Abstract
In this study, 24 Antarctic bacteria, isolated from sediment and soil samples from Deception and Galindez Islands, were characterized for their antimicrobial activity and response to 13 antibiotics and seven heavy metals. Multiple antibiotic resistance was observed for 67% of sediment isolates and 92% of soil isolates, suggesting medium anthropogenic impact in these Antarctic regions. The results revealed a varying response of the Antarctic bacteria to the tested heavy metals. All isolates showed multiple metal resistance towards two to six heavy metals, with minimum inhibitory concentrations ranging from 1.0 mM to 23.2 mM. The majority of the strains in both groups were resistant to lead, nickel, copper and zinc. Plasmids were detected in 21% of isolates. A consortium of highly metal-resistant bacteria could be developed with potential application for biological treatment of wastewaters. Strains that are highly sensitive to cadmium would be promising for developing biosensors to detect this highly toxic heavy metal in environmental samples. All Antarctic bacteria were found to inhibit the growth of one to all eight of the tested indicator bacteria, and 87% inhibited the growth of one to all four of the yeast indicator cultures. Promising psychrotolerant strains were detected as a valuable source of new antimicrobial compounds.
Introduction
Although strict guidelines are provided in the Protocol of Environmental Protection to the Antarctic Treaty for protection of the Antarctic environment, a moderate level of anthropogenic contamination has been reported in Antarctica due to global warming, population growth and industrial development in countries of the Southern Hemisphere (Bargagli Citation2008; Corsolini Citation2009; Lo Giudice et al. Citation2013). Toxic compounds such as heavy metals, antibiotics, pesticides and other persistent pollutants can be transferred to the Antarctic continent through natural processes by mass flow in the atmosphere and water, improper disposal practices and/or incineration of wastes produced at research stations (De Souza et al. Citation2006; Corsolini Citation2009; Lo Giudice et al. Citation2013). In the Antarctic environment, the reduced growth rates of organisms due to the low temperature promote high concentrations of potential contaminants in the Antarctic biota (Grotti et al. Citation2008; Mangano et al. Citation2014). Heavy metals at high levels can have negative effects on all forms of life, while antimicrobials inhibit microbial activity, thus altering the microbiosphere and the biogeochemical cycles of biogenic elements (Martinez Citation2009). Bacteria are generally the first organisms to be affected by the growing presence of toxic compounds in the environment, including antibiotics and heavy metals from natural and industrial processes, resulting in increased numbers of antibiotic- and metal-resistant bacteria in the environment (Silver Citation1996; Davis & Webb Citation1998; Bruins et al. Citation2000; Nies Citation2003).
The increase in resistant strains to commonly used antibiotics in clinical practice necessitates searching for new compounds with antibacterial activity. The adaptations developed by Antarctic microorganisms in the extreme environment promote them as a potential new source of active compounds for the control of pathogenic microorganisms (O'Brien et al. Citation2004; Sánchez et al. Citation2009). Little information is available pertaining to antimicrobial compounds of bacteria from some geographical locations in Antarctica, such as the South Shetland Islands and Argentine Islands. Previously, the authors isolated 24 heterotrophic bacteria from sediment and soil samples collected in the Deception and Galindez Islands in Antarctica; the recovered strains were characterized for their phylogenetic affiliation, psychrotolerance, halotolerance and production of hydrolytic enzymes (Tomova et al. Citation2014). The present work was conducted to estimate the response of the isolated Antarctic bacteria towards antibiotics and heavy metals, as well as their potential to produce antimicrobial substances. Monitoring antibiotic resistance in bacteria from remote areas such as Antarctica could be a useful tool to evaluate the degree of anthropogenic impact. Highly metal-resistant psychrotolerant strains can be used to develop tools to assess the metal levels in cold environments and could be also a valuable resource for bioremediation applications. Promising psychrotolerant strains could be detected as a valuable source of new antimicrobial compounds.
Materials and methods
Study area and microorganisms
The 24 bacterial strains used in the present study were isolated previously in the Institute of Microbiology (Sofia, Bulgaria) from samples collected on two Antarctic islands. Twelve strains, designated A1, were isolated from a brook sediment sample collected in Whalers Bay on Deception Island, South Shetland Islands (62°59' S, 60°34' W) (), where organic matter and nitrogen contents of 0.2–1.19% and 0.04%, respectively, have been determined (Fermani et al. Citation2007; Tejedo et al. Citation2012). The other 12 strains, designated A2, were isolated from an ornithogenic soil sample taken near the Ukrainian Research Station ‘Akademik Vernadsky' on Galindez Island, Argentine Islands (65°14′44.6″ S, 64°15′26″ W) (), where total carbon and nitrogen contents of 0.22–0.41% and 3.40%, respectively, have been determined (Parnikoza et al. Citation2007; Iutynska & Tashyreva Citation2008). The Antarctic strains belong to Gammaproteobacteria, Betaproteobacteria, Firmicutes and Actinobacteria, based on 16S rRNA gene sequencing (Tomova et al. Citation2014). The pure cultures were stored at +4°C and recultured on nutrient agar slants every 2 months to maintain their purity and viability. Suspensions of pure cultures mixed with 30% (v/v) glycerol were stored at –80°C.
Antibiotic susceptibility and resistance tests
The Antarctic bacteria were checked for antibiotic resistance with the following antibiotics: ciprofloxacin (Cp, 5 µg), gentamicin (G, 10 µg), amikacin (Am, 30 µg), tobramycin (Tb, 10 µg), novobiocin (Nb, 5 µg), lincomycin (L, 15 µg), tetracycline (T, 30 µg), ampicillin (A, 10 µg), chloramphenicol (C, 30 µg), vancomycin (V, 30 mg), erythromycin (E, 15 µg), kanamycin (K, 30 µg) and cefazolin (Cfz, 30 µg).
Antibiotic susceptibility of the strains was assayed following the Kirby–Bauer disc diffusion method (Bauer et al. Citation1966) on peptone–yeast extract agar (PYA) medium. Aliquots of each bacterial suspension grown exponentially in nutrient broth were spread on the surface of PYA plates. Antibiotic discs (Bul Bio; NCIPD, Sofia, Bulgaria) impregnated with known amounts of antibiotics were placed aseptically on the surface of the inoculated plates and incubated at 18 ± 2°C for 24 h. After incubation, the organisms were classified as sensitive or resistant to each antibiotic according to the diameter of inhibition zones (including the disc) given on a standard antibiotic disc chart.
Heavy metal resistance tests
The Antarctic bacteria were screened for their heavy metal resistance patterns using the agar well diffusion method (Hassen et al. Citation1998). Seven heavy metals – chromium (Cr), copper (Cu), nickel (Ni), cobalt (Co), cadmium (Cd), zinc (Zn) and lead (Pb) – were used as salts: K2Cr2O7, CuSO4.5H2O, NiCl2, CoCl2.6H2O, CdSO4.8H2O, ZnSO4.7H2O and Pb(CH3COO)2.3H2O, respectively. In a preliminary test, 0.05% (w/v) metal salt solutions were prepared in distilled water and sterilized in a boiling water bath for 20 min. Sterile PYA plates were prepared and wells (7 mm in diameter) were punched by a sterile borer. After inoculation of the plates with overnight-grown indicator cultures, 100 µl of each metal salt solution was added to the wells. After incubation of the plates at 18–20°C for 48 h, the inhibition (sterile) zones were measured as an indicator of resistance/sensitivity. Zones were recorded as the distance from the edge of the zone to the edge of the well. Isolates showing a clear zone of 1 mm or less were considered as resistant strains (Rani et al. Citation2010).
The minimum inhibitory concentration (MIC) of metal ions for the Antarctic bacteria was determined by gradually increasing or decreasing the heavy metal concentrations in the following cationic concentration ranges (mM): Cu: 0.2–24; Cd: 0.14–14.2; Ni: 3.8–46; Cr: 1.4–17; Zn: 0.2–20.8; Co: 0.2–21; Pb: 1.3–26. The MIC value was defined as the lowest concentration of metal ion at which a visible inhibition (clear) zone of 1–2 mm around the well was observed after incubation of the plates at 18–20°C for 48 h (Sabdono et al. Citation2012). Strains that were not inhibited by a concentration of heavy metals less than 1.0 mM were regarded as resistant (Malik & Jaiswal Citation2000).
Plasmid profiling
Plasmid DNA was extracted from the bacterial cultures grown in nutrient broth using the commercial GenElute Plasmid Midiprep Kit (Sigma-Aldrich) in accordance with the manufacturer's instructions. Agarose gel electrophoresis was performed in a horizontal slab gel of 0.7% agarose submerged in Tris acetate running buffer at 70 V for 2 h. Plasmid DNA bands were stained with ethidium bromide (0.5 µg ml–1) for 15 min and visualized by an ultraviolet transilluminator. Approximate sizes of plasmids were calculated from logarithmic plots against reference plasmids of DNA ladder, supercoiled (Sigma D5292; Sigma-Aldrich, St. Louis, MO (USA)).
Screening for antimicrobial activity
Antimicrobial compound production by the Antarctic bacteria was determined using the agar well diffusion method with slight modification (Perez et al. Citation1990). The following indicator cultures were used: Gram-positive bacteria Bacillus subtilis, Bacillus cereus, Sarcina lutea and Micrococcus luteus; Gram-negative bacteria Pseudomonas aeruginosa, Escherichia coli, Acinetobacter johnsonii and Xanthomonas oryzae; and yeast strains Candida lipolytica, Candida davisiana, Cryptococcus terricola and Leucosporidiella muscorum. The Antarctic bacteria were grown in nutrient broth at 18–20°C for 24 h before detection of the antimicrobial activity by the agar well diffusion method. Suspensions of overnight-grown indicator cultures were prepared and smeared on to the surface of nutrient agar in Petri plates. Wells (7 mm in diameter) were punched aseptically in the agar medium, to which 100 μl of each strain suspension was added. After incubation of the plates at 25°C for 48 h, the diameter (in mm) of the resulting clear zones (including the well), if any, was recorded.
Results and discussion
Antibiotic resistance and sensitivity of the Antarctic bacteria
The resistance patterns to 13 antibiotics in the 24 Antarctic bacteria were determined and the results are shown in . No remarkable difference in the frequency of antibiotic resistance was observed between the A1 and A2 groups of isolates. Of the A1 group, all Proteobacteria (eight strains) showed multiple antibiotic resistance, while all Actinobacteria strains were sensitive to 12 or all 13 of the antibiotics. Of the A2 strains, 92% showed multiple antibiotic resistance, and only Bacillus sp. A2-4 was sensitive to all target antibiotics. Bacteria of both tested groups showed a high degree of multiple antibiotic resistance, most frequently towards lincomycin (79%), cefazolin (75%), ampicillin (71%), novobiocin and erythromycin (62%), chloramphenicol and vancomycin (58%), and tetracycline (42%). Only Pseudomonas sp. A1-7 and Burkholderia sp. A2-16 showed resistance to gentamicin, Sporosarcina sp. A2-3 and Burkholderia sp. A2-16 to ciprofloxacin, Pseudomonas sp. A2-8 and Burkholderia sp. A2-16 to amikacin and kanamycin, and only Burkholderia sp. A2-16 was resistant to tobramycin. Of the tested strains, only Burkholderia sp. A2-16 was found to be resistant to all target antibiotics.
Table 1. Antibiotic susceptibility of the Antarctic bacteria expressed as zone of inhibition (mm diameter).
Bacterial resistance to antibiotics is an extensively investigated phenomenon of considerable medical importance (Goldstein Citation2007). Resistant bacteria are common in the natural environment, especially in aquatic habitats (Kümmerer Citation2009), and even in habitats that seem unlikely to have been exposed to anthropogenic antibiotics (Séveno et al. Citation2002; Moskot et al. Citation2012). It has long been recognized that specific antibiotic resistance mechanisms can be acquired through mutation of the bacterial genome or by gaining additional genes; different physiological states are also important for the survival of bacteria in the presence of antibiotics. Antibiotic resistance genes are often located on plasmids that are able to transfer horizontally among diverse bacterial populations, thus contributing to the widespread dissemination of antibiotic resistance in the environment (Davison Citation1999; Herreros et al. Citation2005). Antibiotic resistance has also been reported among bacteria in cold environments such as Antarctic sandstone in McMurdo Valley (Siebert et al. Citation1996), the Arctic permafrost subsoil in Siberia (Mindlin et al. Citation2008), Antarctic King George Island (Wong et al. Citation2011), and seawater and penguin faecal samples collected near Palmer Station, Antarctica (Miller et al. Citation2009). A high frequency of bacteria resistant to antibiotics can be viewed as an indicator of environmental pollution (Zhang et al. Citation2009). Isolation of antibiotic-resistant bacteria from areas with limited human activity can be a good indicator of human impact on these natural areas, such as the Antarctic region (Miller et al. Citation2009). The relatively high percentage of multidrug-resistant bacteria determined in this study (79%) may suggest medium anthropogenic impact in these Antarctic regions.
Heavy metal resistance of the Antarctic bacteria
The Antarctic bacteria were investigated for resistance to seven heavy metals, and MICs for each metal ion were determined. A varying response of bacterial strains of both groups to the tested heavy metals was observed (). Although there is no currently acceptable concentration of metal ions, which could be used for distinguishing metal-resistant and metal-sensitive bacteria, strains able to grow at concentrations of metal ions at and above 1.0 mM were considered resistant, as suggested by Malik and Jaiswal (Citation2000). The highest MIC values of each heavy metal determined for A1 and A2 strains were compared (). For A1 strains (A), MICs decreased in the order: Pb (21.1 mM, Janthinobacterium sp. A1-4), Ni (15.4 mM, Rhodococcus sp. A1-14), Cu (12 mM, Pseudomonas sp. A1-12 and Rhodococcus sp. A1-14), Zn (6.9 mM, Pseudomonas sp. A1-1, A1-7, A1-8; Janthinobacterium sp. A1-4 and Arthrobacter sp. A1-17), Co (4.2 mM, Pseudomonas sp. A1-7), Cr (3.4 mM, Arthrobacter sp. A1-16 and A1-17) and Cd (1.4 mM, Arthrobacter sp. A1-16). The highest MICs for A2 strains (B) decreased in a similar order: Ni (23.6 mM, Pseudomonas sp. A2-1, A2-8), Pb (10.5 mM, Sporosarcina sp. A2-3 and Pseudomonas sp. A2-8, A2-13), Cu (8 mM, Pseudomonas sp. A2-1, A2-7), Zn (6.9 mM, Pseudomonas sp. A2-1, A2-15), Cr (6.8 mM, Bacillus sp. A2-4), Co (2.1 mM, Sporosarcina sp. A2-3, Bacillus sp. A2-4 and Pseudomonas sp. A1-13) and Cd (1.4 mM, Pseudomonas sp. A2-15). The observed similar order of resistance to metals of both groups of strains (according to the highest MICs) probably relates to the metal concentrations in the sampling areas. Lo Giudice et al. (Citation2013) found tolerance to the heavy metals of bacterial isolates from Antarctic shallow sediments (in the Terra Nova Bay) in the order Cd > Cu > Zn > Hg, which appeared to be strictly related to the metal concentrations in the study area. The present results revealed a high frequency of multimetal resistance of both groups of strains: all A1 strains exhibited resistance to four to six metal ions, and 92% of A2 strains were resistant to three to six metal ions (). Although sediments are considered the natural collectors of pollutants (Andrade et al. Citation2001), the frequency of metal resistance of A1 sediment isolates was found to be comparable to that of A2 soil isolates. All A1 strains were found to be resistant to Pb, Cu and Ni ions, and 92% (11 strains) to Zn ions. All A2 strains were resistant to Pb and Ni ions, 58% (seven strains) to Cu ions and 42% (five strains) to Zn ions. Half of A1 strains and 83% of A2 strains showed resistance to Cr ions. The majority of the strains in each group (75%) were found to be sensitive to Co; about 92% of A1 strains and 83% of A2 strains were sensitive to Cd ions.
Figure 3. Minimum inhibitory concentration (MIC) of heavy metals against the Antarctic bacteria. (A) A1 isolates; (B) A2 isolates.
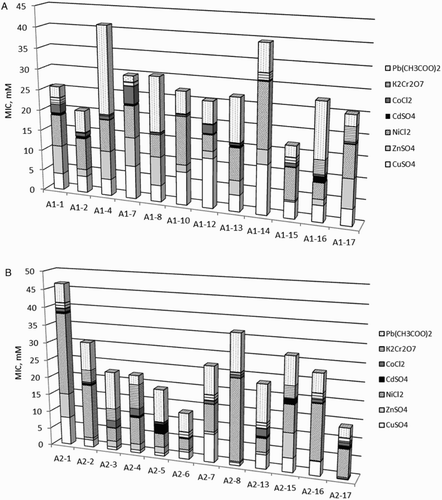
Figure 4. Comparison of multimetal resistance of A1 and A2 groups of the Antarctic bacteria. 1 = resistance to two heavy metals; 2 = resistance to three heavy metals; 3 = resistance to four heavy metals; 4 = resistance to five heavy metals; 5 = resistance to six heavy metals.
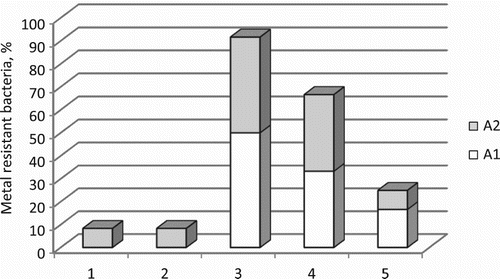
Resistance to heavy metals has been reported for different bacterial genera and in some cases it is present together with resistance to antibiotics. Both kinds of resistance are becoming a global phenomenon, with resistant bacteria that are ubiquitous and have been detected in Antarctic seawater (De Souza et al. Citation2006). As suggested by Shruti et al. (Citation2012), the varying response of the tested bacteria to the heavy metals may be due to the differences in their cell-wall composition or to variations in resistance mechanisms. The tolerance of soil bacteria to heavy metals has been proposed as an indicator of the potential toxicity of heavy metals to other forms of biota (Hassen et al. Citation1998). Different resistance mechanisms that have developed in bacteria could serve as a basis for their use in bioremediation approaches (Filali et al. Citation2000; Malik Citation2004). In this sense, the following multimetal-resistant strains can be selected as especially promising for application in bioremediation of sites polluted by heavy metals: 10 Pseudomonas strains (A1-1, A1-7, A1-8, A1-10, A1-12, A2-1, A2-2, A2-7, A2-13, A2-15), Janthinobacterium sp. A1-4, Rhodococcus sp. A1-14, Arthrobacter sp. (A1-16, A1-17), Sporosarcina sp. A2-3, Bacillus sp. A2-4 and Burkholderia sp. A2-16. The high metal resistance of these strains may suggest that they overproduce some multidrug resistance efflux pumps that are known to be involved in bacterial resistance to a wide range of toxic compounds (Pages et al. Citation2008). A mixed culture model of selected isolates could be developed for biological treatment of wastewaters. Strains that are highly sensitive to Cd and Co could be valuable in developing biosensors to detect these most toxic metal ions in environmental samples.
Plasmid testing
The Antarctic bacteria were tested to determine whether metal and antibiotic resistance genes are plasmid encoded. Plasmid DNA, most probably encoding metal/antibiotic resistance genes, was detected and isolated in five multimetal- and multiantibiotic-resistant Pseudomonas strains, A1-1, A1-2, A1-7, A2-1 and A2-13 (data not shown). Resistance to heavy metals in bacteria is usually associated with plasmids which also encode resistance to antibiotics, although a direct correlation between antibiotic and heavy metal resistance of the strains tested in this study cannot be established. Clustered resistance genes are more likely to simultaneously pass to other bacteria in the environment (Filali et al. Citation2000; Lawrence Citation2000; Spain & Alm Citation2003). Thus, in an environment with multiple stresses, for example antibiotics and heavy metals, it would be more beneficial to the survival of bacteria to acquire resistance to both stresses. It has been reported that bacterial plasmids are ubiquitous, including natural microbial assemblages of the marine ecosystem of Antarctica (Kobori et al. Citation1984; De Souza et al. Citation2006; Miller et al. Citation2009; Mangano et al. Citation2011). Plasmid DNA was detected in 25% of A1 strains and in 17% of A2 strains. No detectable plasmids were established for the other resistance strains, suggesting the location of resistance genes in the chromosomes.
Antimicrobial activity
In the course of screening for novel antimicrobial substances, the Antarctic isolates were tested for antimicrobial activity against eight bacterial and four yeast cultures. The results revealed a higher potential of A1 isolates to produce antimicrobial substances than A2 isolates (). As can be seen, the number of A1 isolates inhibiting the growth of bacteria B. subtilis, B. cereus, A. johnsonii and E. coli, and yeast L. muscorum, was comparable to the number of A2 isolates, while a higher percentage of A1 isolates inhibited the growth of bacteria M. luteus, S. lutea and P. aeruginosa, and yeasts C. lipolytica, C. davisiana and Cr. terricola (75%, 58%, 75%, 50%, 58% and 41%, respectively) than the percentage of A2 isolates inhibiting the growth of these target strains (8%, 25%, 25%, 41%, 41% and 16%, respectively). Of the A1 isolates, Pseudomonas sp. A1-1 showed the broadest spectrum, inhibiting the growth of all target bacterial and yeast cultures, and Arthrobacter sp. A1-15 showed the narrowest spectrum, inhibiting the growth of only one bacterial and two yeast cultures. Pseudomonas sp. A1-8 exhibited activity against all bacterial cultures, followed by Pseudomonas sp. A1-2, A1-10 and A1-13, showing activity against seven of the eight target bacterial strains. Of the A2 isolates, 33% showed inhibition activity against five cultures, 33% against four cultures and 25% against three of the bacterial pathogens. Sporosarcina sp. A2-17 strain showed the narrowest spectrum of activity, against two bacterial and two yeast cultures.
Table 2. Antimicrobial activity of the Antarctic bacteria expressed as zone of inhibition (mm diameter).
Owing to the harsh conditions, microorganisms living on the Antarctic continent and neighbouring islands have acquired unique adaptation strategies to survive in the extreme environment. In order to gain competitive advantage, some microorganisms produce antimicrobial compounds to inhibit the growth of their competitors (O'Brien et al. Citation2004; Lo Giudice et al. Citation2007a). Cold-adapted microorganisms have been recognized as a new and promising source to search for novel antimicrobial metabolites with potential for application in different industrial sectors, such as food-processing, pharmaceutical chemistry and cosmetic industries (Ravot et al. Citation2006; Sánchez et al. Citation2009, Citation2010). The antimicrobial activities of microorganisms from Antarctica, especially bacteria, have only been investigated recently. Antibiotic activities of culturable Antarctic bacteria have been reported for Actinobacteria, Gammaproteobacteria, bacilli and cyanobacteria (Moncheva et al. Citation2002; Nedialkova & Naidenova Citation2004; O'Brien et al. Citation2004; De Souza et al. Citation2006; Lo Giudice et al. Citation2007b; Biondi et al. Citation2008; Shekh et al. Citation2011; Solecka et al. Citation2012; Dong et al. Citation2013; Encheva et al. Citation2013; Asencio et al. Citation2014).
It is known that culture conditions have a major impact on the production of microbial bioactive secondary metabolites such as antibiotics. Competitive cultivation between different microbial taxa has been suggested as a new tool to induce antimicrobial metabolite production (Mearns-Spragg et al. Citation1998; Lo Giudice et al. Citation2007a). In the present study, all of the studied Antarctic bacteria expressed antibacterial activity against two to eight indicator bacteria, and 75% showed antifungal activity against one to four indicator yeasts. Of particular importance are isolates exhibiting good inhibition activity against human pathogenic bacteria, namely E. coli, P. aeruginosa and A. johnsonii. Some Antarctic strains limiting the development of phytopathogenic strain X. oryzae, such as Pseudomonas sp. A1-1, Pseudomonas sp. A1-10, Arthrobacter sp. A1-15, Sporosarcina sp. A2-6 and Burkholderia sp. A2-16, have potential for application in agriculture, for plant protection. The strains combining antimicrobial activity and multiple resistance to antibiotics and heavy metals are especially promising, including seven Pseudomonas strains (A1-1, A1-2, A1-7, A1-8, A1-10, A2-13, A2-15), Janthinobacterium sp. A1-4 and Burkholderia sp. A2-16, suggesting that these Antarctic bacteria are potential sources of genes encoding for antimicrobial compounds, antibiotic resistance and metal resistance. These three capabilities probably provide competitive advantage to Antarctic bacteria to enable them to survive in the harsh environment (Russell Citation2006; Lo Giudice et al. Citation2007a, Citation2007b; Wong et al. Citation2011).
Acknowledgement
The authors thank Professor O. Tashirev for providing sediment and soil samples.
Funding
The authors thank the National Fund for Scientific Research of the Bulgarian Ministry of Education and Science for financial support of this work [grant DNTS/Ukraine 01/1-2012].
Disclosure statement
No potential conflict of interest was reported by the authors.
Notes
1 This article is dedicated to the memory of Dr Victoria Gesheva.
References
- Andrade S, Poblet A, Scagliola M, Vodopivez C, Curtosi A, Pucci A, Marcovecchio J. 2001. Distribution of heavy metals in surface sediments from an Antarctic marine ecosystem. Environ Monit Assess. 66:147–158. doi: 10.1023/A:1026487612504
- Asencio G, Lavin P, Alegría K, Domínguez M, Bello H, González-Rocha G, González-Aravena M. 2014. Antibacterial activity of the Antarctic bacterium Janthinobacterium sp. SMN 33.6 against multi-resistant Gram-negative bacteria. Electron J Biotechnol. 17:1–5. doi: 10.1016/j.ejbt.2013.12.001
- Bargagli R. 2008. Environmental contamination in Antarctic ecosystems. Sci Total Environ. 400:212–226. doi: 10.1016/j.scitotenv.2008.06.062
- Bauer AW, Kirby WMM, Sherris JC, Turck M. 1966. Antibiotic susceptibility testing by a standardized single disc method. Am J Clin Pathol. 45:493–496.
- Biondi N, Tredici MR, Taton A, Wilmotte A, Hodgson DA, Losi D, Marinelli F. 2008. Cyanobacteria from benthic mats of Antarctic lakes as a source of new bioactivities. J Appl Microbiol. 105:105–115. doi: 10.1111/j.1365-2672.2007.03716.x
- Bruins MR, Kapil S, Oehme FW. 2000. Microbial resistance to metals in the environment. Ecotoxicol Environ Safe. 45:198–207. doi: 10.1006/eesa.1999.1860
- Corsolini S. 2009. Industrial contaminants in Antarctic biota. J Chromatogr A. 1216:598–612. doi: 10.1016/j.chroma.2008.08.012
- Davis J, Webb V. 1998. Antibiotic resistance in bacteria. Emerging infections: biomedical research reports. New York: Academic Press, 239–273.
- Davison J. 1999. Genetic exchange between bacteria in the environment. Plasmid. 42:73–91. doi: 10.1006/plas.1999.1421
- De Souza MJ, Nair S, Loka Bharathi PA, Chandramohan D. 2006. Metal and antibiotic-resistance in psychrotrophic bacteria from Antarctic marine waters. Ecotoxicology. 15:379–384. doi: 10.1007/s10646-006-0068-2
- Dong N, Di Z, Yu Y, Yuan M, Zhang X, Li H. 2013. Extracellular enzyme activity and antimicrobial activity of culturable bacteria isolated from soil of Grove Mountains, East Antarctica. Acta Microbiol Sin. 53:1295–1306.
- Encheva M, Zaharieva N, Kenarova A, Chipev N, Chipeva V, Hristova P, Ivanova I, Moncheva P. 2013. Abundance and activity of soil actinomycetes from Livingston Island, Antarctica. Bulg J Agric Sci. 19:68–71.
- Fermani P, Metaloni G, Van de Vijver B. 2007. Soil microalgal communities on an antarctic active volcano (Deception Island, South Shetlands). Polar Biol. 30:1381–1393. doi: 10.1007/s00300-007-0299-6
- Filali BK, Taoufik J, Zeroual Y, Dzairi FZ, Talbi M, Blaghen M. 2000. Waste water bacterial isolates resistant to heavy metals and antibiotics. Curr Microbiol. 41:151–156. doi: 10.1007/s0028400
- Goldstein FW. 2007. Combating resistance in a challenging, changing environment. Clin Microbiol Infect. 13:2–6. doi: 10.1111/j.1469-0691.2007.01721.x
- Grotti M, Soggia F, Lagomarsino C, Dalla Riva S, Goessler W, Francesconi KA. 2008. Natural variability and distribution of trace elements in marine organisms from Antarctic coastal environments. Antarc Sci. 20:39–51.
- Hassen A, Saidi N, Cherif M, Boudabous A. 1998. Resistance of environmental bacteria to heavy metal. Bioresour Technol. 64:7–15. doi: 10.1016/S0960-8524(97)00161-2
- Herreros MA, Sandoval H, González L, Castro JM, Fresno JM, Tornadijo ME. 2005. Antimicrobial activity and antibiotic resistance of lactic acid bacteria isolated from Armada cheese (a Spanish goats’ milk cheese). Food Microbiol. 22:455–459. doi: 10.1016/j.fm.2004.11.007
- Iutynska HO, Tashyreva HO. 2008. Microbial communities of soil-like substrates of Antarctic island Galindez (Article in Ukrainian). Mikrobiol Z. 70:3–8.
- Kobori H, Sullivan CW, Shizuya H. 1984. Bacterial plasmids in Antarctic natural microbial assemblages. Appl Environ Microbiol. 48:515–518.
- Kümmerer K. 2009. Antibiotics in the aquatic environment – a review. Part II. Chemosphere. 75:435–441. doi: 10.1016/j.chemosphere.2008.12.006
- Lawrence JG. 2000. Clustering of antibiotic resistance genes: beyond the selfish operon. ASM News. 66:281–286.
- Lo Giudice A, Brilli M, Bruni V, De Domenico M, Fani R, Michaud L. 2007a. Bacterium–bacterium inhibitory interactions among psychrotrophic bacteria isolated from Antarctic seawater (Terra Nova Bay, Ross Sea). FEMS Microbiol Ecol. 60:383–396. doi: 10.1111/j.1574-6941.2007.00300.x
- Lo Giudice A, Bruni V, Michaud L. 2007b. Characterization of Antarctic psychrotrophic bacteria with antibacterial activities against terrestrial microorganisms. J Basic Microbiol. 47:496–505. doi: 10.1002/jobm.200700227
- Lo Giudice A, Casella P, Bruni V, Michaud L. 2013. Response of bacterial isolates from Antarctic shallow sediments towards heavy metals, antibiotics and polychlorinated biphenyls. Ecotoxicology. 22:240–250. doi: 10.1007/s10646-012-1020-2
- Malik A. 2004. Metal bioremediation through growing cells. Environ Int. 30:261–278. doi: 10.1016/j.envint.2003.08.001
- Malik A, Jaiswal R. 2000. Metal resistance in Pseudomonas strains isolated from soil treated with industrial wastewater. World J Microbiol Biotechnol. 16:177–182. doi: 10.1023/A:1008905902282
- Mangano S, Caruso C, Michaud L, Giudice AL, Bruni V. 2011. Incidence of plasmid and antibiotic resistance in psychrotrophic bacteria isolated from Antarctic sponges. Atti Accad Pelorit Pericol Cl Sci Fis Mat Nat. 89 1–9.
- Mangano S, Michaud L, Caruso C, Giudice AL. 2014. Metal and antibiotic resistance in psychrotrophic bacteria associated with the Antarctic sponge Hemigellius pilosus (Kirkpatrick, 1907). Polar Biol. 37:227–235. doi: 10.1007/s00300-013-1426-1
- Martinez JL. 2009. Environmental pollution by antibiotics and by antibiotic resistance determinants. Environ Pollut. 157:2893–2902. doi: 10.1016/j.envpol.2009.05.051
- Mearns-Spragg A, Bregu M, Boyd KG, Burgess JG. 1998. Cross-species induction and enhancement of antimicrobial activity produced by epibiotic bacteria from marine algae and invertebrates, after exposure to terrestrial bacteria. Lett Appl Microbiol. 27:142–146. doi: 10.1046/j.1472-765X.1998.00416.x
- Miller RV, Gammon K, Day MJ. 2009. Antibiotic resistance among bacteria isolated from seawater and penguin fecal samples collected near Palmer Station, Antarctica This article is one of a selection of papers in the Special Issue on Polar and Alpine Microbiology. Can J Microbiol. 55:37–45. doi: 10.1139/W08-119
- Mindlin S, Soina V, Petrova M, Gorlenko Z. 2008. Isolation of antibiotic resistance bacterial strains from Eastern Siberia permafrost sediments. Russ J Genet. 44:27–34. doi: 10.1134/S1022795408010043
- Moncheva P, Tishkov S, Dimitrova N, Chipeva V, Antonova–Nikolova S, Bogatzevska N. 2002. Characteristics of soil actinomycetes from Antarctica. J Cult Collect. 3:3–14.
- Moskot M, Kotlarska E, Jakóbkiewicz-Banecka J, Gabig-Cimińska M, Fari K, Grzegorz Węgrzyn G, Wróbel B. 2012. Metal and antibiotic resistance of bacteria isolated from the Baltic Sea. Int Microbiol. 15:131–139.
- Nedialkova D, Naidenova M. 2004. Screening the antimicrobial activity of Actinomycetes strains isolated from Antarctica. J Cult Collect. 4:29–35.
- Nies DH. 2003. Efflux-mediated heavy metal resistance in prokaryotes. FEMS Microbiol Rev. 27:313–339. doi: 10.1016/S0168-6445(03)00048-2
- O'Brien A, Sharp R, Russell NJ, Roller S. 2004. Antarctic bacteria inhibit growth of food-borne microorganisms at low temperatures. FEMS Microbiol Ecol. 48:157–167. doi: 10.1016/j.femsec.2004.01.001
- Pages D, Rose J, Conrod S, Cuine S, Carrier P, Heulin T, Achouak W. 2008. Heavy metal tolerance in Stenotrophomonas maltophilia. Plos One. 3:e1539. doi:10.1371/journal.pone.000153
- Parnikoza IYu, Miryuta NYu, Maidanyuk DN, Loparev SA, Korsun SG, Budzanivska IG, Shevchenko TP, Polischuk VP, Kunakh VA, I.A. Kozeretska IA. 2007. Habitat and leaf cytogenetic characteristics of Deschampsia antarctica Desv. in the Maritime Antarctica. Polar Sci. 1:121–128.
- Perez C, Pauli M, Bazevque P. 1990. An antibiotic assay by the agar well diffusion method. Acta Biol Med Exp. 15:113–115.
- Rani MJ, Hemambika B, Hemapriya J, Kannan VR. 2010. Comparative assessment of heavy metal removal by immobilized and dead bacterial cells: A biosorption approach. Afr J Environ Sci Technol. 4:077–083.
- Ravot G, Masson JM, Lefèvre F. 2006. 34 applications of extremophiles: the industrial screening of extremophiles for valuable biomolecules. Methods Microbiol. 35:785–813. doi: 10.1016/S0580-9517(08)70037-0
- Russell NJ. 2006. Antarctic microorganisms: coming in from the cold. Culture. 27:1–4.
- Sabdono A, Radjasa OK, Utomo HS. 2012. Screening of multi-metal resistances in a bacterial population isolated from coral tissues of Central Java coastal waters, Indonesia. Int J Ocean Marine Ecol Syst. 1:11–23.
- Sánchez LA, Gomez FF, Delgado OD. 2009. Cold-adapted microorganisms as a source of new antimicrobials. Extremophiles. 13:111–120. doi: 10.1007/s00792-008-0203-5
- Sánchez LA, Hedström M, Delgado MA, Delgado OD. 2010. Production, purification and characterization of serraticin A, a novel cold-active antimicrobial produced by Serratia proteamaculans 136. J Appl Microbiol. 109:936–945. doi: 10.1111/j.1365-2672.2010.04720.x
- Séveno NA, Kallifidas D, Smalla K, van Elsas JD, Collard JM, Karagouni AD, Wellington EMH. 2002. Occurrence and reservoirs of antibiotic resistance genes in the environment. Rev Med Microbiol. 13:15–27. doi: 10.1097/00013542-200201000-00002
- Shekh RM, Singh P, Singh SM, Roy U. 2011. Antifungal activity of Arctic and Antarctic bacteria isolates. Polar Biol. 34:139–143. doi: 10.1007/s00300-010-0854-4
- Shruti M, Bali G, Saranya SK. 2012. Lead biosorption by a bacterium isolated from industrial effluents. Int J Microbiol Res. 4:196–200. doi: 10.9735/0975-5276.4.3.196-200
- Siebert J, Hirsch P, Hoffmann B, Gliesche CG, Peissl K, Jendrach M. 1996. Cryptoendolithic microorganisms from Antarctic sandstone of Linnaeus Terrace Asgard Range: diversity, properties and interactions. Biodivers Conserv. 5:1337–1363. doi: 10.1007/BF00051982
- Silver S. 1996. Bacterial resistances to toxic metal ions – a review. Genetics. 179:9–19.
- Solecka J, Zajko J, Postek M, Rajnisz A. 2012. Biologically active secondary metabolites from Actinomycetes. Cent Eur J Biol. 7:373–390. doi: 10.2478/s11535-012-0036-1
- Spain A, Alm E. 2003. Implications of microbial heavy metal tolerance in the environment. Rev Undergrad Res. 2:1–6.
- Tejedo P, Pertierra LR, Benayas J, Convey P, Justel A, Quesada A. 2012. Trampling on maritime Antarctica: can soil ecosystems be effectively protected through existing codes of conduct? Polar Res. 31:10888, http://dx.doi.org/10.3402/polar.v31i0.10888http://dx.doi.org/10.3402/polar.v31i0.10888 doi: 10.3402/polar.v31i0.10888
- Tomova I, Gladka G, Tashyrev O, Vasileva-Tonkova E. 2014. Isolation, identification and hydrolytic enzymes production of aerobic heterotrophic bacteria from two Antarctic islands. Int J Environ Sci. 4:614–625.
- Wong C, Tam H, Alias S, González M, González-Rocha G, Domínguez-Yévenes M. 2011. Pseudomonas and Pedobacter isolates from King George Island inhibited the growth of foodborne pathogens. Polish Polar Res. 32:3–14. doi: 10.2478/v10183-011-0003-y
- Zhang XX, Zhang T, Fang HHP. 2009. Antibiotic resistance genes in water environment. Appl Microbiol Biotechnol. 82:397–414. doi: 10.1007/s00253-008-1829-z