ABSTRACT
The C-terminal domain (CTD) of the largest subunit of RNA polymerase II (Pol II) consists of YSPTSPS heptapeptide repeats, and the phosphorylation status of the repeats controls multiple transcriptional steps and co-transcriptional events. However, how CTD phosphorylation status responds to distinct environmental stresses is not fully understood. In this study, we found that a drastic reduction in phosphorylation of a subset of Ser2 residues occurs rapidly but transiently following exposure to H2O2. ChIP analysis indicated that Ser2-P, and to a lesser extent Tyr1-P was reduced only at the gene 3’ end. Significantly, the levels of polyadenylation factor CstF77, as well as Pol II, were also reduced. However, no increase in uncleaved or readthrough RNA products was observed, suggesting transcribing Pol II prematurely terminates at the gene end in response to H2O2. Further analysis found that the reduction of Ser2-P is, at least in part, regulated by CK2 but independent of FCP1 and other known Ser2 phosphatases. Finally, the H2O2 treatment also affected snRNA 3’ processing although surprisingly the U2 processing was not impaired. Together, our data suggest that H2O2 exposure creates a unique CTD phosphorylation state that rapidly alters transcription to deal with acute oxidative stress, perhaps creating a novel “emergency brake” mechanism to transiently dampen gene expression.
Introduction
In eukaryotic cells, protein-coding genes, as well as genes encoding long non-coding RNAs and mostly small nuclear RNAs (snRNAs) are transcribed by RNA polymerase (Pol) II. The largest subunit of Pol II, Rpb1, contains a unique C-terminal domain (CTD) that consists of repeats of the heptapeptide Tyr-Ser-Pro-Thr-Ser-Pro-Ser. The CTD is evolutionarily conserved and composed of a species-specific number of repeats (e.g., 26 in yeast and 52 in mammals). This unique CTD structure is known to be highly phosphorylated, and its phosphorylation status is dynamically modified by specific kinases and phosphatases during various steps of transcription, such as preinitiation complex assembly, promoter proximal pausing, elongation, termination, and polymerase recycling. Depending on the phosphorylation status of the CTD, various transcription and RNA processing factors are recruited/released to coordinate transcription and RNA processing [for review, see Citation1, Citation2–6].
The maintenance of cellular homeostasis under various types of environmental stress conditions involves highly controlled transcriptional regulatory programs. Many studies have shown that stress-induced transcription factors, which often function by recruiting auxiliary factors such as chromatin modifiers, control the expression of multiple stress responsive genes, which is necessary to deal with multiple stresses [for review, see Citation7–9]. For example, in response to oxidative stress, NRF2 is stabilized and binds to the transcriptional cofactors CBP and p300 to control the expression of a series of genes encoding factors that function in the antioxidant defense system [Citation10,Citation11]. Under heat shock stress, HSF1 senses temperature via conformational changes and interacts with p300 and a cofactor, Strap, to activate target genes, for example those encoding heat shock proteins 70 and 90 [Citation12,Citation13]. How changes in Pol II modification, e.g., CTD phosphorylation, in response to environmental stresses contribute to changes in transcription is not well understood. Interestingly, though, several studies have shown that modification of Pol II can in fact change in response to stress. For example, Rpb1 is known to be ubiquitylated and degraded in response to UV exposure or exposure to high concentrations of H2O2 [Citation14–17]. CTD Tyr1 phosphorylation was shown to be required for proper expression of stress-related genes in budding yeast [Citation18]. These reports suggest that modifications of Pol II itself, including the phosphorylation state of the CTD, can be important in controlling global gene expression in response to various stresses.
Phosphorylation of Ser2 is one of the most extensively studied CTD modifications [Citation19]. Ser2 phosphorylation (Ser2-P) levels change dramatically during transcription, and Ser2-P is involved in multiple steps in the transcriptional process, such as Pol II pause release [Citation20,Citation21], splicing [Citation22,Citation23], polyadenylation [Citation24,Citation23,Citation25,Citation22], histone modification [Citation26,Citation27] and snRNA processing [Citation28,Citation29]. To control all these diverse functions properly, Ser2-P frequently works in conjunction with other modifications. For example, Tyr1-P prevents recruitment of termination factor Pcf11, which is otherwise facilitated by Ser2-P [Citation30]. Both Ser2-P and Ser5-P are important for proper splicing by enhancing recruitment of splicing components such as U2AF65 and U2 snRNP to transcription sites [Citation22,Citation31] and interaction of the CTD with the spliceosome during co-transcriptional splicing [Citation32]. Furthermore, double phosphorylation of Ser2 and Ser7 helps recruit the Integrator complex, which functions in snRNA 3ʹ processing [Citation33,Citation34]. The above examples show that precise control of the phosphorylation state of the CTD is essential for proper transcriptional regulation, which is controlled by coordinated actions of CTD kinases and phosphatases.
Several kinases are known to modify Ser2. On most genes, Ser2 is phosphorylated by the cyclin-dependent kinase CDK9, a subunit of P-TEFb, a modification that is important for Pol II release from promoter pausing and elongation [Citation35]. BRD4, which can function to activate P-TEFb, is also itself an atypical kinase that phosphorylates Ser2, and which can facilitate release of Pol II from promoter pausing via activating topoisomerase I to resolve torsional stress [Citation36–38]. CDK-11, −12 and −13 are also all able to phosphorylate Ser2. For example, CDK11 can phosphorylate Ser2 on histone genes and during HIV viral transcription, facilitating 3ʹ end processing [Citation39,Citation40]. CDK12 and its paralog CDK13 are also known to phosphorylate Ser2 and globally function as transcription elongation factors [Citation41–46]. Finally, DYRK1A is reported to phosphorylate Ser2 and Ser5 and regulate expression of growth-related genes [Citation47].
Phosphatases that target Ser2, on the other hand, appear to be more limited in number. FCP1 (TFIIF-associated CTD phosphatase) was the first identified CTD-specific phosphatase [Citation48,Citation49] and has been extensively characterized [Citation50,Citation51]. FCP1 has been shown to be regulated by the general transcription factors TFIIF (RAP74) and TFIIB, and casein kinase 2 (CK2). FCP1 is recruited to the Pol II complex via docking sites on the Rpb4 subunit and on the RAP74 subunit of TFIIF, an interaction that is important to control FCP1 phosphatase activity [Citation52,Citation53]. In addition, the phosphorylation of FCP1 by CK2 stimulates FCP1 activity and enhances the binding of RAP74 to FCP1 [Citation54, Citation55].
Subsequent to the characterization of FCP1 as a Ser2 phosphatase, many attempts have been made to find other CTD phosphatases. For example, SCP1/2/3 were identified as proteins containing regions similar to the FCP1 homology domain, but SCP1 is more likely to dephosphorylate Ser5 than Ser2, and appears to function only on several neural genes [Citation56,Citation57]. HSPC129 and UBLCP1 are two other members of the FCP1 family and have been reported to have CTD phosphatase activity in vitro, although their activity in vivo has not been characterized [Citation58,Citation59]. Additionally, there are other CTD phosphatases, such as SSU72 and RPAP2, but they do not function on Ser2 [Citation60–63]. More recently, PP2A, which interacts with the Integrator, has been identified as a Ser2, Ser5, and Ser7 phosphatase [Citation64,Citation65].
In this study, we initially set out to examine possible changes in CTD phosphorylation in response to several different stresses. Strikingly, we found that Ser2 phosphorylation was specifically, rapidly and transiently eliminated in response to oxidative (H2O2) stress. This acute decrease of Ser2-P causes dissociation of both Pol II and the mRNA 3ʹ processing factor CstF77 at the end of the gene, likely reflecting premature transcription termination and Pol II dissociation. 3ʹ processing of U1, U4, and U5 snRNAs was also disrupted by H2O2, although the U2 snRNA was unaffected. Finally, CK2 inhibition attenuated the decrease of Ser2-P, pointing to an alternate pathway not involving either FCP1 or PP2A. Together, our results suggest a novel mechanism involving CTD phosphorylation by which cells can rapidly and reversibly alter gene expression in response to a specific stress.
Results
Oxidative stress reduces Ser2 phosphorylation levels
To investigate whether differential CTD modification might contribute to the cellular response to stress, we first determined whether several stresses, specifically exposure to H2O2, UV irradiation, or heat shock, can affect CTD phosphorylation. To this end, we first performed Western blotting (WB) using multiple antibodies specific to Rpb1 and phosphorylated Tyr1, Ser2, Thr4, Ser5, and Ser7. We used two types of antibodies for phosphorylated Ser2. 3E10 preferentially recognizes solely phosphorylated Ser2, while H5 is more specific for Ser2-P in the context of neighboring Ser5-P [Citation66,Citation67]. Whole-cell lysates were prepared from HeLa cells exposed to the different stresses as indicated in . γH2AX levels were measured as a DNA damage marker, and actin was used as loading control. Strikingly, the Ser2-P signal detected by 3E10 but not by H5, was sharply decreased after H2O2 exposure for 1 hour (). While increased γH2AX levels were observed in both H2O2-treated and UV-exposed samples, reduction of Ser2-P (3E10) was only observed in the H2O2-treated cells, suggesting that this acute reduction of Ser2-P did not arise as a consequence of elevated DNA damage or repair. Tyr1-P levels also appeared to be modestly reduced by the H2O2 treatment, but the phosphorylation status of the other CTD residues was not significantly affected.
Figure 1. Acute reduction of Ser2 phosphorylation levels by H2O2 exposure. Western blots of whole-cell lysates prepared from cells treated as indicated. Blots were probed with the antibodies indicated on the right. (a) HeLa cells were subjected to the stress indicated on the top for the indicated time. (b) HeLa cells were incubated with 0.5 mM H2O2 for the indicated times. (c) HeLa cells were incubated with the indicated concentrations of H2O2 for 1 hour. (d) U87, MCF7, and DT40 cells were treated with the indicated concentrations of H2O2 for 1 hour.
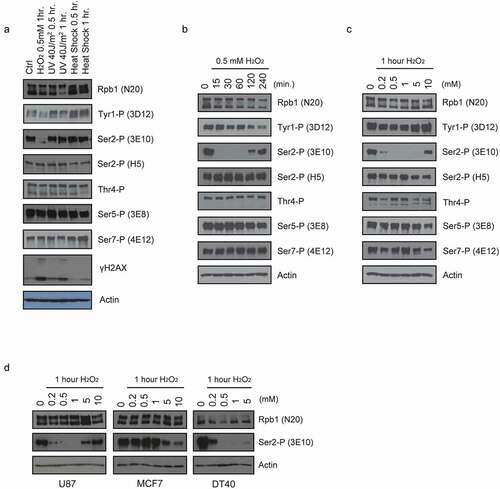
The above results led us to examine CTD phosphorylation levels following H2O2 treatment in more detail. We first performed a time course in which HeLa cells were treated with 0.5 mM H2O2 for different times and the lysates were analyzed by WB exactly as in . Remarkably, the Ser2-P (3E10) signal completely disappeared after only 15-minute H2O2 treatment, but began to recover after 120 minutes and was almost fully restored after 4 hours (). Tyr1-P levels decreased modestly over the entire time course, but none of the other residues, including Ser2-P detected by H5, appeared to be affected. Next, HeLa cells were treated with a range of H2O2 concentrations, from 0 to 10 mM, for 1 hour, then lysates were prepared and analyzed by WB as above (). Ser2-P levels were almost eliminated at the lowest concentration tested, 0.2 mM, and were undetectable from 0.5 mM to 5 mM. Interestingly, however, at the highest dose, 10 mM, Ser2-P levels were only slightly reduced relative to the control. Tyr1-P levels appeared modestly reduced although also rose at 10 mM, while the other residues paradoxically seemed largely unaffected but declined somewhat at 10 mM. Together our results indicate that H2O2 can cause dramatic and highly specific, but transient, decreases in Ser2 phosphorylation.
We next tested whether H2O2-induced loss of Ser2-P occurs in other cell types. We measured the effect of H2O2 exposure on Ser2-P (3E10) in human glioblastoma U87 cells, human MCF7 breast cancer cells, and chicken DT40 lymphoma cells. Cells were treated with the indicated concentrations of H2O2 for 1 hour, then Ser2-P levels were measured by WB (). Similar to what we observed in HeLa cells, Ser2-P (3E10) levels were sharply reduced in U87 and DT40 cells. Unexpectedly, the effect in MCF7 cells was modest and observed only at high H2O2 concentrations, much like observed with most other CTD residues in HeLa cells (see ). This result suggests that loss of Ser2-P in response to H2O2 is conserved at least in vertebrates but depends on cellular context.
Oxidative stress-induced loss of Ser2-P affects promoter-distal events in the transcription cycle
We next investigate how the H2O2-induced decrease in Ser2-P might affect the Pol II function. We first examine the effects of two well-studied Ser2 kinase inhibitors on the Ser2-P pattern in comparison with the H2O2 treatment. HeLa cells were treated with 0.5 mM H2O2 or with either of two inhibitors, 5,6-dichloro-1-β-d-ribofuranosylbenzimidazole (DRB), or flavopiridol (Flavo), both of which inhibit CDK9 kinase activity in P-TEFb, resulting in inhibition of the transition from transcription initiation to elongation [Citation68,Citation69]. After treatment for 1 hour, cells were collected, and lysates were analyzed by WB with some of the antibodies used above (). As shown above, the signals obtained with H5 were not affected by the H2O2 treatment, whereas 3E10 signals were drastically reduced. In sharp contrast, treatment with either of the two CDK9 inhibitors sharply reduced H5 signals but had no effect on the 3E10 reactivity. As expected, Ser5-P levels were unaffected. These results indicate that the effects of H2O2 on Ser2 phosphorylation do not reflect inhibition of CDK9/P-TEFb, and also suggest that loss of Ser2-P occurs further downstream in the transcription cycle than the initiation of the elongation transition controlled by P-TEFb. It is also notable that neither inhibitor affected Ser2-P levels (3E10) under these conditions (1 hour) but only reduced Ser2-P/Ser5-P (H5). This is consistent with the view that CDK9/P-TEFb functions primarily in promoter-proximal regions, where Ser2-P/Ser5-P is most abundant [Citation2]. Reduced 3E10 reactivity has been observed after longer times, however [Citation70].
Figure 2. H2O2 and CDK9 inhibitors affect Ser2 phosphorylation and transcription/ 3’ processing differently. (a) Western blot of whole-cell lysates from Hela cells treated with 0.5 mM H2O2, 50 uM DRB (5,6-dichloro-1-b-D-ribofuranosylbenzimidazole) or 0.5 uM Flavopiridol for 1 hour. Blots were probed with the antibodies indicated on the right. (b, c) RT-qPCR. Diagrams at the top depict analyzed genes. Positions and strand directions of primers used in RT-qPCR to detect nascent, spliced and uncleaved RNAs are indicated by arrowheads. The graphs display the RT-qPCR data from RNA isolated from HeLa cells treated as in (A) with the compound indicated on the right, normalized to corresponding data from control cells. Values shown are the mean ± s.d. of n = 3 independent replicates.
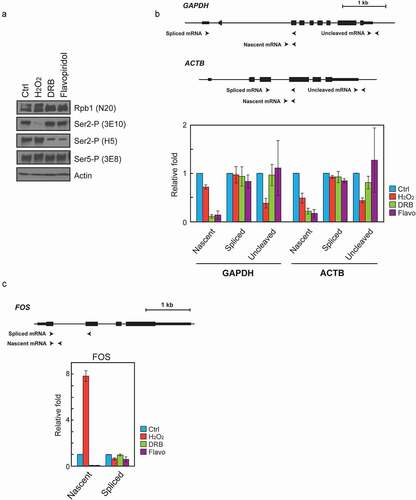
To examine further how/if H2O2 affects transcribing Pol II, we next measured nascent and spliced (mature) mRNA levels from three genes, GAPDH, ACTB, and FOS, using RT-qPCR. Nascent GAPDH transcript levels, as measured with primers spanning a splice junction, were sharply reduced by treatment with either of the two CDK9 inhibitors, while H2O2 treatment caused only a modest reduction in this species (). Similarly, nascent ACTB transcript levels were drastically reduced by treatment with either of the two CDK9 inhibitors. Interestingly, however, the H2O2 treatment reduced ACTB nascent transcript levels more significantly, by ~50% (). This might reflect the locations of primers for the detection of nascent ACTB transcripts, which were targeted to the middle of the gene compared to the more upstream primers for GAPDH (, top; see also below). Spliced transcript levels of GAPDH and ACTB transcripts were unchanged at this time point (). With FOS, addition of the CDK9 inhibitors also drastically reduced nascent mRNA levels, whereas H2O2 exposure strikingly increased it, as reported previously [Citation71]. As with the other two genes, the spliced FOS mRNA levels were essentially unchanged (). These results support the conclusion that H2O2-induced loss of Ser2-P likely did not affect Ser2 phosphorylation by CDK9/P-TEFb, and that any effects on transcription occur downstream of the transition from initiation to elongation.
We next examined whether H2O2 exposure indeed affects downstream events, such as 3ʹ cleavage. Ser2-P is known to be important for efficient 3ʹ end processing, and importantly solely phosphorylated Ser2 (i.e., recognized by the 3E10 antibody) is generally detected at gene 3ʹ ends [Citation25,Citation66]. To investigate this question, we first measured levels of 3ʹ -uncleaved RNAs transcribed from the GAPDH and ACTB genes, by using RT-qPCR primers that span the 3ʹ processing site. The results showed that H2O2 treatment substantially decreased levels of uncleaved transcripts relative to the untreated control (). This was somewhat surprising given that a lack of Ser2-P would be expected to reduce cleavage and thus increase the amount of uncleaved transcripts, as has previously been reported [Citation22–24]. Notably, neither of the CDK9 inhibitors had a significant effect on the amount of uncleaved RNA relative to the control (). This result is also somewhat unexpected given the large effect of these inhibitors on the accumulation of nascent (unspliced) transcripts. This may largely reflect the very low absolute levels of these transcripts, which may have arisen from transcription initiated prior to addition of the inhibitors. (ΔCT values of each amplicon are shown in Supplemental Figure 1.) In any case, these data are consistent with the possibility that H2O2 exposure results in rapid dephosphorylation of Ser2 on elongating Pol II, leading to stalling or premature termination, preventing the transcribing Pol II from reaching the end of the gene.
To examine more directly the possibility that H2O2 exposure leads to premature termination of transcription, we next performed ChIP assays on the GAPDH gene locus (). After 1-hour H2O2 treatment, treated and controlled HeLa cells were fixed, and lysates were prepared for ChIP assays with several different antibodies. ChIP analysis using 3E10 (for Ser2-P) revealed only low levels of reactivity along the length of the gene in both controlled and H2O2-treated samples (). In the control samples, levels increased dramatically around the poly(A) site until ~500 bp downstream and then decreased. In contrast, 3E10 levels remained low along the entire length of the gene in the H2O2-treated samples, failing to increase as observed with the controls. ChIP analysis using the N20 antibody (for total Rpb1) showed very high signals just downstream of the promoter (5ʹ pause) and low but significant signals along the length of the gene in both controlled and treated samples (). Importantly though N20 signals in the treated cells were significantly reduced relatively to the control towards the end of the gene. Notably, the reduction in Rpb1 levels was much less than the decrease in Ser2-P, indicating that the bulk of the Pol II remaining on the gene is devoid of Ser2-P. The reduction in Rpb1 levels was though similar in magnitude to the decrease in uncleaved RNA detected in the H2O2-treated samples (see ), supporting the idea that the decrease in RNA reflects premature termination by a fraction of the elongating Pol II. This premature termination seems to occur towards the end of the gene, as the reduction of the N20 signal begins only after the body 2 primer set (shown in ). Consistent with this idea, the nascent mRNA levels detected in ACTB were more reduced by H2O2 exposure than they were by GAPDH (). As noted above, this may reflect the fact that the primers for detecting ACTB nascent mRNA were relatively further downstream on the gene compared to the primers used for GAPDH.
Figure 3. H2O2 decreases Ser2-P levels and Pol II and PA factor occupancy at the gene 3’ end. (a) Schematic illustration of the human GAPDH gene. Positions of amplicons are indicated. (b-e) ChIP assays for Ser2-P, Rpb1, Tyr1-P and CSTF77, respectively. HeLa cells were either untreated (Ctrl) or treated with 0.5 mM H2O2 for 1 hour prior to addition of formaldehyde and preparation of cell lysates. Results are presented as the percent of input signal. Values shown are the mean ± s.e. [n = 5 for Rpb1; n = 3 for Ser2-P, Tyr1-P and CSTF77]. The P value was determined by two-tailed Student’s t-test. (**p < 0.01, *p < 0.05).
![Figure 3. H2O2 decreases Ser2-P levels and Pol II and PA factor occupancy at the gene 3’ end. (a) Schematic illustration of the human GAPDH gene. Positions of amplicons are indicated. (b-e) ChIP assays for Ser2-P, Rpb1, Tyr1-P and CSTF77, respectively. HeLa cells were either untreated (Ctrl) or treated with 0.5 mM H2O2 for 1 hour prior to addition of formaldehyde and preparation of cell lysates. Results are presented as the percent of input signal. Values shown are the mean ± s.e. [n = 5 for Rpb1; n = 3 for Ser2-P, Tyr1-P and CSTF77]. The P value was determined by two-tailed Student’s t-test. (**p < 0.01, *p < 0.05).](/cms/asset/8406c087-cfa8-44a2-985c-a405678c8c16/ktrn_a_2009421_f0003_oc.jpg)
In our initial WBs, we also observed small decreases in Tyr1-P following the H2O2 treatment. We therefore also performed ChIP assays using the 3D12 Ab for Tyr1-P (). Consistent with previous studies [Citation72–74], a strong Tyr1-P signal was observed at the Pol II pause site and this was similar in both controlled and treated samples. Levels were low across the gene body, but rose modestly at and beyond the poly(A) site in control samples, again consistent with previous findings [Citation72,Citation73]. However, this increase was not observed with the H2O2-treated samples, where Tyr1-P signals were essentially undetectable in this region. This lack of Tyr1-P may also contribute to premature termination [Citation30].
Ser2-P is important for efficient association of the polyadenylation (PA) machinery at gene 3ʹ ends. We therefore tested with ChIP whether levels of a PA factor, CstF77, associated with GAPDH are affected by H2O2 (). We chose CstF77 because strong Ser2-P signals have been shown to coincide with CstF77 levels around poly(A) sites [Citation25]. Signals around the promoter and along the gene were similar between controlled and treated cells. However, while a sharp increase in CstF77 was observed downstream of the poly(A) site in control cells, signals were much lower in the treated cells. This likely reflects both a reduction in the association of CstF77 and other PA factors with Pol II as well as the reduced levels of Pol II reaching the gene 3’ end.
H2O2 blocks 3’ processing of some but not all snRNAs
Ser2-P has also been implicated in 3’ processing of snRNA transcripts. Several studies using CTD mutant models and kinase inhibitors have shown that phosphorylation of the CTD at Ser2 and/or Ser7 is important for snRNA 3’ processing [Citation23,Citation28,Citation29,Citation33,Citation34,Citation75]. We therefore examined whether the H2O2 treatment affects 3’ processing of snRNAs, using RT-qPCR to measure total and uncleaved RNA levels, using the same RNA samples as in and 2c. As previously reported [Citation28,Citation29,Citation75], uncleaved transcript levels of all the snRNAs we tested (U1, U2, U4, and U5) were sharply increased by DRB or Flavo treatment. H2O2 exposure similarly increased uncleaved U1, U4, and U5 snRNA transcripts, but curiously the processing of U2 snRNA was not affected by the H2O2 treatment (). These results not only extend the effects of H2O2 treatment, presumably the loss of Ser2 phosphorylation, to snRNA synthesis, but also suggest a mechanistic difference between U1/U4/U5 and U2 snRNA processing.
Figure 4. H2O2 inhibits U1, U4 and U5 but not U2 snRNA 3’ cleavage. Top, schematic illustrations describing the gene structure of the human snRNA genes. Arrowheads denote the positions of the primers used to amplify total and uncleaved RNA. Vertical arrows indicate the site of 3’ cleavage. Bottom. HeLa cells were treated with the compounds indicated on the right as in . Total RNA was isolated, and expression levels of total and uncleaved U1, U2, U4, and U5 snRNAs were analyzed by RT-qPCR. Expression of 7SK RNA was used as an internal control. The RT-qPCR data from treated cells were normalized to those of the corresponding control cells, and are represented as the mean ± s.d. of n = 3 replicates.
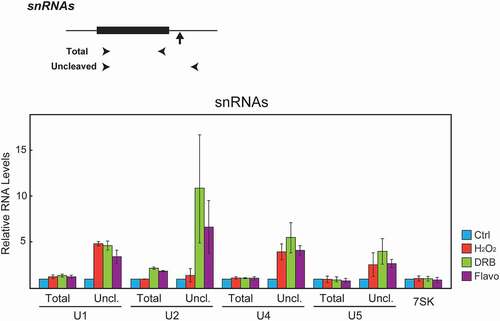
Inhibition of CK2, but not FCP1 or other phosphatases, attenuates oxidative stress-induced Ser2 dephosphorylation
Next, we wish to investigate what underlies the loss of Ser2-P in response to H2O2. Given the rapidity and completeness with which this occurs, it seems most likely that a phosphatase is activated, but it could also reflect repression of Ser2 kinase activity. We first explored the possibility that oxidative stress stimulates a phosphatase activity that leads to Ser2 dephosphorylation and focused on the CK2-FCP1 pathway. CK2 is known to be involved in the oxidative stress response and is activated by stress, including H2O2, as part of the cellular redox system [Citation76]. Notably, CK2 has been reported to activate FCP1 [see Introduction; Citation54, Citation55]. To examine whether CK2 is involved in H2O2-induced Ser2-P loss, we treated HeLa cells with either of two CK2 inhibitors, 4,5,6,7-tetrabromo-1 H-benzotriazole (TBB), or CX-4945 (CX) for 30 minutes, then exposed them to H2O2 for 1 hour. Cell lysates were prepared and analyzed by WB (). Notably, the H2O2-induced reduction in Ser2-P levels was partially attenuated by treatment with either of the CK2 inhibitors, with the effect of TBB being especially strong. Levels of Ser5-P were unaffected as were levels of FCP1. This data suggests that CK2 functions in H2O2-induced loss of Ser2-P. To test whether FCP1 also functions in this process, FCP1-targeting or control siRNAs were transfected into HeLa cells. After 24-hour incubation, cells were exposed to H2O2 for the times indicated, and protein levels were then analyzed by WB (). The data show that despite efficient FCP1 knockdown, H2O2 treatment still led to complete loss of 3E10 reactivity. These results provide strong evidence that H2O2-induced loss of Ser2-P does not require FCP1. We also tested if depletion of other CTD phosphatases such as SCP1, UBLCP1, SSU72 and PRAP2 (see Introduction) prevents H2O2-mediated Ser2-P loss, but none had any effect (data not shown).
Figure 5. CK2, but not FCP1, is involved in H2O2-induced Ser2-P inhibition. (a) Effects of CK2 inhibition on H2O2-induced Ser2-P loss were measured by Western blot. Hela cells were pre-treated with the indicated concentrations of TBB or CX-4945 for 15 min, then treated with 0.5 mM H2O2 for 1 hour. Blots were probed with antibodies indicated on the right. (b) Effect of FCP1 knockdown on H2O2-induced Ser2-P reduction. HeLa cells were transfected with control siRNA and siRNA targeting FCP1 as indicated and cultured for 36 hours. 0.5 mM H2O2 was then added for the times shown, whole-cell lysates prepared and analyzed by Western blot. Antibodies used are indicated on the right.
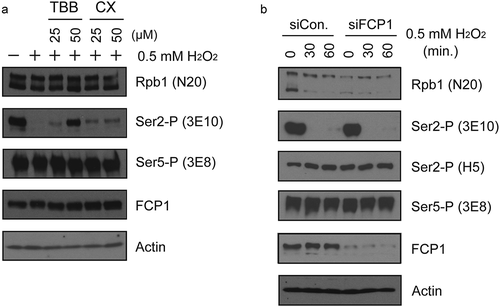
Given that the phosphatase PP2A has also been reported to be capable of dephosphorylating Ser2-P [Citation65], we also tested whether PP2A is involved in H2O2-induced loss of Ser2-P. To this end, we treated HeLa cells with H2O2 and increasing concentrations of the PP1/PP2A inhibitor okadaic acid, and analyzed cell lysates with WB as above (Supplemental Figure 2). Notably, this resulted in increased CTD phosphorylation, as judged both by the increase in the Rbp1 IIo isoform at the expense of IIa and by the increase in H5 (Ser2-P/Ser5-P) and 3E8 (Ser5-P) reactivity. These findings are consistent with the idea that PP2A can function as a CTD phosphatase, functioning early in the transcription cycle [Citation64,Citation65]. Strikingly, however, okadaic acid failed to rescue H2O2-induced loss of Ser2-P (3E10), and indeed even appeared to reduce further the low levels of Ser2-P that remain following H2O2 exposure. Whatever the significance of this additional loss of Ser2-P, these results provide strong evidence that PP2A, like FCP1, is not involved in H2O2-induced Ser2-P loss.
We also further explore the possibility that a Ser2 kinase is repressed by H2O2 and that this results in the observed loss of Ser2-P. As shown above, we have already ruled out the involvement of the major Ser2 kinase, CDK9/P-TEFb. We next asked whether the atypical kinase BRD4 is involved in H2O2-induced Ser-P loss, as CTD phosphorylation by BRD4 can be detected by 3E10 but not H5 [Citation37]. To examine this we utilized the BRD4 inhibitor JQ1, and observed that it had no effect on the Ser2-P levels detected by 3E10 (Supplemental Figure 3), providing strong evidence that BRD4 is not involved in the H2O2 inhibition we observed. Additionally, we found that treatment of HeLa cells with actinomycin D did not affect Ser2-P levels (3E10) and did not prevent loss of Ser2-P by H2O2 (Supplemental Figure 4). These results suggest that the Ser2-P loss is independent of ongoing transcription, which is when Ser2 phosphorylation generally occurs [Citation19].
Together, our data support the idea that an unknown phosphatase, perhaps activated by CK2, is responsible for the rapid loss of Ser2-P induced by H2O2.
Discussion
In this study, we identified a rapid, transient, and highly specific reduction of phosphorylated Ser2 on the Pol II CTD in response to oxidative (H2O2) stress. While a slight reduction in Tyr1-P was also observed, the loss of phosphorylation was otherwise so specific that it was detected only with an antibody with specificity for Ser2-P alone, and not with one that preferentially recognizes Ser2,5-P. As discussed below, this is also indicative that the Ser2-P deficiency occurs in Pol II molecules located toward the 3’ ends of transcribed genes. Consistent with this our data indicates that the rapid loss of Ser2-P likely causes premature transcription termination and Pol II dissociation before reaching the end of the gene (see model in ). SnRNA 3’ processing was also found to be disrupted by H2O2 exposure although unexpectedly one, U2 snRNA, was not affected. Finally, we provide evidence that CK2 is implicated in the loss of Ser2-P, but this is likely independent of known Ser2 phosphatases, such as FCP1 and PP2A. Our study thus describes a novel mode of gene regulation via direct modulation of CTD phosphorylation in response to a specific stress. Below we discuss the significance of our findings and what additional questions they raise.
Figure 6. Model for H2O2-induced premature transcription termination. In response to H2O2-induced oxidative stress, CK2 activates an unidentified phosphatase (phosphatase X) that dephosphorylates Ser2-P only when RNA Pol II is near the 3ʹ end of a transcribing gene. This triggers the release of PA factors such as CstF77 from the elongation complex, as well as RNA Pol II itself, and transcription is prematurely terminated.
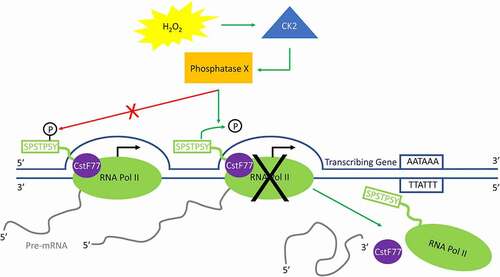
The rapid, complete, and highly specific loss of Ser2-P in response to H2O2 was striking. To our knowledge, such an effect on CTD phosphorylation or Rpb1 modification of any sort, has not been observed previously. Several studies have examined the effects of H2O2 on Rpb1/CTD modification and observed no or less dramatic effects. For example, Hintermair et al. also examined the effects of H2O2 on CTD phosphorylation, but they did not observe effects on Ser2-P even though they also used the 3E10 antibody [Citation77]. Their results thus seem to contradict ours but the treatment times and culture conditions used were substantially different, so the results are not comparable. In another example, Inukai et al. found that treatment of HeLa cells with a high concentration (10 mM) of H2O2, while not leading to a detectable change in CTD phosphorylation levels, resulted, after a recovery period, in phosphorylation of Ser5 by an atypical CTD kinase, ERK1/2, which led to Rpb1 ubiquitination and subsequent degradation [Citation15]. Notably, in our experiments, treatment with this high concentration of H2O2 failed to cause the loss of Ser2-P observed at lower concentrations, although whether this is related to the findings of Inukai et al. is unclear [Citation15]. It was also reported that brief exposure to a low level of H2O2 (15 minutes, 25 uM) can lead to proline hydroxylation and ubiquitination, although not degradation, of Ser5-phosphorylated Rpb1 [Citation16]. However, the functional significance of this modification is not known. In any case, our studies showing that transient modulation of CTD phosphorylation status itself also occurs following H2O2 exposure adds another layer to the possible transcriptional responses to oxidative stress.
It is interesting and significant that we detected H2O2-induced ablation of Ser2-P with the 3E10 antibody but saw no effect at all with H5. A number of previous studies have described differences in the behavior of these antibodies. As mentioned above, Chapman et al. provided evidence [Citation66], using a series of differentially phosphorylated heptad repeat peptides that 3E10 preferentially recognizes repeats solely phosphorylated on Ser2, while H5 prefers Ser2/Ser5 diphosphorylated repeats. An earlier study by Jones et al. had also provided evidence that H5 prefers the diphosphorylated derivative [Citation67]. A number of previous studies, both in yeast [Citation30,Citation78] and humans [Citation72,Citation79], showed that Ser5-P levels are highest near promoters and decrease toward gene 3’ ends, while Ser2-P increases until the 3’ end and only then decreases. Consistent with this, Bataille et al. observed from ChIP experiments in yeast that H5 signals increase toward the middle of genes but then decrease by the gene 3’ end [Citation80], while 3E10 signals increase until the 3’ end and only decrease after this. Our data showed the same pattern with 3E10 in untreated HeLa cells, and it was these 3’ signals that were sharply reduced by H2O2.
What underlies the dramatic H2O2-induced loss of Ser2-P that we observed? It is intriguing that what we believe to be highly specific dephosphorylation appears to be independent of the well-studied Ser-2 phosphatase FCP1, even though our data implicates the FCP1 regulator CK2 in the process. Interestingly, diverse CK2 substrates including other phosphatases, such as CDC25B, have been identified [Citation81,Citation82]. Although we are unaware of evidence linking such phosphatases to CTD dephosphorylation, it is not inconceivable that one of these enzymes could be involved in H2O2-mediated Ser2-P loss. Our data also show that PP2A, recently found to be capable of dephosphorylating Ser2-P, as well as Ser5/7-P, is also not involved in the H2O2 response. It is notable that Zheng et al. found a PP2A/Integrator-containing complex capable of dephosphoryating Ser2-P while Huang et al. did not [Citation64,Citation65]. This may reflect the fact that the latter study used 3E10 to detect Ser2-P, while the former did not and is consistent with the observation that the PP2A complex appears to function at promoter-proximal sites. These results are thus consistent with our findings that H2O2-induced Ser2-P dephosphorylation was only detected with 3E10 and occurs in promoter-distal regions.
In this study, we focus on H2O2-induced Ser2-P loss because of its rapidity and completeness. However, we also observed a reduction in phosphorylation of another CTD residue, Tyr1. Although the decrease in total Tyr1-P levels following H2O2 exposure was modest, Tyr1-P signals were more sharply reduced at the gene 3ʹ end in our ChIP experiments. This observation is interesting because a recent study has shown that the level of Tyr1-P affects the phosphorylation of Ser2 by altering the specificity of P-TEFb during transcription [Citation74]. This relationship between Tyr1-P and Ser2-P may be relevant to the molecular mechanism underlying the H2O2-induced Ser2-P that we observe here. Also, with respect to the Tyr1-P function, it is known that Tyr1-P is important for the stability of the CTD. Genetic modification and biochemical experiments showed that loss of Tyr1-P leads to degradation of the CTD by the proteasome [Citation72,Citation73]. Although we have not obtained evidence that H2O2-induced loss of Tyr1-P leads to CTD/Pol II degradation, an interesting possibility is that the Tyr1-P loss we observed functions in quality control by eliminating Pol lI damaged by oxidative stress.
Another interesting aspect of our findings is the effect of oxidative stress on snRNA 3ʹ processing. The similar response of U1, U4, and U5 snRNA processing to the H2O2 treatment suggests a common 3’ end formation mechanism that is possibly dependent on Ser2-P. Our finding that U2 behaved differently than the other three snRNAs was unexpected because all four snRNA genes have similar well-conserved characteristics, such as simple promoters comprising distal and proximal sequence elements, and 3’ box sequences required for proper 3’ processing [Citation83]. In addition, the factors required for snRNA 3’ processing also have appeared to be very similar if not identical. For example, the multi-subunit Integrator complex and Ser2-P are necessary for snRNA 3’ end processing, at least for U1 and U2 [Citation23,Citation28,Citation29,Citation84,Citation85]. Ser7-P, while perhaps not essential [Citation23], is also important for snRNA processing [Citation33,Citation34].
Based on the above, it is difficult to see how U2 and the other snRNAs differ so significantly in their response to H2O2. Other studies though have suggested possible differences between U1 and U2 transcription and 3’ processing. For instance, the two cis-acting elements, the 3ʹ box, and the upstream sequence element (USE), which is a sequence upstream of the 3ʹ box, act together to allow U2 3’ end formation, whereas U1 3’ end formation appears to depend only on the 3’ box [Citation85,Citation86]. Furthermore, it has been reported that transcription of snRNA genes involves distinct TFIID complexes, consisting of only a subset of the TBP-associated factors (TAFs) that constitute the canonical TFIID [Citation87]. U1 and U2 in fact appear to utilize different TAF complexes. TAF7, which has been shown to have a repressive effect on transcription of certain genes [Citation88,Citation89], is present in the U1-associated TAF complex but is absent from the U2 TAF complex [Citation87,Citation90]. However, whether these differences relate to the differential sensitivity between U2 and other snRNA genes to H2O2 are unclear.
In summary, our study has described a striking change in Pol II CTD phosphorylation in response to H2O2. Especially notable was our finding suggesting that the Ser2-P loss is limited to heptad repeats in which Ser2 but not both Ser2 and Ser5 are phosphorylated. This is consistent with a model in which elongating Pol II molecules situated toward gene 3ʹ ends are efficiently and rapidly dephosphorylated after H2O2 exposure, leading to premature termination and/or 3ʹ processing defects (). While many studies have shown that specific transcription factors, and thus specific transcriptional pathways [Citation8,Citation91,Citation92], can be affected by oxidative stress, our study provides a more global mechanism by which cells can bring a rapid but transient halt to gene expression in response to stress. Although further work will be necessary to reveal the precise effects and mechanisms of Ser2-P dephosphorylation induced by H2O2, our study has provided a novel pathway for transcriptional regulation in response to oxidative stress.
Materials and methods
Cell culture and treatments
HeLa, MCF7, and U87 cells were cultured in DMEM supplemented with 10% FBS at 37°C with 5% CO2. DT40 cells were cultured in RPMI1640 medium containing 10% FBS and 1% chicken serum at 37°C with 5% CO2. For heat shock, cells were cultured at 42°C with 5% CO2 for the times indicated in . For UV irradiation, 90% of culture medium was removed before irradiation, leaving 1 to 2 ml of the medium. Cells were then exposed to 40 J/cm2 of 254 nm UV using a Stratalinker 1800 (Stratagene). After irradiation, the collected culture medium was put back into the cell dishes, and cells were incubated at 37°C with 5% CO2 for the time indicated in . H2O2 solution (Sigma) was directly added to the culture medium and gently mixed using an orbital shaker for 30 seconds, then incubated for different times as indicated. Flavopiridol (MedChemExpress), 5,6-dichloro-1-β-d-ribofuranosylbenzimidazole (Sigma), TBB (MedChemExpress), CX-4945 (Cayman chemicals), Actinomycin D (ActD) (Sigma), JQ-1(+) (Sigma) and okadaic acid (Sigma) were dissolved in DMSO then added to cultures at the indicated concentrations in each experiment. Small interfering RNAs were transfected using Lipofectamine RNAiMAX Transfection Reagent (Invitrogen) according to the manufacturers’ instructions, and cells were incubated for 36 hours followed by H2O2 addition as indicated. Sequence of FCP1 and control siRNAs are indicated in Supplemental Table 1.
RT-qPCR
Total RNA was extracted from tissue culture samples using TRIzol (Invitrogen) according to the manufacturer instructions. Total RNA (1 ug) was reverse-transcribed using Maxima RT (Thermo Scientific) primed with random hexamers (Invitrogen). RT-qPCR was performed with Power SYBR using StepOnePlus (Applied Biosystems). Quantitative-PCR data were analyzed by ΔΔCT method, normalizing to 7SK transcripts. Primer sequences are listed in Supplemental Table 1. Experiments were repeated a minimum of three times, as indicated in Figure Legends.
Western blotting
Cells were lysed with SDS sample buffer (50 mM Tris pH 7.4, 100 mM NaCl, 1% Triton X-100, 0.1% SDS, 1% sodium deoxycholate, and 1x proteinase inhibitor cocktail [Biotools]) and resolved by SDS-PAGE (7.5% for Rpb1/phosphorylated RPB1, FCP1, and ACTB; 12% for γ-H2AX). Proteins were then transferred to nitrocellulose membranes and incubated with primary antibodies overnight at 4°C. Primary antibodies used in this study were as follows: Rpb1 (N20: Santa Cruz Biotechnology), Tyr1-P (3D12; Active Motif), Ser2-P (3E10; Millipore), Ser2-P (H5; Covance), Thr4-P CTD heptad (Novus Biologicals; [Citation93]), Ser-5 (3E8; Millipore), Ser7-P (4E12; Millipore), CTDP1/FCP1 (Bethyl Laboratories), γ-H2AX (Bethyl Laboratories), CSTF77 (Bethyl Laboratories), or ACTB (Sigma). Secondary HRP-conjugated anti-mouse and anti-rabbit IgGs (Sigma) were used at 1:10,000 for 2 hours at room temperature. Following washes with PBST (1× PBS, 0.1% Tween-20), ECL-Prime (GE Healthcare) was used to visualize the chemi-luminescence signal.
Chromatin immunoprecipitation assay [ChIP)
The detailed procedures and buffer composition for ChIP were described in Hsin et al[93]. Briefly, HeLa cells were grown to 80% confluence in 10 cm dishes. Cultured cells were cross-linked with 1% formaldehyde for 10 minutes and then collected using a cell scraper. Samples were sonicated until chromatin fragments were shared to a range of 100–400 bp. For immunoprecipitation (IP], 1–4 ug antibody and 20 ul of Sepharose G (GE Healthcare) were added to samples which were then incubated at 4°C overnight. After IP, beads were washed with Wash Buffer (WB) 1, WB2, WB3, and TE-plus, 5 minutes for each wash. DNA fragments were eluted with elution buffer, then eluted DNA fragments were collected by using PCR purification kit (QIAGEN). Purified DNAs were analyzed by qPCR (ABI StepOnePlus). All primer sequences used are listed in Supplemental Table 1. Antibodies used for ChIP were Rpb1 (N20: Santa Cruz Biotechnology), Tyr1-P (3D12; Active Motif), Ser2-P (3E10; Millipore), CSTF77 (Bethyl Laboratories).
Supplemental Material
Download Zip (1.2 MB)Acknowledgments
We thank Jing-Ping Hsin for providing experimental materials and for helpful discussion. We also thank members of the Manley laboratory for discussion. This work was supported by NIH grant R35 GM118136 (J.L.M.). L.L. was supported by the National Science Foundation Graduate Research Fellowship under Grant No. DGE – 1644869.
Disclosure statement
No potential conflict of interest was reported by the authors.
Supplementary material
Supplemental data for this article can be accessed here
Additional information
Funding
References
- Eick D, Geyer M. The RNA polymerase II carboxy-terminal domain (CTD) code. Chem Rev. 2013;113(11):8456–8490.
- Harlen KM, Churchman LS. The code and beyond: transcription regulation by the RNA polymerase II carboxy-terminal domain. Nat Rev Mol Cell Biol. 2017;18(4):263–273.
- Hsin J-P, Manley JL. The RNA polymerase II CTD coordinates transcription and RNA processing. Genes Dev. 2012;26(19):2119–2137.
- Stump AD, Ostrozhynska K. Selective constraint and the evolution of the RNA Polymerase II C-Terminal Domain. Transcription. 2013;4(2):77–86.
- Yurko NM, Manley JL. The RNA polymerase II CTD “orphan” residues: emerging insights into the functions of Tyr-1, Thr-4, and Ser-7. Transcription. 2018;9(1):30–40.
- Zaborowska J, Egloff S, Murphy S. The pol II CTD: new twists in the tail. Nat Struct Mol Biol. 2016;23(9):771–777.
- Gomez-Pastor R, Burchfiel ET, Thiele DJ. Regulation of heat shock transcription factors and their roles in physiology and disease. Nat Rev Mol Cell Biol. 2018;19(1):4–19.
- Priya Dharshini LC, Vishnupriya S, Sakthivel KM, et al., Oxidative stress responsive transcription factors in cellular signalling transduction mechanisms. Cell Signall. 2020;72:109670.
- Samarakkody AS, Shin N-Y, Cantor AB. Role of RUNX Family Transcription Factors in DNA Damage Response. Mol Cells. 2020;43(2):99–106.
- Ganner A, Pfeiffer Z-C, Wingendorf L, et al. The acetyltransferase p300 regulates NRF2 stability and localization. Biochem Biophys Res Comm. 2020;524(4):895–902.
- Katoh Y, Itoh K, Yoshida E, et al. Two domains of Nrf2 cooperatively bind CBP, a CREB binding protein, and synergistically activate transcription. Genes Cells. 2001;6(10):857–868.
- Hentze N, Le Breton L, Wiesner J, et al. Molecular mechanism of thermosensory function of human heat shock transcription factor Hsf1. eLife. 2016;5:e11576.
- Xu D, Zalmas LP, La Thangue NB. A transcription cofactor required for the heat-shock response. EMBO reports. 2008;9(7):662–669.
- Bregman DB, Halaban R, van Gool AJ, et al. UV-induced ubiquitination of RNA polymerase II: a novel modification deficient in Cockayne syndrome cells. Proc Natl Acad Sci USA. 1996;93(21):11586–11590.
- Inukai N, Yamaguchi Y, Kuraoka I, et al. A novel hydrogen peroxide-induced phosphorylation and ubiquitination pathway leading to RNA polymerase II proteolysis. J Biol Chem. 2004;279(9):8190–8195.
- Mikhaylova O, Ignacak ML, Barankiewicz TJ, et al. The von Hippel-Lindau tumor suppressor protein and Egl-9-Type proline hydroxylases regulate the large subunit of RNA polymerase II in response to oxidative stress. Mol Cell Biol. 2008;28(8):2701–2717.
- Ratner JN, Balasubramanian B, Corden J, et al. Ultraviolet radiation-induced ubiquitination and proteasomal degradation of the large subunit of RNA polymerase II. Implications for transcription-coupled DNA repair. Journal of Biological Chemistry. 1998;273(9):5184–5189.
- Yurko N, Liu X, Yamazaki T, et al. MPK1/SLT2 Links Multiple Stress Responses with Gene Expression in Budding Yeast by Phosphorylating Tyr1 of the RNAP II CTD. Mol Cell. 2017;68(5):913–925.
- Bowman EA, Kelly WG. RNA polymerase II transcription elongation and Pol II CTD Ser2 phosphorylation: a tail of two kinases. Nucleus. 2014;5(3):224–236.
- Chen FX, Woodfin AR, Gardini A, et al. PAF1, a Molecular Regulator of Promoter-Proximal Pausing by RNA Polymerase II. Cell. 2015;162(5):1003–1015.
- Ni Z, Saunders A, Fuda NJ, et al. P-TEFb Is Critical for the Maturation of RNA Polymerase II into Productive Elongation In Vivo. Mol Cell Biol. 2008;28(3):1161–1170.
- Gu B, Eick D, Bensaude O. CTD serine-2 plays a critical role in splicing and termination factor recruitment to RNA polymerase II in vivo. Nucleic Acids Res. 2013;41(3):1591–1603.
- Hsin J-P, Xiang K, Manley JL. Function and Control of RNA Polymerase II C-Terminal Domain Phosphorylation in Vertebrate Transcription and RNA Processing. Mol Cell Biol. 2014;34(13):2488–2498.
- Ahn SH, Kim M, Buratowski S. Phosphorylation of Serine 2 within the RNA Polymerase II C-Terminal Domain Couples Transcription and 3′ End Processing. Mol Cell. 2004;13(1):67–76.
- Davidson L, Muniz L, West S. 3′ end formation of pre-mRNA and phosphorylation of Ser2 on the RNA polymerase II CTD are reciprocally coupled in human cells. Genes Dev. 2014;28(4):342–356.
- Li B, Howe L, Anderson S, et al. The Set2 histone methyltransferase functions through the phosphorylated carboxyl-terminal domain of RNA polymerase II. J Biol Chem. 2003;278(11):8897–8903.
- Li M, Phatnani HP, Guan Z, et al. Solution structure of the Set2–Rpb1 interacting domain of human Set2 and its interaction with the hyperphosphorylated C-terminal domain of Rpb1. Proc Natl Acad Sci USA. 2005;102(49):17636–17641.
- Medlin JE, Uguen P, Taylor A, et al. The C-terminal domain of pol II and a DRB-sensitive kinase are required for 3ʹ processing of U2 snRNA. EMBO J. 2003;22(4):925–934.
- Medlin J, Scurry A, Taylor A, et al. P-TEFb is not an essential elongation factor for the intronless human U2 snRNA and histone H2b genes. EMBO J. 2005;24(23):4154–4165.
- Mayer A, Heidemann M, Lidschreiber M, et al. CTD Tyrosine Phosphorylation Impairs Termination Factor Recruitment to RNA Polymerase II. Science. 2012;336(6089):1723–1725.
- David CJ, Boyne AR, Millhouse SR, et al. The RNA polymerase II C-terminal domain promotes splicing activation through recruitment of a U2AF65–Prp19 complex. Genes Dev. 2011;25(9):972–983.
- Nojima T, Rebelo K, Gomes T, et al. RNA Polymerase II Phosphorylated on CTD Serine 5 Interacts with the Spliceosome during Co-transcriptional Splicing. Mol Cell. 2018;72(2):369–379.
- Egloff S, O’Reilly D, Chapman RD, et al. Serine-7 of the RNA polymerase II CTD is specifically required for snRNA gene expression. Science. 2007;318(5857):1777–1779.
- Egloff S, Szczepaniak SA, Dienstbier M, et al. The integrator complex recognizes a new double mark on the RNA polymerase II carboxyl-terminal domain. J Biol Chem. 2010;285(27):20564–20569.
- Jonkers I, Kwak H, Lis JT. Genome-wide dynamics of Pol II elongation and its interplay with promoter proximal pausing, chromatin, and exons. eLife. 2014;3:e02407.
- Baranello L, Wojtowicz D, Cui K, et al. RNA Polymerase II Regulates Topoisomerase 1 Activity to Favor Efficient Transcription. Cell. 2016;165(2):357–371.
- Devaiah BN, Lewis BA, Cherman N, et al. BRD4 is an atypical kinase that phosphorylates Serine2 of the RNA Polymerase II carboxy-terminal domain. Proc Natl Acad Sci USA. 2012;109(18):6927–6932.
- Liu W, Ma Q, Wong K, et al. Brd4 and JMJD6-associated anti-pause enhancers in regulation of transcriptional pause release. Cell. 2013;155(7):1581–1595.
- Gajdušková P, Ruiz de Los Mozos I, Rájecký M, et al. CDK11 is required for transcription of replication-dependent histone genes. Nat Struct Mol Biol. 2020;27(5):500–510.
- Pak V, Eifler TT, Jäger S, et al. CDK11 in TREX/THOC Regulates HIV mRNA 3′ End Processing. Cell Host Microbe. 2015;18(5):560–570.
- Bartkowiak B, Liu P, Phatnani HP, et al. CDK12 is a transcription elongation-associated CTD kinase, the metazoan ortholog of yeast Ctk1. Genes Dev. 2010;24(20):2303–2316.
- Blazek D, Kohoutek J, Bartholomeeusen K, et al. The Cyclin K/Cdk12 complex maintains genomic stability via regulation of expression of DNA damage response genes. Genes Dev. 2011;25(20):2158–2172.
- Fan Z, Devlin JR, Hogg SJ, et al. CDK13 cooperates with CDK12 to control global RNA polymerase II processivity. Sci Adv. 2020;6(18):eaaz5041.
- Greifenberg AK, Hönig D, Pilarova K, et al. Structural and Functional Analysis of the Cdk13/Cyclin K Complex. Cell Rep. 2016;14(2):320–331.
- Liang K, Gao X, Gilmore JM, et al. Characterization of human cyclin-dependent kinase 12 (CDK12) and CDK13 complexes in C-terminal domain phosphorylation, gene transcription, and RNA processing. Mol Cell Biol. 2015;35(6):928–938.
- Tellier M, Zaborowska J, Caizzi L, et al. CDK12 globally stimulates RNA polymerase II transcription elongation and carboxyl-terminal domain phosphorylation. Nucleic Acids Res. 2020;48(14):7712–7727.
- Di Vona C, Bezdan D, Islam ABMMK, et al. Chromatin-wide Profiling of DYRK1A Reveals a Role as a Gene-Specific RNA Polymerase II CTD Kinase. Mol Cell. 2015;57(3):506–520.
- Archambault J, Chambers RS, Kobor MS, et al. An essential component of a C-terminal domain phosphatase that interacts with transcription factor IIF in Saccharomyces cerevisiae. Proc Natl Acad Sci USA. 1997;94(26):14300–14305.
- Chambers RS, Kane CM. Purification and Characterization of an RNA Polymerase II Phosphatase from Yeast. J Biol Chem. 1996;271(40):24498–24504.
- Ghosh A, Shuman S, Lima CD. The structure of Fcp1, an essential RNA polymerase II CTD phosphatase. Mol Cell. 2008;32(4):478–490.
- Mayfield JE, Burkholder NT, Zhang YJ. Dephosphorylating Eukaryotic RNA Polymerase II. Biochim Biophys Acta. 2016;1864(4):372–387.
- Chambers RS, Wang BQ, Burton ZF, et al. The activity of COOH-terminal domain phosphatase is regulated by a docking site on RNA polymerase II and by the general transcription factors IIF and IIB. J Biol Chem. 1995;270(25):14962–14969.
- Kimura M, Suzuki H, Ishihama A. Formation of a Carboxy-Terminal Domain Phosphatase (Fcp1)/TFIIF/RNA Polymerase II (pol II) Complex in Schizosaccharomyces pombe Involves Direct Interaction between Fcp1 and the Rpb4 Subunit of pol II. Mol Cell Biol. 2002;22(5):1577–1588.
- Abbott KL, Renfrow MB, Chalmers MJ, et al. Enhanced Binding of RNAP II CTD Phosphatase FCP1 to RAP74 Following CK2 Phosphorylation. Biochemistry. 2005;44(8):2732–2745.
- Palancade B, Dubois M-F, Bensaude O. FCP1 phosphorylation by casein kinase 2 enhances binding to TFIIF and RNA polymerase II carboxyl-terminal domain phosphatase activity. J Biol Chem. 2002;277(39):36061–36067.
- Yeo M, Lee S-K, Lee B, et al. Small CTD phosphatases function in silencing neuronal gene expression. Science. 2005;307(5709):596–600.
- Yeo M, Lin PS, Dahmus ME, et al. A novel RNA polymerase II C-terminal domain phosphatase that preferentially dephosphorylates serine 5. J Biol Chem. 2003;278(28):26078–26085.
- Qian H, Ji C, Zhao S, et al. Expression and characterization of HSPC129, a RNA polymerase II C-terminal domain phosphatase. Mol Cell Biochem. 2007;303(1–2):183–188.
- Zheng H, Ji C, Gu S, et al. Cloning and characterization of a novel RNA polymerase II C-terminal domain phosphatase. Biochem Biophys Res Commun. 2005;331(4):1401–1407.
- Egloff S, Zaborowska J, Laitem C, et al. Ser7 Phosphorylation of the CTD Recruits the RPAP2 Ser5 Phosphatase to snRNA Genes. Mol Cell. 2012;45(1):111–122.
- Krishnamurthy S, He X, Reyes-Reyes M, et al. Ssu72 Is an RNA polymerase II CTD phosphatase. Mol Cell. 2004;14(3):387–394.
- Meinhart A, Silberzahn T, Cramer P. The mRNA Transcription/Processing Factor Ssu72 Is a Potential Tyrosine Phosphatase. J Biol Chem. 2003;278(18):15917–15921.
- Xiang K, Manley JL, Tong L. An unexpected binding mode for a Pol II CTD peptide phosphorylated at Ser7 in the active site of the CTD phosphatase Ssu72. Genes Dev. 2012;26(20):2265–2270.
- Huang K-L, Jee D, Stein CB, et al. Integrator Recruits Protein Phosphatase 2A to Prevent Pause Release and Facilitate Transcription Termination. Mol Cell. 2020;80(2):345–358.
- Zheng H, Qi Y, Hu S, et al. Identification of Integrator-PP2A complex (INTAC), an RNA polymerase II phosphatase. Science. 2020;370(6520):eabb5872.
- Chapman RD, Heidemann M, Albert TK, et al. Transcribing RNA polymerase II is phosphorylated at CTD residue serine-7. Science. 2007;318(5857):1780–1782.
- Jones JC, Phatnani HP, Haystead TA, et al. C-terminal Repeat Domain Kinase I Phosphorylates Ser2 and Ser5 of RNA Polymerase II C-terminal Domain Repeats. J Biol Chem. 2004;279(24):24957–24964.
- Chao S-H, Price DH. Flavopiridol Inactivates P-TEFb and Blocks Most RNA Polymerase II Transcription in Vivo. J Biol Chem. 2001;276(34):31793–31799.
- Peterlin BM, Price DH. Controlling the Elongation Phase of Transcription with P-TEFb. Mol Cell. 2006;23(3):297–305.
- Albert TK, Rigault C, Eickhoff J, et al. Characterization of molecular and cellular functions of the cyclin-dependent kinase CDK9 using a novel specific inhibitor. Br J Pharmacol. 2014;171(1):55–68.
- Rao GN, Lasségue B, Griendling KK, et al. Hydrogen peroxide-induced c-fos expression is mediated by arachidonic acid release: role of protein kinase C. Nucleic Acids Res. 1993;21(5):1259–1263.
- Descostes N, Heidemann M, Spinelli L, et al. Tyrosine phosphorylation of RNA polymerase II CTD is associated with antisense promoter transcription and active enhancers in mammalian cells. eLife. 2014;3:e02105.
- Hsin J-P, Li W, Hoque M, et al. RNAP II CTD tyrosine 1 performs diverse functions in vertebrate cells. eLife. 2014;3:e02112.
- Mayfield JE, Irani S, Escobar EE, et al. Tyr1 phosphorylation promotes phosphorylation of Ser2 on the C-terminal domain of eukaryotic RNA polymerase II by P-TEFb. eLife. 2019;8:e48725.
- Yamamoto J, Hagiwara Y, Chiba K, et al. DSIF and NELF interact with Integrator to specify the correct post-transcriptional fate of snRNA genes. Nature Commun. 2014;5(1):4263.
- Kim K-J, Cho K-D, Jang KY, et al. Platelet-activating factor enhances tumour metastasis via the reactive oxygen species-dependent protein kinase casein kinase 2-mediated nuclear factor- κ B activation. Immunology. 2014;143(1):21–32.
- Hintermair C, Heidemann M, Koch F, et al. Threonine-4 of mammalian RNA polymerase II CTD is targeted by Polo-like kinase 3 and required for transcriptional elongation. EMBO J. 2012;31(12):2784–2797.
- Komarnitsky P, Cho E-J, Buratowski S. Different phosphorylated forms of RNA polymerase II and associated mRNA processing factors during transcription. Genes Dev. 2000;14(19):2452–2460.
- Dias JD, Rito T, Torlai Triglia E, et al. Methylation of RNA polymerase II non-consensus Lysine residues marks early transcription in mammalian cells. eLife. 2015;4:e11215.
- Bataille AR, Jeronimo C, Jacques P-É, et al. A Universal RNA Polymerase II CTD Cycle Is Orchestrated by Complex Interplays between Kinase, Phosphatase, and Isomerase Enzymes along Genes. Mol Cell. 2012;45(2):158–170.
- Bian Y, Ye M, Wang C, et al. Global Screening of CK2 Kinase Substrates by an Integrated Phosphoproteomics Workflow. Sci Rep. 2013;3(1):3460.
- Theis-Febvre N, Filhol O, Froment C, et al. Protein kinase CK2 regulates CDC25B phosphatase activity. Oncogene. 2003;22(2):220–232.
- Jawdekar GW, Henry RW. Transcriptional regulation of human small nuclear RNA genes. Biochim Biophys Acta. 2008;1779(5):295–305.
- Baillat D, Hakimi M-A, Näär AM, et al. Integrator, a Multiprotein Mediator of Small Nuclear RNA Processing, Associates with the C-Terminal Repeat of RNA Polymerase II. Cell. 2005;123(2):265–276.
- Uguen P, Murphy S. The 3’ ends of human pre-snRNAs are produced by RNA polymerase II CTD-dependent RNA processing. EMBO J. 2003;22(17):4544–4554.
- Uguen P, Murphy S. 3’-box-dependent processing of human pre-U1 snRNA requires a combination of RNA and protein co-factors. Nucleic Acids Res. 2004;32(10):2987–2994.
- Zaborowska J, Taylor A, Roeder RG, et al. A novel TBP-TAF complex on RNA Polymerase II-transcribed snRNA genes. Transcription. 2012;3(2):92–104.
- Devaiah BN, Lu H, Gegonne A, et al. Novel functions for TAF7, a regulator of TAF1-independent transcription. J Biol Chem. 2010;285(50):38772–38780.
- Kloet SL, Whiting JL, Gafken P, et al. Phosphorylation-Dependent Regulation of Cyclin D1 and Cyclin A Gene Transcription by TFIID Subunits TAF1 and TAF7. Mol Cell Biol. 2012;32(16):3358–3369.
- Takahashi H, Takigawa I, Watanabe M, et al. MED26 regulates the transcription of snRNA genes through the recruitment of little elongation complex. Nat Commun. 2015;6(1):5941.
- Hayes JD, Dinkova-Kostova AT, Tew KD. Oxidative Stress in Cancer. Cancer Cell. 2020;38(2):167–197.
- Tonelli C, Chio IIC, Tuveson DA. Transcriptional Regulation by Nrf2. Antioxid Redox Signal. 2018;29(17):1727–1745.
- Hsin J-P, Sheth A, Manley JL. RNAP II CTD Phosphorylated on Threonine-4 Is Required for Histone mRNA 3’ End Processing. Science. 2011;334(6056):683–686.