: 10.3923/ijct.2009.44.51.
- Mostafa NG, Yunnus AF, Elawwad A. Adsorption of Pb(II) from water onto ZnO, TiO2, and Al2O3: process study, adsorption behaviour, and thermodynamics. Adsorp Sci Technol. 2022;2022:7582756. doi: 10.1155/2022/7582756.
- Hassan MR, Aly MI. Magnetically synthesized MnFe2O4 nanoparticles as an effective adsorbent for lead ions removal from an aqueous solution. J Water Supply: Res Technol-Aqua. 2021;70(6):901–920. doi: 10.2166/aqua.2021.132.
- Jiao C, Tan X, Lin A, et al. Preparation of activated carbon supported bead string structure nano zero valent iron in a polyethylene glycol-aqueous solution and its efficient treatment of Cr(VI) wastewater. Molecules. 2019;25(1):47–61. doi: 10.3390/molecules25010047.
- Bizhan Zadeh S, Daghighi M, Torkian L. Preparation, characterization, equilibrium isotherms, and adsorption kinetics of Al2O3–SiO2 nanoparticle for rapid and high adsorption of Pb(II)from water. Int J Pharm Phytopharmacol Res. 2019;9(1):51–57.
- Bayuo J, Abukari MA, Pelig-Ba KB. Desorption of chromium (VI) and lead (II) ions and regeneration of the exhausted adsorbent. Appl Water Sci. 2020;10(7):1–6. doi: 10.1007/s13201-020-01250-y.
- Pandey A, Bera D, Shukla A, et al. Studies on Cr(VI), Pb(II) and Cu(II) adsorption–desorption using calcium alginate as biopolymer. Chem Spec Bioavailab. 2007;19(1):17–24. doi: 10.3184/095422907X198031.
- Vasilescaeteanu G, Sandulescu EB, Alistar CF, et al. A short note on water quality and some biodiversity components in Gurban valley, Giurgiu county. AgroLife Scientific Journal 2023;12(2):167–180. doi: 10.17930/AGL2023222.
- Mitelut AC, Danaila-Guidea SM, Popescu SA, et al. Use of hydrogels as a sustainable solution for water and nutrients management in plant culture. Scientific Bulletin-Series F. Biotechnologies 2022;26(2):73–84.
- Vamanu E, Dangnon DB. Characterizing water kefir beverages with antioxidant effects: preliminary analysis. Scientific Bulletin-Series F. Biotechnologies 27(2):98–104.