ABSTRACT
DNA nanostructures with molecular recognition qualities have been developed, but the conceptualization of DNA-based molecular nanoelectronics is still a thought-provoking subject. An efficient and speedy charge transfer (CT) process through DNA nanoassembly is demanded for farther exploitation of DNA nanoelectronics with programmable features. The CT properties are represented in terms of localization lengths. Because of the DNA molecule’s unique and novel characteristics, it can be applied in a variety of multidisciplinary research areas such as nanobiomedicine, nanooptoelectronics and nanobiotechnology. By using this interesting phenomena, we can integrate nanotechnology with both, biology as well as engineering, and can use it as a tool for many biological and engineering applications such as DNA chips, DNA nanogrids and DNA nanoribbons. Here, we have presented a review on various experiments that measure CT and charge transport in DNA. It is a very wide and interesting area in which many scientists have published many articles. So here we have tried to show the whole picture of it.
Introduction
The integration of nanotechnology with biological science and bioengineering is producing much advancement. DNA has been used extensively to form nanoscale structures that may be used as nanotechnology devices in the future (Mujica et al. Citation2000; Carroll & Gorman Citation2002; Osakada et al. Citation2006). Though large number of DNA nanostructures has been constructed, the realization of DNA-based molecular nanoelectronics is still challenging since its chemical and physical properties continue to remain unclear (Carroll & Gorman Citation2002).
Structurally manipulated DNA motifs, which are also called as DNA tiles, have been used by many researchers as building blocks for the creation of DNA-based nanostructures. Such DNA tiles are linked together with a branched junction, called sticky-end DNAs, which are employed for self-assembling nanostructures, such as two- or three-dimensional DNA nanostructures. These self-assembling nanostructures can be originated by mixing of short single strands of the DNA (Osakada et al. Citation2006). By using these functionalized DNAs as building blocks, the conception of functionalized DNA nanostructures could be accomplished, resulting in generation of DNA-based nanotechnologies and nanoelectronics (Carroll & Gorman Citation2002). In its natural surroundings, DNA remains in liquid solution, and hence, experimentally, DNA molecule can be studied either in solution form or in artificially enforced dry environments. In experiments based on solution, chemically processed DNA is used in order to host with a donor and an acceptor molecule at different places along its long axis. The electron transport in DNA is a very important process in biomolecules and other large polymeric systems. Some have told that DNA is a molecular wire that can carry charges with no resistance. However, some find DNA as an insulator (Mujica et al. Citation2000; Dekker & Ratner Citation2001; Carroll & Gorman Citation2002) (Table ).
Table 1. Advantages of DNA for the charge transport.
Functioning of charge transfer (CT)
DNA double-helix structure consists of two strands, and basically they have four bases: 1. guanine (G), 2. cytosine (C), 3. adenine (A), 4. thymine (T). DNA molecules recognize other DNA molecules by their base-pairing relationship. e.g.: A prefers to pair with T and G prefers to pair with C (Seeman Citation1998, Citation2003).
The hydrogen bonding brings the DNA molecules closer together. In order to bond to other strands, the double helix has a single stranded overhang (sticky end) that will form hydrogen bond with complimentary strand (Seeman Citation1998, Citation2003) (Figure ).
Figure 1. The DNA double-helix strands consist of two linear strands with four bases – guanine (G), cytosine (C), adenine (A) and thymine (T). The bases A on the one strand pair up with the complementary bases T on the other strand, while G pairs up with C. Electron transport experiments on DNA molecules have donor and acceptor groups added at each end. In such experiments, the DNA molecules are sandwiched between two metal electrodes (E1 and E2). adapted from Dekker and Ratner (Citation2001).
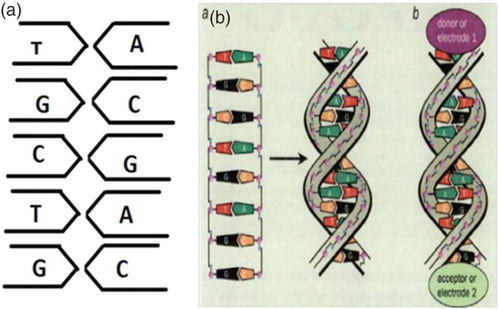
In the experiments of electron/charge transport in DNA, there are two groups: donor and acceptor. We can also call them as electrodes of a nanowire (if we consider DNA as a nanowire) (Boon & Barton Citation2002). So at the ends of the DNA double helix, at one end the donor group will be attached (electrode 1) and at the another end of the DNA double helix the acceptor(electrode 2) will be attached (Jortner et al. Citation1998; Boon & Barton Citation2002). Here, we can also say that the DNA molecules are sandwiched between the two donor and acceptor groups (Jortner et al. Citation1998).
The donor is a site along the base stack, where a charge is intentionally interposed into the structure, and ‘hole trapping’ site cognized as acceptor site at a distance (Ingold & Nazarov Citation1992; Berlin et al. Citation2001; Kawai et al. Citation2001; Yu & Song Citation2001).
The interpretation of the charge migration is generally given in terms of the change of localization site for the hole (Olofsson & Larsson Citation2001; Troisi & Orlandi Citation2001). The inherent structure of the molecule is compromised by the transfer process, in the sense that the charge state at distinct sites beside the helix before and after the hole migration is different (Olofsson & Larsson Citation2001). In these experiments, there is no tunnelling barrier for the charge to overcome when injected into the molecule (Ingold & Nazarov Citation1992; Yu & Song Citation2001). The solid state experiments are based on several techniques such as imaging, spectroscopy and measurements of electrical transport that divulge the electric current flux through the molecule under the influence of an external field. The donor and the acceptor act as reservoirs of charges and this conception renders to pass on the charge state along the unaltered helix.
It is not specified if the mobile charges are electrons or holes: this counts on the accessibility of electron states, on their occupancy, and on the alignment to the Fermi levels of the reservoirs (Giese et al. Citation2001). Extensive experimental and theoretical studies have shown that electron transfer reaction can occur by two basic mechanisms: coherent process or tunnelling and thermal hopping or one step of super exchange or multistep hopping (Ingold & Nazarov Citation1992; Giese et al. Citation2001; Yu & Song Citation2001) (Table ).
Table 2. Difference between coherent and thermal hopping.
In coherent/tunnelling one, electron tunnelling, a single step process from the donor to acceptor in which there is no exchange of energy between electron and biomolecule during the transfer so the electron is never localized, because of this sense this process is also called as coherent. Here, rate of such reactions decreases exponentially with distance between donor and acceptor. So, for long DNAs, one refers to this type of the transport mechanism which can be insignificant on any reasonable time scale (Olofsson & Larsson Citation2001; Klotsa et al. Citation2005; Macía et al. Citation2005).
The other mechanism for electron transfer is called as thermal hopping or we can also call that as hopping mechanism or multistep hopping or incoherent process. Electron is localized on the molecule and exchanges energy with it. It is done in multistep mechanism from the donor to acceptor. This type of hopping can transfer the electron over far longer distance than the coherent mechanism (Hall et al. Citation1996; Grinstaff Citation1999; Delaney & Barton Citation2003; Porath et al. Citation2004) (Figure ).
Figure 2. Charge carriers hop from the G-C base pair to the next G-C pair. The figure clearly shows that the A-T base pairs have higher energy than the G-C base pairs; so they act as a barrier to the charge transport. From one G-C base pair to the next G-C pair, time travelled by a charge – t12, relatively for the second G-C pair to the next G-C pair – t23 and so on.
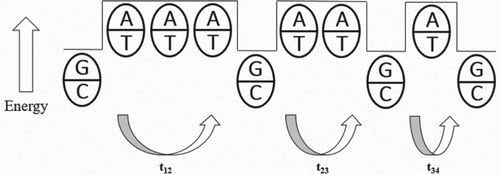
Charge carriers hop along the length of the DNA molecules from one G-C base pair to the next. Here, the energies of G-C and A-T in DNA sequence show that a positive charged hole has a lower energy on the G-C sites and moves from one G-C pairs to the next by tunnelling, which is coherent through the A-T sites. Here, charged carrier is localized along the path. The total time it takes to move along the path is the sum of the times it takes to jump between the individual G-C base pairs (Storhoff & Mirkin Citation1999; Giese Citation2000; Berlin et al. Citation2000; Boon et al. Citation2003; Delaney & Barton Citation2003).
Here, to understand why the DNA sequence makes a difference, the comparison of the relative energies of A-T and G-C pairs is required, which is done by
A hole which is considered as a positive charge is more stable on a G-C base pair than on an A-T base pair (Giese Citation2000). We can say from some experiments that the energy difference between two pairs is larger than the thermal energy of a charge carrier (Barnett Citation2001; Schuster Citation2004). So under such conditions, basically the hole will localize on G-C pair. As the A-T base pair have higher energy; so they act as a barrier to the hole transfer. The hole can tunnel in a coherent form from the first G-C spot to the second one. It can either go on to the further G-C spot or can hop back to the 1st one (Giese Citation2002).
Here, coherent CT rate decreases exponentially with increase in travelled distance. When the distance between G-C becomes too long for CT, then thermal hopping mechanism takes place and charge carriers jump efficiently (Wang et al. Citation2004; Ortmann et al. Citation2009).
Charge transport in DNA is governed by several mechanisms such as electronic conduction alongside of base pair sequences, ionic conduction coupled with the counter ions, reorientation of the water dipoles around the duplex and phonon sensing due to the structural fluctuations of the duplex.
Charge transport in DNA has great biological importance because of extended electronic states of the DNA, which can help in the processes of biological sensing as well as DNA damage repairing.
With the help of various base-pairing pattern studies, some researchers studied the localization of the hole and also the transportation of the charge through the DNA pairs. It showed that the CT was sequence dependent, and localization of oxidative damage can be studied with the help of the electrical behaviour of the different pairs. For example, DNA sequence with non Watson and Crick pairs has lower conductivity than with a fully W-C paired DNA sequence (Ortmann et al. Citation2009).
So basically, if we try to compare such base-pairing patterns of oligonucleotides, for example, natural W-C paired oligonucleotides and oligonucleotides with perturbations, we can get some interesting results. Compared to the natural W-C paired sample, mismatch sample shows a decrease in the conductivity and smaller structural disturbances (Wang et al. Citation2004).
Another approach to modify DNA properties is to make up DNA–metal multifaceted structure. The way of charge transport through such structure is an important technique for the characterization of this DNA–metal system. For example, thymine–mercury–thymine can be used as an important system for the designing of the application in DNA-based nanobiosensors (Rawtani & Agrawal, Citation2012, Citation2013, Citation2014).
Here also if we try to compare the efficiency of different DNA-pairing samples such as natural standard DNA, DNA with mismatched base pair and DNA–metal multifaceted structure, it shows that DNA–metal system has lowest CT efficiency than the natural standard DNA sample. As the temperature of the structures increases, the frequency of the CT also increases (Boon et al. Citation2002; Roche et al. Citation2003; Takada et al. Citation2004).
For such experiments, some general techniques can be used such as scanning tunnelling microscopy, UV–visible spectroscopy, Raman spectroscopy and steady-state fluorescence. Computer modelling and theoretical approaches such as molecular dynamics and quantum mechanics were also studied.
Concept of CT procedure
CT rate through the (G-C)n sequence is rapid. The long-range CT through DNA nanoscaled assembly mainly consists of (G-C)n sequence fabricated with DNA nanoscaled assembly by using three DNA blocks such as charge injection block (donor-group-labelled block), charge-conductive block and charge-detection block (acceptor-group-labelled block). These groups are considered for the effective charge injection with a consecutive A sequence and a rapid charge migration with the (G-C)n sequence. Basically all three groups are linked by two sticky ends. After hybridization, the CT through the DNA made by the three DNA building blocks was observed by monitoring the formation of the acceptor group or E2 (Figure ).
Mathematical model
From the perspective of biology and genetics, long-range charge transport is too significant as it relates intimately to mutation, base damage and repair. Oxidation potential of A, T, G and C is respectively 1.97, 2.11, 1.49 and 2.14 V. As G has the lowest oxidation potential, it oxidizes most easily and produces hole. For DNA’s engineering application and biological interest, several studies have been devoted to electron conductivity of DNA by experimental approach. Detailed mechanism of charge transport in DNA is a not a far-famed subject; so studies pertaining to theoretical considerations should be made in detail to overcome these problems (Kawano & Maruyama Citation2005).
General model for the derivation of charge migration of biomolecules, mostly proteins and DNAs, it contain multiple time scales which have been derived quantum mechanically.
where (n, m) denote the sites of proteins and (i, j) represent the rotational states of the protein chain. Cni represents the probability to find the charge at the nth site of the biological molecule (Giese & Spichty Citation2000).
Kinetic modelling
Analysis of all the experiments and the results of it can also be done by the MATLAB software.
Here, two types of kinetic models are given from which simultaneous differential equations can be obtained (Nunez et al. Citation1999) (Figure ).
Figure 4. Kinetic schemes where E1 is donor group and E2 is acceptor group. These two models show that the different patterns of charge migration (Osakada et al. Citation2006).
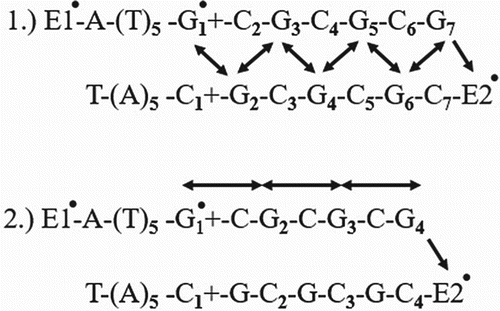
A simultaneous differential equation obtained can be represented as
where
and [E1] correspond to the charge population at G and acceptor-group sites, respectively, k is the CT rate constant between Gs, and k1 is the rate constant for the CT form G•+ to acceptor group. We can also calculate relative energies of the base pairs.
Dynamic behaviour and effect of water
The dynamics of electrons and holes is much more complex than the originally proposed band like showing of charge movement by ‘π-path ways’ offered by interactions among heterocycles of base pairs (Lewis et al. Citation2003).
Theoretical analysis of descriptive steady-state dimensions of the sequence reliance of the hole efficiency has led to the formulation of a model that treats charge movement as a set of variable range hops between two adjacent base pairs.
Essential information involves only the experimental data for such predictions on relative rates for hopping steps of different lengths of the base pairs. The implication of relative (not absolute) rates of theoretical analysis for steady-state experiments is a direct consequence of two competitive decay channels existing for a hole at each step of the charge movement process (hole transfer between nearest – neighbour G sites through the A-T bridge) and irreversible side reaction of the G+ (cation) with water (Schlag et al. Citation2000a; You et al. Citation2004).
Knowledge of relative hopping rates is deficient to decide how fast a hole generated in DNA can be transported over a definite distance (Conwell & Rakhmanova Citation2000) (Figure ).
Figure 5. Charge transfer in DNA base pairs after photoexcitations of linker by a laser pulse. A hole firstly undergoes a transition from photoexcited linker to the adjacent G-C pair, as shown in the thick arrow. Then, it can either hop to next G-C pairs [thin line] or return to linker with the subsequent electron-hole recombination [thin line].
![Figure 5. Charge transfer in DNA base pairs after photoexcitations of linker by a laser pulse. A hole firstly undergoes a transition from photoexcited linker to the adjacent G-C pair, as shown in the thick arrow. Then, it can either hop to next G-C pairs [thin line] or return to linker with the subsequent electron-hole recombination [thin line].](/cms/asset/3ef085c8-f6fd-4496-9d85-84fb7dc64069/tfls_a_1207570_f0005_b.gif)
After certain theoretical examinations, it has been notified that the inconsistency between theoretical and experimental data of the hopping rate is due to the underestimation of localization phenomena in the characterization of charge movement actions. This phenomenon can result from the coupling of electronic motion to vibrational dynamics of base pairs and can also be caused by polarization of the molecule or solvent (Schlag et al. Citation2000a; Lewis et al. Citation2000, Citation2002). These studies conclude that self-trapping of a hole leads to the formation of a large polaron that spreads in lengths over 5–6 base pairs and moves together with polarized surroundings along the stack of the base pairs. Further the competition formation between quantum delocalization of charges and localization of charges due to vibration or solvation forces, consequently the formation of rather compact hole states with the typical dimensions less than 7 angstrom (small polaron). The response of solvent to charge motion favouring the adiabatic mechanism of hole transfer over the nonadiabatic mechanism (Kurnikov et al. Citation2002).
Figure 6. Schematic representation of double helical B-form DNA. The linearly arranged array of p-stacked aromatic heterocycles is depicted in grey; the sugar-phosphate backbone is coloured black: (a) view perpendicular to the helical axis and (b) view down the helical axis. Adapted from Treadway et al. (Citation2002).
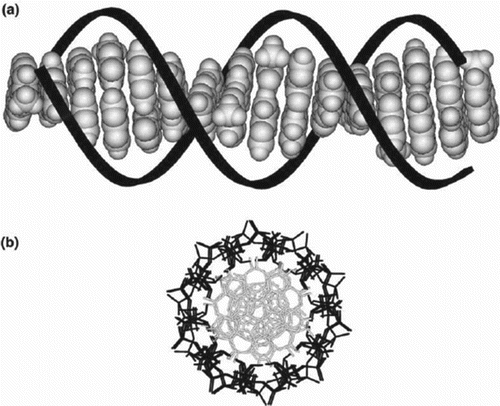
Theoretical framework for study the influence of solvent polarization on resultant charge transport
The Hamiltonian for the electron movement along a DNA strand consists of three parts (Peyrard Citation2004):
where
signifies particle charge transport due to base pairs,
accounts the dynamics of H-bond vibrations and
represents dynamics proportional to twisted angle between two successive base pairs ().
Sequential electronic motion can be described in terms of the tight-binding Hamiltonian (Peyrard Citation2004):
Here
ΔE = the difference in ionization potentials of A-T and G-C base pairs
b = transfer integral
= creation operator for a hole at the ith site
= annihilation operators for a hole at the ith site
Index i marks DNA base pairs in the sequence, and the sum is taken over G-C sites only.
To give an account of interaction between charge– solvent, a multi-dimensional coordinate has been introduced that characterizes the polarization of water molecules around the kth base pair (Peyrard Citation2004).
Then a standard linear response enables one to express the interaction with the water environment as function of as
The interaction with water is ascertained by the site populations and by matrix A with elements
(which can be specified if solvation energies for oxidized states of G bases in DNA are known).
where θnn−1 is the relative angle between two adjacent base pairs which measure displacements from its equilibrium twist angle θ0 and Jn the reduced moment of inertia.
Energetics of the relative base pairs
Energetics of the relative base pairs of DNA can be obtained by the two equations given below (Gust et al. Citation1993):
Here
T = absolute temperature
kb = Boltzman constant
ΔG = thermodynamic driving force.
Differential studies pertaining to CT
Several studies pertaining to CT were performed. Giese and coworkers have demonstrated that the CT between guanine (G) sites happens due to multihopping mechanism. They also showed that CT between Gs segregated by (A:T)n (n 4) sequences can take place because the adenines (As) also act as the charge carriers. Further, Barton and O’Neill also proved that CT through a DNA is described as conformational gated hopping among stacked domains. The long-range CT between Gs across A-T base pairs occurs in a slow time scale, microsecond to millisecond range. In another study, Porath and coworkers put poly(dG)-poly(dC) DNA only at distance of 8 nm by applying electrostatic trapping technique. All these studies successfully demonstrated that DNA conducts as a large gap of semiconductor.
Biological applications
One of the important applications of assembly of DNA is in so-called DNA chips. They can be used as a probe to see whether certain genetic codes are present in the given specimen of DNA (Fasman Citation1975; Giese Citation2000). These microfabricated chips with many parallel DNA probes are becoming wide spread in medical and analytical diligences. Currently, the chips are reading out optically but further miniaturization might necessitate new read out schemata, possibly involving the electron transfer properties of DNA. Such strategies might feat the different electrochemical responses of single and double stranded DNA molecules that attach to the surface (Fink & Schönenberger Citation1999; LaBean et al. Citation2000; Schlag et al. Citation2000b; Hu et al. Citation2002; Niemeyer Citation2002; Liu et al. Citation2003; Wengel Citation2004; Xu et al. Citation2005). These radically distinctive assembly properties of DNA unitedly with its unparalled recognition, optical characteristics, stability and adaptability propose that DNA may become one of the most significant species in the general area of molecular electronics (Vázquez de Parga et al. Citation1998; Aerschot et al. Citation2001; Rakitin et al. Citation2001).
Holliday junction: Branched DNA is employed to make various DNA complexes. As shown in Figure , the four strands of DNA get combined and make one Holliday junction (Rothemund Citation2006a). In this structure, the base pairs on one (green) molecule bind together with the fragments of other two (red and blue) molecules that have the complementary bases. When the fourth fragment is added, which is shown as yellow, which results as a cross. Using such mechanism, we can make different structures such as cubes.
Further evidence suggesting that DNA charge transport could be biologically relevant derives from understanding with iron–sulphur cluster bearing DNA repair proteins. For instance, when it is bound to DNA, the redox potential of the [4Fe-4S] cluster present in proteins is observed to be altered so that the protein is more easily oxidized. By comparing potentials of DNA as well as free DNA, the binding affinity of the oxidized form is estimated and found to be at least three times higher in magnitude than that of the reduced form (Schleif Citation1988; Travers Citation1989; Rothemund Citation2006a).
In reduced state, a repair protein binds to DNA and becomes easily oxidized to transfer an electron to other repair protein bound at a distal site of DNA, hence reduction of distally bound DNA and subsequently elevating its further dissociation (Rothemund, Citation2006b). But this DNA-governed reaction can only happen if the intervening DNA base stack is either intact or intimately well stacked; if not, the protein remains associated with the DNA and can progressively migrate to the damaged site (Travers Citation1989).
DNA-driven charge transport consequently serves the function to spread the repair proteins in regions of damage within the genome. DNA-mediated charge transport essentially serves the function of redistribution of repair proteins to a damage site, which basically provides a way for protein to scan bigger regions of DNA without physically binding to each base. Importantly, this scanning plays a pivotal role under the oxidative stress conditions (Yavin et al., Citation2005), when, due to excessive stress, guanine radicals are formed. Basically, flash-based quench method with ruthenium as an intercalator display that guanine radicals provide not only signal for repair but also promote the oxidation of DNA repair proteins in a DNA-mediated fashioned (Schwögler & Carell Citation2000; Niemeyer Citation2001; Bjork et al. Citation2002; Benenson et al. Citation2003; Bauschlicher & Lawson Citation2007) (Figure ).
G-quadruplexes(G4-DNA)
Current developments in the field of CT such as G4 quadruplexes, DNA–nanotube interactions and atomistic simulations of DNA have attracted much attention of the researchers. G4-DNA (four-stranded helical structures) has an important role in regulation of various biological processes in vivo, but as far as in vitro is concerned, peculiarity has been shifted towards its interesting structure.
Four-stranded helical structures known as G4-DNA is formed in nucleic acids by sequences that are rich in guanine. The sequencing of many genomes has disclosed that they have potential to form G-quadruplexes owing to rich in sequence motifs (Woiczikowski et al. Citation2010). Four guanine bases associate to form square planar structures through Hoogsten Hydrogen Bonding, which is called as guanine-tetrad. They stack on each other to form G-quadruplexes. With the help of the direction of the tetrads, we can get to know whether the structure is parallel or anti-parallel (Tucker et al. Citation2012).
Charge transport in G-quadruplex DNA molecule obviously requires a donor group, which forms the structure donor-G4-DNA. This structure is more stiffer and transport charges over longer distances because of G-rich content. It also has higher rigidity than the double-stranded DNA base pairs but the overall structure is robust (Tucker et al. Citation2012).
Due to limitation to act as a substrate for telomerase, G-quadruplex DNA inhibits telomerase activity; therefore, in recent times, research interest shifted towards designing of G-quadruplex-ligands that inhibit telomerase activity either via induction or stabilization of a G-quadruplex.
In each and every individual, as a specialized DNA sequence, telomeres are needed for many crucial biological and physiological processes such as cell replication, genetic stability, ageing, DNA damage and cancer. Usually telomere gets shortened with each cell replication in normal cells and subsequently when a critical length of chromosomes is achieved, cells undergo apoptosis. Nonetheless, most of the cancer cells (around 80–90%) usually maintain their telomere length by activation of telomerase and hence become abnormal (Tucker et al. Citation2012).
The formation of Telomere-G4 slows down telomerase activity, which basically obstructs tumour tissue immortalization mechanism. Hence, much of research interest has been switched towards exploitation of TG4 as an attractive target for cancer therapeutic (Tucker et al. Citation2012).
Moreover, recent bioinformatics studies showed that about 370,000 putative G-quadruplex-forming sequences exist throughout the human genome (Yaku et al. Citation2012) (Figure ).
Figure 8. Structure of a G-tetrad (quadruplex). Adapted from Bidzinska et al. (Citation2013).
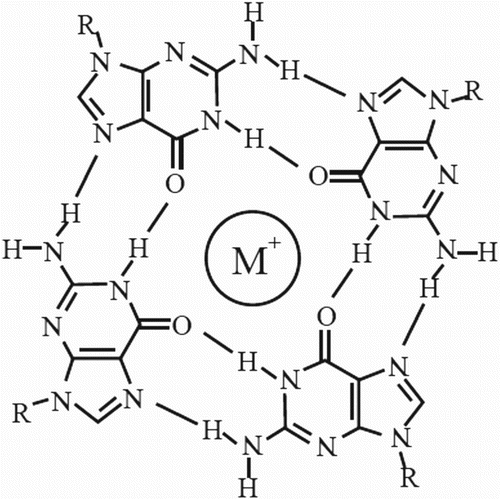
Research establishes that this group is deposited onto the hard substrate because of its polarizability and it can transport current over longer distance compared to other structures (Zhao et al. Citation2004). Due to DNA’s self-assembling properties such as pairing specificity and conformational flexibility suggest their greater potential for designing of DNA-based nanostructures and DNA-based nanoelectonic devices. Hence, different topological structures of G4-DNA render more possibilities towards fabrication of nanodevices (Wang & Liang Citation2015).
G4 model
G4 hypothesis suggests that two respective ends of G4-DNA attached with two semi-infinite one-dimensional electrodes (Wang & Liang Citation2015).
The Hamiltonian equation for G4-DNA model defined as
where
stands for electrodes at the left and right ends of the G4-DNA;
shows the coupling between electrodes and G4-DNA and
corresponds to the environmental effects due to stochastic variation of the G4 structure (Wang & Liang Citation2015).
The tm depicts amplitude due to hopping between Gs in G4 packet; The tn,n+1 is the hopping parameters due to nearest electron; and the
is the creation (annihilation) operator of electron or hole at k sites. The
represents on-site energy (hopping parameter) in the electrode, correspondingly (Di Felice Citation2015).
The numeric studies demonstrate G4-DNA impelled charge transport that is based on basic mono-G tight-binding G4 hypothesis.
Owing to cationic effect, CT in G4-DNA is found to be greater extent from normal DNA with the presence of hydrogen bond in G4-DNA. Comparison of various functions pertaining to topological structures of G4 by biomedical methods is quite problematic (Kumarasamy & Sun Citation2013). Alternatively, physical methods not only give insight about the physiological mechanism of G4 functions but also CT of nanodevices (Choi et al. Citation2013).
CT and base-pairing patterns
In charge transport in DNA, contribution of some mechanisms such as electronic conduction beside base pair sequences, ionic conduction coupled with the counter ions, reorientation of the water dipoles around the duplex and phonons resulting from the structural fluctuations of the duplex. In the charge transport DNA has great biological importance because of extended electronic states of the DNA which can help in the processes of biological sensing as well as DNA damage repairing. Functioning of DNA with the biological as well as the man-made polymers, ability to preserve, transfer and transmit information, which make them exceptional (Kratochvılova et al. Citation2010). With the help of various base-pairing pattern studies, some researchers studied the localization of the hole and also the transportation of the charge through the DNA pairs. It showed that the CT was sequence dependent and with the help of the electrical behaviour of the different pairs, localization of oxidative damage can be studied. For example, DNA sequence with non-W-C pairs has lower conductivity when compared with a fully W-C-paired DNA sequence (Drummond et al. Citation2003). So basically, if we try to compare such base-pairing patterns of oligonucleotides, for example, natural W-C paired oligonucleotides and oligonucleotides with perturbations, we can get some interesting results. Compared to the natural W-C paired sample, mismatch sample shows decrease of the conductivity and smaller structural disturbances (Sessler & Jayawickramarajah Citation2005).
Another approach to modify DNA properties is to compose DNA–metal multifaceted structure. The way of charge transport through such structure is an important technique for the characterization of this DNA–metal system. For example, thymine–mercury–thymine can be used as an important system for the designing of the application in DNA-based nanobiosensors (Kratochvílova et al. Citation2014).
Here also if we try to compare the efficiency of different DNA-pairing samples such as natural standard DNA, DNA with mismatched base pair and DNA–metal multifaceted structure, it shows that DNA–metal system has the lowest CT efficiency than the natural standard DNA sample. As the temperature of the structures increases, the frequency of the CT also increases (Clever & Shionoya Citation2010).
For such experiments, some general techniques can be used such as scanning tunnelling microscopy, UV–visible spectroscopy, Raman spectroscopy, and steady-state fluorescence. Computer modelling as well as theoretical approaches such as molecular dynamics and quantum mechanics were also studied (Dieter-Wurm et al. Citation1992; Joseph & Schuster Citation2007; Takezawa & Shionoya Citation2012).
Conclusion
A more rapid and efficient CT process through the DNA nanoassembly is expected for further development of programmable DNA nanoelectronics. The charge migrates through DNA by a hopping process. Each hopping step depends strongly upon the hopping distance. However, very long-distal charge transport is possible because the total distance is fall apart and the longest step acquire to be rate determining. Two types of hopping processes (coherent and thermal) are depicted. This article elaborates about various types of models (mathematical, kinetic and dynamic), with the help of which, theoretical as well as practical data of positive charges (holes) and electrons can be easily examined and determined. This term can be applied not only in nanoelectronics but also in many different biological applications. In future, the constructive idea for sensing and also for the detection of any particular DNA sequence, this expression of transporting the charge through the base pairs of DNA will be very utilitarian and it will also be helpful in modern application of genetics. This paper provides the reader overall information about the phenomena of CT in DNA and that too in the easiest way.
Disclosure statement
No potential conflict of interest was reported by the authors.
References
- Aerschot AV, Meldgaard M, Schepers G, Volders F, Rozenski J, Busson R, Herdewijn P. 2001. Improved hybridisation potential of oligonucleotides comprising O-methylated anhydrohexitol nucleoside congeners. Nucleic Acids Res. 29:4187–4194. doi: 10.1093/nar/29.20.4187
- Barnett RN. 2001. Charge migration in DNA: ion-gated transport. Science. 294:567–571. doi: 10.1126/science.1062864
- Bauschlicher CW, Lawson JW. 2007. Current-voltage curves for molecular junctions: effect of substitutients. Phys Rev B. 75:115406–115411. doi: 10.1103/PhysRevB.75.115406
- Benenson Y, Adar R, Paz-Elizur T, Livneh Z, Shapiro E. 2003. DNA molecule provides a computing machine with both data and fuel. Proc Natl Acad Sci USA. 100:2191–2196. doi: 10.1073/pnas.0535624100
- Berlin YA, Burin AL, Ratner MA. 2000. On the long range charge transfer in DNA. J Phys Chem A. 104:443–445. doi: 10.1021/jp9933323
- Berlin YA, Burin AL, Ratner MA. 2001. Charge hopping in DNA. J Am Chem Soc. 123:260–268. doi: 10.1021/ja001496n
- Bidzinska J, Cimino-Reale G, Zaffaroni N, Folini M. 2013. G-Quadruplex structures in the human genome as novel therapeutic targets. Molecules. 18:12368–12395. doi: 10.3390/molecules181012368
- Bjork MT, Ohlsson BJ, Thelander C, Persson AI, Deppert K, Wallenberg LR, Samuelson L. 2002. Nanowire resonant tunneling diodes. Appl Phys Lett. 81:4458–4460. doi: 10.1063/1.1527995
- Boon EA, Livingston AL, Chmiel N, David S, and Barton J. 2003. DNA mediated charge transport for DNA repair. Proc Natl Acad Sci USA. 100:12543–12547. doi: 10.1073/pnas.2035257100
- Boon EM, Barton JK. 2002. Charge transport in DNA. Curr Opin Struct Biol. 12:320–329. doi: 10.1016/S0959-440X(02)00327-5
- Boon EM, Barton JK, Pradeepkumar PI, Isaksson J, Petit C, Chattopadhyaya JB. 2002. An electrochemical probe of DNA stacking in an antisense oligonucleotide containing a C3′-endo-locked sugar. Angew Chem Int Ed. 41:3402–3405. doi: 10.1002/1521-3773(20020916)41:18<3402::AID-ANIE3402>3.0.CO;2-6
- Carroll RL, Gorman CB. 2002. The genesis of molecular electronics. Angew Chem Int Ed Engl. 41:4378–4400. doi: 10.1002/1521-3773(20021202)41:23<4378::AID-ANIE4378>3.0.CO;2-A
- Choi J, Park J, Tanaka A, Park MJ, Jang YJ, Fujitsuka M, Kim SK, Majima T. 2013. Hole trapping of G-Quartets in a G-Quadruplex. Angew Chem Int Ed. 52:1134–1138. doi: 10.1002/anie.201208149
- Clever GH, Shionoya M. 2010. Metal–base pairing in DNA. Coord Chem Rev. 254:2391–2402. doi: 10.1016/j.ccr.2010.04.014
- Conwell EM, Rakhmanova SV. 2000. Polarons in DNA. Proc Natl Acad Sci USA. 97:4556–4560. doi: 10.1073/pnas.050074497
- Dekker C, Ratner MA. 2001. Electronic properties of DNA. Phys World. 14:29–33. doi: 10.1088/2058-7058/14/8/33
- Delaney S, Barton JK. 2003. Long-range DNA charge transport. J Org Chem. 68:6475–6483. doi: 10.1021/jo030095y
- Dieter-Wurm I, Sabat M, Lippert B. 1992. Model for a platinated DNA triplex: Watson-Crick and metal-modified Hoogsteen pairing. J Am Chem Soc. 114:357–359. doi: 10.1021/ja00027a054
- Di Felice R. 2015. 122 Optimizing charge transfer through G-quadruplex. J Biomol Struct Dyn. 33:77. doi: 10.1080/07391102.2015.1032755
- Drummond TG, Hill MG, Barton JK. 2003. Electrochemical DNA sensors. Nature Biotechnol. 21:1192–1199. doi: 10.1038/nbt873
- Fasman GD. 1975. Handbook of biochemistry and molecular biology—nucleic acids. 3rd ed. Boca Raton, FL: CRC Press.
- Fink HW, Schönenberger C. 1999. Electrical conduction through DNA molecules. Nature. 398:407–410. doi: 10.1038/18855
- Giese B. 2000. Long-distance charge transport in DNA: the hopping mechanism. Acc Chem Res. 33:631–636. doi: 10.1021/ar990040b
- Giese B. 2002. Long-distance electron transfer through DNA. Annu Rev Biochem. 71:51–70. doi: 10.1146/annurev.biochem.71.083101.134037
- Giese B, Amaudrut J, Köhler AK, Spormann M, Wessely S. 2001. Direct observation of the hole transfer through DNA by hopping between adenine bases and by tunneling. Nature. 412:318–320. doi: 10.1038/35085542
- Giese B, Spichty M. 2000. Long distance charge transport through DNA: quantification and extension of the hopping model. Chem Phys Chem. 1:195–198.
- Grinstaff MW. 1999. How do charges travel through DNA?—An update on a current debate. Angew Chem Int Ed. 38:3629–3635. doi: 10.1002/(SICI)1521-3773(19991216)38:24<3629::AID-ANIE3629>3.0.CO;2-4
- Gust D, Moore TA, Moore AL. 1993. Molecular mimicry of photosynthetic energy and electron transfer. Acc Chem Res. 26:198–205. doi: 10.1021/ar00028a010
- Hall DB, Holmlin RE, Barton JK. 1996. Oxidative DNA damage through long-range electron transfer. Nature. 382:731–735. doi: 10.1038/382731a0
- Hu J, Zhang Y, Gao H, Li M, Hartmann U. 2002. Artificial DNA patterns by mechanical nanomanipulation. Nano Lett. 2:55–57. doi: 10.1021/nl0156336
- Ingold GL, Nazarov YuV. 1992. Charge tunneling rates in ultrasmall junctions in single charge tunneling. New York, NY: Plenum; p. 21–107.
- Jortner J, Bixon M, Langenbacher T, Michel-Beyerle ME. 1998. Charge transfer and transport in DNA. Chemistry. 95:12759–12765.
- Joseph J, Schuster GB. 2007. Long-distance radical cation hopping in DNA: the effect of Thymine−Hg(II)−Thymine base pairs. Org Lett. 9:1843–1846. doi: 10.1021/ol070135a
- Kawai K, Takada T, Tojo S, Ichinose N, Majima T. 2001. Observation of hole transfer through DNA by monitoring the transient absorption of pyrene radical cation. J Am Chem Soc. 123:12688–12689. doi: 10.1021/ja0158152
- Kawano S, Maruyama Y. 2005. Mathematical model for polaronic effects of charge transport in DNA. JSME Int J Ser B. 48:456–463. doi: 10.1299/jsmeb.48.456
- Klotsa D, Römer RA, Turner MS. 2005. Electronic transport in DNA. Biophys J. 89:2187–2198. doi: 10.1529/biophysj.105.064014
- Kratochvílova I, Golan M, Vala M, Špérová M, Weiter M, Páv O, Šebera J, Rosenberg I, Sychrovský V, Tanaka Y, Bickelhaupt FM. 2014. Theoretical and experimental study of charge transfer through DNA: impact of mercury mediated T-Hg-T base pair. J Phys Chem B. 118:5374–5381. doi: 10.1021/jp501986a
- Kratochvılova I, Todorciuc T, Kral K, Nemec H, Buncek M, Sebera J, Zalis S, Vokacova Z, Sychrovsky V, Bednarova L, et al. 2010. Charge transport in DNA oligonucleotides with various base-pairing patterns. J Phys Chem B. 114:5196–5205. doi: 10.1021/jp100264v
- Kumarasamy VM, Sun D. 2013. Current and future views in G-Quadruplex secondary structure as an anti-cancer target. Sabin J Phys Chem Biophys. 3:5.
- Kurnikov IV, Tong GSM, Madrid M, Beratan DN. 2002. Hole size in oxidized double helical DNA: competition between quantum delocalization and solvation localization energies. J Phys Chem B. 106:7–10. doi: 10.1021/jp0132329
- LaBean TH, Yan H, Kopatsch J, Liu FR, Winfree E, Reif JH, Seeman NC. 2000. Construction, analysis, ligation and self-assembly of DNA triple crossover complexes. J Am Chem Soc. 122:1848–1860. doi: 10.1021/ja993393e
- Lewis FD, Liu X, Liu J, Miller SE, Hayes RT, Wasielewski MR. 2000. Direct measurement of hole transport dynamics in DNA. Nature. 406:51–53. doi: 10.1038/35017524
- Lewis FD, Liu X, Miller SE, Hayes RT, Wasielewski MR. 2002. Dynamics of electron injection in DNA hairpins. J Am Chem Soc. 124:11280. doi: 10.1021/ja026751d
- Lewis JP, Cheatham TE, Starikov EB, Wang H, Sankey OF. 2003. Dynamically amorphous character of electronic states in poly(dA)-poly(dT) DNA. J Phys Chem B. 107:2581–2587. doi: 10.1021/jp026772u
- Liu H, Gao J, Lynch SR, Saito YD, Maynard L, Kool ET. 2003. A four-base paired genetic helix with expanded size. Science. 302:868–871. doi: 10.1126/science.1088334
- Macía E, Triozon F, Roche S. 2005. Contact-dependent effects and tunneling currents in DNA molecules. Phys Rev B. 71:113106. doi: 10.1103/PhysRevB.71.113106
- Mujica V, Roitberg AE, Ratner M. 2000. Molecular wire conductance: electrostatic potential spatial profile. J Phys Chem. 112:6834–6839. doi: 10.1063/1.481258
- Niemeyer CM. 2001. Nanoparticles, proteins, and nucleic acids: biotechnology meets materials science. Angew Chem Int Ed. 40:4128–4158. doi: 10.1002/1521-3773(20011119)40:22<4128::AID-ANIE4128>3.0.CO;2-S
- Niemeyer CM. 2002. The developments of semisynthetic DNA-protein conjugates. Trends Biotechnol. 20:395–401. doi: 10.1016/S0167-7799(02)02022-X
- Nunez ME, Hall DB, Barton JK. 1999. Long-range oxidative damage to DNA: effects of distance and sequence. Chem Bio. 6:85–97. doi: 10.1016/S1074-5521(99)80005-2
- Olofsson J, Larsson S. 2001. Electron hole transport in DNA. J Phys Chem B. 105:10398–10406. doi: 10.1021/jp011052t
- Ortmann F, Hannewald K, Bechsted F. 2009. Charge transport in guanine-based materials. J Phys Chem B. 113:7367–7371. doi: 10.1021/jp901029t
- Osakada Y, Kawai K, Fujitsuka M, Majima T. 2006. Charge transfer through DNA nanoscaled assembly programmable with DNA building blocks. PNAS. 103:18072–18076. doi: 10.1073/pnas.0607148103
- Peyrard M. 2004. Nonlinear dynamics and statistical physics of DNA. Nonlinearity. 17:R1–R40. doi: 10.1088/0951-7715/17/2/R01
- Porath D, Cuniberti G, Felice RD. 2004. Charge transport in DNA based devices. Curr Chem. 237:183–228. doi: 10.1007/b94477
- Rakitin A, Aich P, Papadopoulos C, Kobzar Yu, Vedeneev AS, Lee JS, Xu JM. 2001. Metallic conduction through engineered DNA: DNA nanoelectronic building blocks. Phys Rev Lett. 86:3670–3673. doi: 10.1103/PhysRevLett.86.3670
- Rawtani D, Agrawal YK. 2012. Study the interaction of DNA with Halloysite nanotube-gold nanoparticle based composite. J BioNanoSci. 6:95–98. doi: 10.1166/jbns.2012.1080
- Rawtani D, Agrawal YK. 2013. A Study of the bBehavior of HNT with DNA Intercalator Acridine Orange. BioNanoSci. 3:52–57. doi: 10.1007/s12668-012-0066-1
- Rawtani D, Agrawal YK. 2014. Interaction of Methotrexate with DNA Analyzed by using gold nanoparticles as a probe. Instrum Sci Technol. 42:308–319. doi: 10.1080/10739149.2013.869757
- Roche S, Bicout D, Macia E, Kats E. 2003. Long-range correlation in DNA: scaling properties and charge transfer efficiency. Phys Rev Lett. 91:228101–228104. doi: 10.1103/PhysRevLett.91.228101
- Rothemund PWK. 2006a. Folding DNA to create nanoscale shapes and patterns. Nature. 440:297–302. doi: 10.1038/nature04586
- Rothemund PWK. 2006b. Scaffolded DNA origami: from generalized multi-crossovers to polygonal networks. In: Nanotechnology: science and computation. Natural computing series. Berlin: Springer; p. 3–21. http://link.springer.com/chapter/10.1007%2F3-540-30296-4_1http://link.springer.com/chapter/10.1007%2F3-540-30296-4_1
- Schlag EW, Sheu SY, Yang DY, Selzle HL, Lin SH. 2000a. Theory of charge transport in polypeptides. J Phys Chem B. 104:7790–7794. doi: 10.1021/jp000606+
- Schlag EW, Yang DY, Sheu SY, Selzle HL, Lin SH, Rentzepis PM. 2000b. Dynamical principles in biological processes: a model of charge migration in proteins and DNA. PNAS. 97:9849–9854. doi: 10.1073/pnas.140196597
- Schleif R. 1988. DNA binding by proteins. Science. 241:1182–1187. doi: 10.1126/science.2842864
- Schuster GB. 2004. Long-range charge transfer in DNA. Berlin, Heidelberg: Springer.
- Schwögler A, Carell T. 2000. Toward catalytically active oligonucleotides: synthesis of a Flavin Nucleotide and its incorporation into DNA. Org Lett. 2:1415–1418. doi: 10.1021/ol005739s
- Seeman NC. 1998. Nucleic acid nanostructures and topology. Angew Chemie Int Ed. 37:3220–3238. doi: 10.1002/(SICI)1521-3773(19981217)37:23<3220::AID-ANIE3220>3.0.CO;2-C
- Seeman NC. 2003. Biochemistry and structural DNA nanotechnology: an evolving symbiotic relationship. Biochemistry. 42:7259–7269. doi: 10.1021/bi030079v
- Sessler JL, Jayawickramarajah J. 2005. Functionalized base-pairs: versatile scaffolds for self-assembly. Chem Commun. 21:1939–1949. doi: 10.1039/b418526a
- Storhoff JJ, Mirkin CA. 1999. Programmed materials synthesis with DNA. Chem Rev. 99:1849–1862. doi: 10.1021/cr970071p
- Takada T, Kawai K, Cai XC, Sugimoto A, Fujitsuka M, Majima T. 2004. Charge separation in DNA via consecutive adenine hopping. J Am Chem Soc. 126:1125–1129. doi: 10.1021/ja035730w
- Takezawa Y, Shionoya M. 2012. Metal-mediated DNA base pairing: alternatives to hydrogen-bonded Watson–Crick base pairs. Acc Chem Res. 45:2066–2076. doi: 10.1021/ar200313h
- Travers AA. 1989. DNA conformation and protein binding. Annu Rev Biochem. 58:427–452. doi: 10.1146/annurev.bi.58.070189.002235
- Treadway CR, Hill MG, Barton JK. 2002. Charge transport through a molecular stack: Double helical DNA. Chem Phys. 281:409–428. doi: 10.1016/S0301-0104(02)00447-0
- Troisi A, Orlandi G. 2001. The hole transfer in DNA: calculation of electron coupling between close bases. Chem Phys Lett. 344:509–518. doi: 10.1016/S0009-2614(01)00792-8
- Tucker WO, Shum KT, Tanner JA. 2012. G-quadruplex DNA Aptamers and their ligands: structure, function and application. Curr Pharm Des. 18:2014–2026. doi: 10.2174/138161212799958477
- Vázquez de Parga AL, Hernán OS, Miranda R, Levy Yeyati A, Mingo N, Martín-Rodero A, Flores F. 1998. Electron resonances in sharp tips and their role in tunneling spectroscopy. Phys Rev Lett. 80:357–360. doi: 10.1103/PhysRevLett.80.357
- Wang H, Lewis JP, Sankey OF. 2004. Band-gap tunneling states in DNA. Phys Rev Lett. 93:016401. doi: 10.1103/PhysRevLett.93.016401
- Wang X, Liang S. 2015. Topological effects of charge transfer in telomere G-quadruplex mechanism on telomerase activation and inhibition. Int J Mod Phys B. 9:54.
- Wengel J. 2004. Nucleic acid nanotechnology–towards Angstrom-scale engineering. Org Biomol Chem. 2:277–280. doi: 10.1039/B313986G
- Woiczikowski PB, Kubar T, Gutiérrez R, Cuniberti G, Elstner M. 2010. Standard stability vs conformational sampling in biomolecular systems: why is the charge transfer efficiency in G4-DNA better than in double stranded DNA? J Chem Phys. 133:035103. doi: 10.1063/1.3460132
- Xu MS, Tsukamoto S, Ishida S, Kitamura M, Arakawa Y, Endres RG, Shimoda M. 2005. Conductance of single thiolated poly(GC)-poly(GC) DNA molecules. Appl Phys Lett. 87:083902. doi: 10.1063/1.2032605
- Yaku H, Fujimoto T, Murashima T, Miyoshi D, Sugimoto N. 2012. Phthalocyanines: a new class of G-quadruplex-ligands with many potential applications. Chem. Commun. 48:6203–6216. doi: 10.1039/c2cc31037f
- Yavin E, Boal AK; Stemp EDA, Boon EM, Livingston AL, O’Shea VL, David SS, Barton JK. 2005. Protein-DNA charge transport: redox activation of a DNA repair protein by guanine radical. Proc Natl Acad Sci USA. 102:3546–3551. doi: 10.1073/pnas.0409410102
- You ZQ, Shao Y, Hsu C-P. 2004. Calculating electron transfer couplings by the Spin-Flip approach: energy splitting and dynamical correlation effects. Chem Phys Lett. 390:116–123. doi: 10.1016/j.cplett.2004.03.142
- Yu Z, Song X. 2001. Variable range hopping and electrical conductivity along the DNA double helix. Phy Rev Lett. 86:6018–6021. doi: 10.1103/PhysRevLett.86.6018
- Zhao Y, Kan Z, Zeng Z, Hao Y, Chen H, Tan Z. 2004. Determining the folding and unfolding rate constants of nucleic acids by biosensor. Application to telomere G-Quadruplex. J Am Chem Soc. 126:13255–13264. doi: 10.1021/ja048398c